DOI:
10.1039/C7RA04228K
(Paper)
RSC Adv., 2017,
7, 32518-32527
Molecular interactions between DOPA and surfaces with different functional groups: a chemical force microscopy study†
Received
14th April 2017
, Accepted 20th June 2017
First published on 26th June 2017
Abstract
The adhesion of mussel foot proteins (Mfps) to a variety of surfaces has been widely investigated, but the mechanisms behind the mussel adhesion to surfaces with different properties are far from being understood. Most of Mfps contain a significant amount of 3,4-dihydroxyphenylalanine (DOPA) which is considered to be responsible for the strong wet adhesion. In the present work, self-assembled monolayers (SAMs) were prepared as a series of model surfaces with variable functional groups. DOPA-surface interactions were investigated using chemical force microscopy (CFM) for the first time, in which an atomic force microscope (AFM) tip was chemically modified with DOPA terminated groups. The ability of DOPA to adhere to different surfaces with variable wettability was compared, showing that DOPA behaves with the strongest and weakest adhesion to C6H5- and OH-terminated surfaces, respectively. The interaction strength of DOPA at different surfaces does not always increase with the increase of surface wettability, because the hydrophobic interaction does not play a decisive role in DOPA adhering to surfaces. By the use of classical and extended Derjaguin–Landau–Verwey–Overbeek (DLVO) theories, the contribution of non-DLVO forces was isolated. We found out DOPA can adhere to each surface functional group, since DOPA residues containing o-hydroxy or aromatic rings alone can control the adhesion process, and the aromatic ring is oriented perpendicularly or parallel to the surface. This study served as a basis for understanding the relationship between DOPA adhesion mechanisms and different wet surfaces, representing important concepts for the design of bioadhesive materials and anti-adhesion surfaces.
Introduction
Marine mussels are a well-known biological family due to their remarkable ability to stick to wet surfaces and achieve long-lasting adhesion.1–7 On the one hand, the undesired colonization has serious impacts, in particular in promoting ship surface corrosion, increasing fuel consumption, and hampering the accuracy and reliability of underwater sensors.8,9 On the other hand, the desired adhesion can be developed as excellent biomimetic adhesives of soft tissue, which promotes tissue regeneration and minimizes surgery time by replacing common surgical procedures.10,11 The reason why mussels are good at attaching to virtually any material underwater, whether natural or synthetic, is that mussels produce a bundle of threads tipped with adhesive pads, known collectively as byssus, composing of different types of mussel foot proteins (Mfps).12,13 3,4-Dihydroxyphenylalanine (DOPA) is a unique amino acid widely found in Mfps: mfp-3 and mfp-5 have been found to have a high content, 20–30 mol%, of DOPA that plays a key role in mussel adhesion.6,14–16 However, the contribution of DOPA in the adhesion process of mussels at surfaces has still been a challenging problem.17–21 Till now, there has been only a little knowledge about the interactions between DOPA and inorganic surfaces or organic surfaces (including polytetrafluoroethylene, polystyrene, and high-density polyethylene) underwater.14,22 While in the natural world, pristine surfaces are always covered by various organic films in a dynamic and patchy process,23,24 and therefore, it is necessary to understand DOPA binding mechanisms to such fouled films due to the great importance in marine biofouling and the biocompatibility of biomaterials. Besides, there is currently a lack of knowledge about how material factors, such as surface chemistry and wettability, can control protein adhesion/morphology.25
With this in mind, we focused on how changing intricate surface chemistry can influence DOPA adhesion, and fabricated a series of model surfaces (self-assembled monolayers (SAMs) which provide an easy and controlled way to create surfaces with different functional groups), and investigated the interactions of DOPA-surface as a function of surface chemistry. Although surface force apparatus (SFA) has been used to measure the macroscopic dissociation of two surfaces adhered by mussel proteins, yet the method did not reveal the adhesive interaction of DOPA–SAMs surface.19,26–32 In order to gain further insight into the molecular interactions between DOPA molecules and the series of SAM surfaces, chemical force microscopy (CFM) was introduced to the present study, in which the contribution of DOPA in mussel adhesion was successfully isolated. With this approach, we were able to quantitatively measure the interactions between DOPA and seven different wettability surfaces systematically, and find the relationship between DOPA adhesion and surface wettability, as well as reveal which surfaces have the potential to be applied to the anti-adherent material and which have the potential to be used in making adhesive materials. By the use of classical and extended Derjaguin–Landau–Verwey–Overbeek (DLVO) theories, the contribution of non-DLVO forces was evaluated. DOPA adhered to various functional groups through different interactions, from which the most important binding types were concluded. This study provides novel strategies for the design of antifouling and adhesive surfaces underwater.
Experimental section
Preparation of self-assembled monolayers (SAMs)
Silicon wafers were cut into square strips and soaked in piranha solution (H2SO4/H2O2 = 3
:
1 v
:
v) for 30 min at 85 °C, and then thoroughly rinsed with Millipore Milli-Q grade water (18.2 MΩ cm). Gold substrates were prepared by first evaporating 5 nm of Cr adhesion layer on cleaned silicon wafers, and then 50 nm of Au under a high vacuum through an electron beam evaporator (Peva-600E, Campro, Taiwan). Prior to SAM formation on these substrates, they were cleaned by dipping them for 1 min in piranha solution, and then rinsed copiously with Millipore Milli-Q grade water. SAMs-functionalized surfaces were prepared as per the established protocols described previously.33–35 The freshly clean substrates were immersed in ethanolic solutions of 11-mercapto-1-undecanol (SH–(CH2)11OH, Aldrich), 11-mercaptoundecanoic acid (SH–(CH2)10COOH, J&K Scientific Ltd), 11-amino-1-undecanethiol, hydrochloride (SH–(CH2)11NH2 HCl, Aldrich), 1H,1H,2H,2H-perfluorodecanethiol (SH–(CH2)2(CF2)7CF3, J&K Scientific Ltd), octadecanethiol (SH–(CH2)17CH3, Alfa Aesar), and 10-phenyldecylmercaptan (SH–(CH2)10C6H5, Aldrich) at the concentration of 1 mM for 24 h, respectively. The substrates were thoroughly rinsed by ethanol to remove physisorbed thiol from them and dried with pure nitrogen stream.
Surface characterization of SAMs
X-ray photoelectron spectroscopy (XPS) was performed on Thermo Scientific ESCALab 250Xi using 200 W monochromated Al Kα radiation. The 500 μm X-ray spot was used for XPS analysis. The base pressure in the analysis chamber was about 3 × 10−10 mbar. Typically the hydrocarbon C 1s line at 284.8 eV from adventitious carbon is used for energy referencing.
The contact angle (CA) was measured through a Kruss DSA CA goniometer (Germany) equipped with a dispensing needle. The sessile droplet with a volume of 5 μL was formed by fixing the needle and approaching the substrate in parallel with the needle direction at a slow feed rate. All the measurements were performed in air at ambient temperature.
Surface potentials of the series of surfaces (size in 20 mm long, 10 mm wide, and 1 mm thick) were measured on a surpass electrokinetic analyzer (Anton Parr, Austria). Determination of the potentials was based on the measurement of streaming potential and current. In the streaming potential measurements, a water buffered with 25 mM Tris HCl solution at pH 5.5 was pumped to flow along the surface of the sample and the potential was measured based on the following relationship:36
|
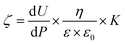 | (1) |
where
ζ is zeta potential; d
U/d
P denotes the slope of streaming potential
versus pressure;
η,
ε0,
ε, and
K represent electrolyte viscosity, vacuum permittivity, dielectric constant of the buffer, and its conductivity, respectively. The streaming potential or alternatively the streaming current was detected by the electrodes placed at both sides of the sample, as shown in Table S1.
†
Surface topography of SAM-functionalized surfaces was measured via AFM in a peakforce tapping mode (Dimension Fast scan, Bruker Corp.). AFM measurements were conducted using a probe made of silicon nitride (Fast Scan-B, Bruker Corp.). The topography images were performed on a scan area of 1 μm × 1 μm at a speed of 3.91 Hz.
AFM tip modification and characterization
AFM silicon nitride tips (HLCT, Bruker Corp.) were cleaned in an O2 plasma (Evactron 25, XEI Scientific, Inc, USA) for 15 min and then subsequently transferred to a piranha solution for 30 min (reaction I in Fig. 1), in order to remove impurities and generate a hydroxyl layer on the tip surface. After gently rinsing with Mili-Q water, the tip was transferred into 0.5 mM silane-PEG-NH2 (MW 3400, Nanocs) in toluene solution for 6 h, resulting in an aminosilane-functionalized tip (reaction II). The tip was thoroughly rinsed with large amounts of toluene solution to remove unreacted molecules. Then the cantilever was stored in an oven at 110 °C for 10 min to stabilize the silane conjugation. Next, the tip was immersed in a N,N-dimethylformamide (DMF) solution containing N-methylmorpholine, 2-(7-aza-1H-benzotriazole-1-yl)-1,1,3,3-tetramethyluronium hexafluorophosphate (HATU) and N-Boc–DOPA for 3 h, followed by coupling of N-Boc–DOPA to the liberated amine in solution, as shown in reaction III. After the modification, N-Boc–DOPA was end-tethered to PEG, and Boc protected amine groups remaining in place to avoid electrostatic interactions.14
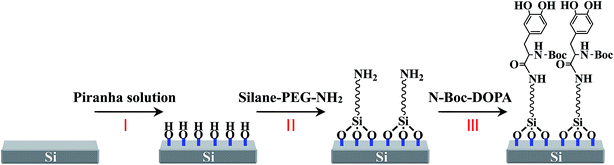 |
| Fig. 1 Schematic of AFM tip preparation. | |
In order to confirm that the chemical modification of the AFM tip was successful, we functionalized a silicon substrate with Boc–DOPA using exactly the same procedure that we used for the tip. We characterized the resulting surface at each step of the procedure through XPS and CA methods (Fig. S1†). C 1s peaks at around 284.8 eV and 286.5 eV were observed, which were assigned to C–C and C–O, respectively. The peak of N 1s at ∼400 eV was observed. The change of the test element (C and N) content was consistent with the change of the surface chemical composition along with the modification process. The contact angle also increased with the modification from 17° to 28°, further illustrating the success of the modification process. In the absence of DOPA, the PEG itself interacted weakly with surfaces as compared with the DOPA-functionalized AFM tips, as shown in Fig. S2.† In the test of adhesion interactions between DOPA and each substrate, a small amount of free amino groups had little effect on the interaction results, which can be neglected even if there were some unsuccessful grafting of DOPA.
Force-measuring technique
The fluid chamber was filled in with 1.5 mL of buffer containing 25 mM Tris HCl at pH 5.5. Atomic force microscopic (AFM, HLCT, Bruker Corp.) experiments were conducted after allowing the system to equilibrate for 30 min. AFM silicon nitride cantilevers with silicon nitride tips (MLCT, Bruker Corp.) were used in all experiments. The spring constants of the tips were in the range of 0.05–0.07 N m−1 calibrated by thermal fluctuation method. In order to ensure that the DOPA used for testing was not oxidized, the DOPA functionalized AFM tip was immersed in 1 mM of ascorbic acid solution for 30 min before each AFM experiment.22,37 In a typical experiment, a cantilever was brought to the surface at a constant speed of 1000 nm s−1 and held on the surface at constant forces of 1–2 nN to allow a sufficient contact of DOPA-modified tip and functional surface. Then the cantilever was moved back at the same speed to break DOPA-surface interactions. We used the same DOPA-terminated tip and made all the AFM measurements in the same conditions (such as spring constant, constant speed, et al.) to minimize the influence of DOPA modification density on DOPA binding strength. All AFM force measurements were carried out at room temperature.
Theory and calculation
DLVO model
Total DLVO interaction force (Ftotal) between two interacting surfaces was calculated as the sum of Lifshitz–van der Waals (Fvan der Waals) and electrostatic interactions (Felectrostatic), as shown in eqn (2):38 |
Ftotal = Fvan der Waals + Felectrostatic
| (2) |
The expression for the van der Waals force between AFM tip and substrate, based on Hamaker's approach and Derjaguin's approximation used in this study, is given by eqn (3):39
|
 | (3) |
where
a is the radius of AFM tip (∼20 nm);
h is the surface-to-surface distance between substrate and AFM tip;
A is the effective Hamaker constant, which is the key point in calculating van der Waals forces, as expressed by
eqn (4):
40,41 |
 | (4) |
where
ε1,
ε2, and
ε3 are the static dielectric constants; media 1 and 2 represent AFM tip and substrate, respectively; media 3 stands for water buffered with 25 mM Tris HCl (pH 5.5);
ε1(iv),
ε2(iv), and
ε3(iv) are the electronic absorption terms;
k is the Boltzmann constant, 1.381 × 10
−23 J K
−1;
T is the Kelvin temperature, 298.15 K;
h is the plank constant, 6.626 × 10
−34 J s.
Assuming constant surface potential on the substrates, the well-known Hogg, Healy, and Fuerstenau (HHF) expression for the electrostatic force is used in fitting the data, as shown in eqn (5):42,43
|
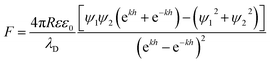 | (5) |
where
ε0 (8.854 × 10
−12 C
2 J
−1 m
−1) is the permittivity of vacuum, and
ε (80) denotes the relative permittivity of the solution;
ψ1 and
ψ2 correspond to the surface potentials of substrate and probe surface, respectively;
h is the surface-to-surface distance between substrate and AFM tip; surface potentials are approximated by
ζ potentials (mV) of functionalized surfaces (Table S1
†);
λD denotes the Debye length as described by
eqn (6):
44,45 |
 | (6) |
where
e is the electron charge (1.602 × 10
−19 C) and
c corresponds to the concentration.
Extended DLVO model
In the present paper, DLVO theory was extended to provide a more complete interpretation of force-distance experimental data, as expressed in eqn (7):38,46,47 |
Ftotal = Fvan der Waals + Felectrostatic + Fstructrual
| (7) |
where Fstructrual represents non-DLVO forces, including hydrogen bonding, metal coordination, π–cation interaction, et al. |
 | (8) |
where C and λ describe coefficient and decay length, respectively; b is a real constant; h denotes separation of tip-substrate. When the fitting profiles were consistent with the experimental profiles, the fitting parameters of EDLVO model were obtained as shown in Table S2.†
Results and discussion
Surface characterization
On the series of SAM-terminated surfaces, contact angle of water droplet on such surfaces increases from ∼25° to ∼109°, as shown in Fig. 2a–g, implying that the model surfaces vary gradually in water wettability. From Fig. 2h–n (except bare gold surface in Fig. 2k), the high-resolution XPS 2p spectrum of SAMs exhibits a characteristic doublet with red curves peaks at around 162.1 eV (2p3/2) and 163.3 eV (2p1/2) corresponding to thiolates (RS-) bound to gold. Additionally, a minor doublet component with blue curves peaks at around 163.8 eV and 164.4 eV appears, corresponding to unbound thiol residues but in a relatively low content.48,49 A peak at around 286.7 eV, 289.1 eV, 286.4 eV is observed and attributed to C–O, COOH, C–N groups, respectively (Fig. 2h′–j′); the peak at ∼284.8 eV is assigned to C–C group in all the cases. For an aromatic SAM (Fig. 2l′), a broad peak at 291.5 eV is observed, locating at ca. 7 eV higher than the C–C peak at ∼284.8 eV, which can be assigned to shakeup satellite. Similar satellite peaks in C 1s XPS were observed in aromatic monolayers on metallic substrates previously.50,51 Fig. 2m′ shows C 1s XPS spectra, mainly composed of –CH2– binding of SAM–CH3. Among the C 1s peaks for the SAM–CF3, the peaks at around 290.9 eV and 293.3 eV are observed as shown in Fig. 2n′, which are assigned to –CF2– and –CF3 peaks, respectively. These results demonstrate the successful film growth of SAM–OH, SAM–COOH, SAM–NH2, SAM–C6H5, SAM–CH3, and SAM–CF3 from gold substrates.
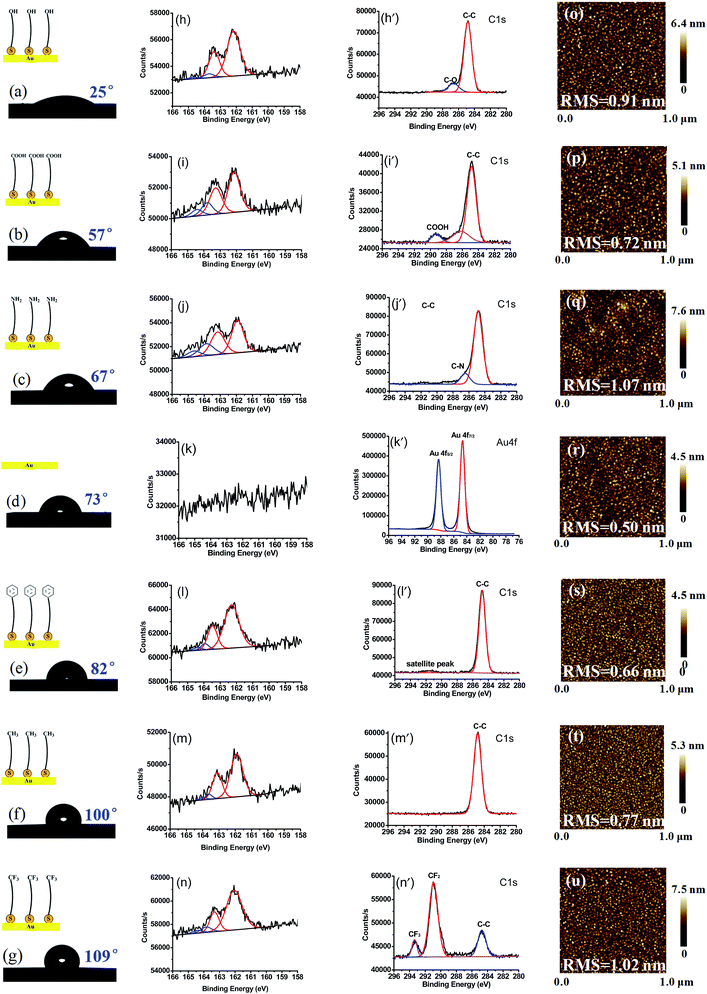 |
| Fig. 2 CA (a–g), XPS spectra (h–n and h′–n′), and AFM topography (o–u) of bare gold surface and different substrates modified by SAM–OH, SAM–COOH, SAM–NH2, SAM–C6H5, SAM–CF3, and SAM–CH3, respectively. | |
From AFM topographies (Fig. 2o–u), almost all these surfaces exhibit a flat and homogenous conformation with a small RMS value of ∼1 nm. The monolayer thickness of each surface was in the range of 1.0–1.3 nm using ellipsometry (Table S3†). And the ratio of peak intensities of S 2p3/2 and Au on each surface is in the range of 0.048–0.055 (Table S3†), indicating that the average density of the functional groups modified on each surface is almost the same.52 From all the parameters above, uniform and smooth monomolecular layers are obtained, in which surface roughness and defects probably have little effect on the Dopa binding strength.
Interpretation of direct force measurements
Fig. 3 shows typical force–distance curves for approach and retraction of a DOPA-modified cantilever from different surfaces, where positive force denotes repulsive interaction and negative force represents attractive interaction, respectively. In the case of hydrophilic surfaces (Fig. 3a), a weak attraction of DOPA-surface occurs at short range; at hydrophobic surfaces (Fig. 3b), the force becomes stronger, especially in the presence of C6H5-terminated surface occurring at long range. Fig. 3c shows the tip retracting from hydrophilic surfaces, exhibiting an increase of adhesive force with the enhancement of surface hydrophobicity from SAM–OH to bare gold. When the hydrophobicity continues to increase from SAM–C6H5 to SAM–CF3, the adhesive force decreases from a maximum attractive force at C6H5-terminated surface (Fig. 3d).
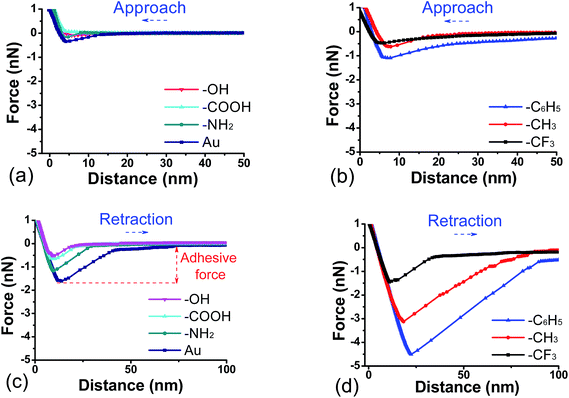 |
| Fig. 3 Approach (a and b) and retraction (c and d) force curves as a function of separation between DOPA-modified AFM tip and functional surfaces in buffer solution. | |
Further investigation on the surface forces are shown as histograms of adhesive forces in the presence of different surfaces (Fig. 4). When the DOPA-modified AFM tip separates from OH-, COOH-, and NH2-terminated surfaces, the distribution of adhesive force is narrow and the peak value is around 1 nN. With the increase of surface hydrophobicity, adhesive force reaches the maximum numerical range of 2.2–6.2 nN in the presence of C6H5-terminated surface. The adhesive force of DOPA-surface becomes weaker when the hydrophobicity continues to increase, such as CH3- and CF3-terminated surfaces. Previous studies showed that hydrophobic forces dominate the adhesion between hydrophobic phenylalanine residue and inorganic substrate,53 while herein we found that the interaction strength of DOPA does not always increase with the increase of contact angle (Fig. 4h). It indicates that the hydrophobic interaction does not play a decisive role in the DOPA adhering to different wet surfaces, instead, other forms of binding are proposed to do.
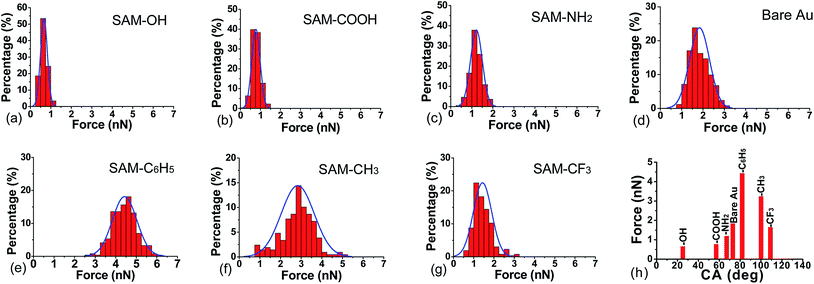 |
| Fig. 4 Histograms of adhesion forces in the presence of (a) SAM–OH, (b) SAM–COOH, (c) SAM–NH2, (d) bare gold, (e) SAM–C6H5, (f) SAM–CF3, (g) SAM–CH3 surfaces, and (h) comparison of all the average adhesive forces. Blue lines correspond to a Gaussian fit. | |
DLVO and EDLVO theories in modeling the approach force profiles
In order to illustrate the contribution of DLVO interactions, the resultant forces (including van der Waals and electrostatic forces) are calculated through eqn (2)–(6) (see green lines in Fig. 5). The calculated profiles are not fitted with the experimental data, primarily attributed to the fact that the continuum theory of attractive van der Waals force and repulsive double-layer force fails to describe the interactions between DOPA-modified AFM tips and functional surfaces as a function of separation from each other. So, an additional force, denoted as structural force, should be taken into account, which plays an important role in such cases. Via the EDLVO model (eqn (7) and (8)), the peak size of the approach curve agrees with the structural force, indicating that other kinds of interactions come into play. On the OH-, COOH-, and NH2-terminated surfaces (Fig. 5a–c), in addition to DLVO forces, a weak attractive force appears at short-range, attributed to hydrogen bond interaction between hydroxyl groups of DOPA (hydrogen donors) and oxygen or nitrogen atoms (hydrogen acceptors) of the surface (Fig. 6a). The electronegativity of nitrogen is weaker than oxygen, so it is considered that the binding strength of N⋯H–O hydrogen bonds (in the case of NH2-terminated surface) is also weaker than that of O⋯H–O hydrogen bonds (in the case of OH- and COOH-terminated surfaces). But, it is noticed that the attractive force reaches the strongest one in the presence of NH2-terminated surface among the hydrophilic surfaces, because cation–π interaction may also be present between the charged amine groups of surface and the catechol groups of DOPA in addition to hydrogen bonds, which is considered to make a strong contribution to the attractive force. The aromatic ring and o-hydroxy groups in the residues of DOPA can form hydrophobic and coordination interaction with the gold surface (Fig. 5d), respectively. Compared with hydrophobic interaction, coordination plays a decisive role in the adhesion of DOPA to gold surface.54
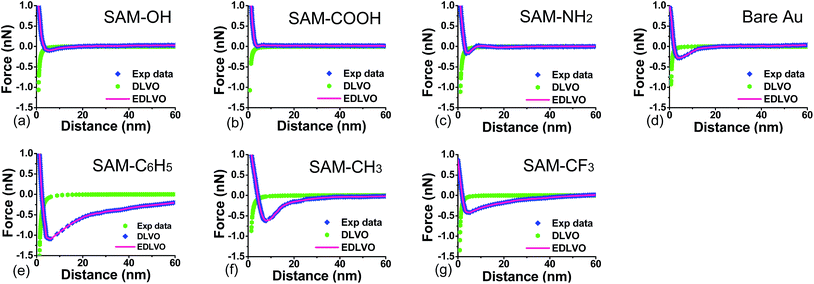 |
| Fig. 5 Force profiles and their predictions through DLVO and EDLVO models between DOPA-modified AFM tips and functional surfaces in buffer solution. | |
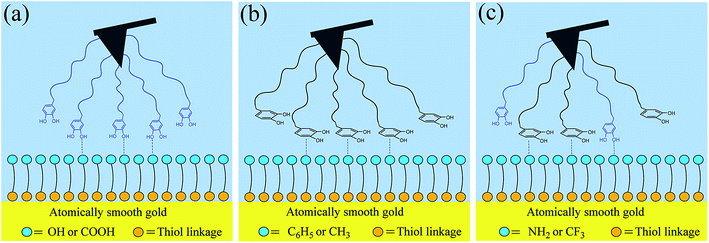 |
| Fig. 6 Schematic representation of interactions of DOPA-surface. | |
When the surface wettability tends to be more hydrophobic, such as C6H5-terminated surface, the attractive force reaches a maximum value, probably due to quadrupole–quadrupole interaction between aromatic groups of DOPA and the functional surface,55–57 or both quadrupole–quadrupole and hydrophobic interactions (Fig. 6b). When the surface contact angle continues to increase, the attractive force in the case of CH3-terminated surface is dominated by the hydrophobic interaction between the aromatic ring of DOPA and the alkyl surface. By contrast, the attractive force continues to decrease on the CF3-terminated surface, because part of the DOPA molecules of the probe participate into hydrophobic interactions and part of them participate into hydrogen bonds,58 in which case hydrogen bond interaction is considered to make a weak contribution to the attractive force.
Via the extended DLVO (EDLVO) model, the fitting profiles are obtained (see pink lines in Fig. 5), with all the fitting parameters listed in the above Table S2.† It can be concluded that the new model successfully predicts the relationship between force and distance observed in the experimental profiles, indicating that other kinds of interactions (such as hydrophobic interaction et al.) come into play. On the OH-, COOH-, and NH2-terminated surface (Fig. 5a–c), in addition to electrostatic and van der Waals forces, a weak attractive force appears at short-range, attributed to H-bond interaction between hydroxyl groups of DOPA (hydrogen donors) and oxygen or nitrogen atoms (hydrogen acceptors) on the surface. For gold surface, the attraction is stronger, because of the hydrophobic interaction of DOPA–gold, which is similar to DOPA–hydrophobic surface interaction. When the surface wettability tends to be more hydrophobic, such as C6H5-terminated surface, the attractive force reaches a maximum value, due to quadrupole–quadrupole interaction between aromatic groups of DOPA and the functional surface, as well as hydrophobic interaction between aromatic group of DOPA and hydrophobic chain of the surface. It indicates that two surfaces of DOPA-modified AFM tip and functional surface contact with each other closely, which is the main reason why they tend to adhere each other during separation. When the surface contact angle continues to increase, a likely reason for the decrease of attractive force in the case of CH3-terminated surface is the single hydrophobic interaction between the aromatic ring of DOPA and the alkyl surface. By contrast, the attractive force reduces further as shown in Fig. 5g, because of both contributions of H-bond and hydrophobic interactions, in which H–bond interaction originates from hydroxyl groups of DOPA bound to fluorine (highly electronegative atoms) of perfluorinated surface.
Herein, we prepared a series of SAM-modified gold surfaces with a variation in water wettability and surface chemistry, and investigated the interactions of DOPA with model surfaces through chemical force microscopy. It was found that surface wettability is not the single key parameter to prevent or enhance DOPA-surface adhesion, but the surface chemistry plays an important role in it. Moreover, it was found out DOPA has a strong ability to adhere to various surfaces, because DOPA contains catechol groups owning both of aromatic ring and o-hydroxy structure which can determine the adhesion behavior between DOPA and surface. For o-hydroxy controlled adhesion surfaces, such as OH- and COOH-terminated surfaces, the aromatic ring of DOPA is proposed to have a perpendicular orientation with the surface as shown in Fig. 6a; for aromatic ring controlled adhesion surfaces, such as CH3- and C6H5-terminated surfaces, the aromatic ring is suggested to have a parallel orientation with the surface as shown in Fig. 6b. For the SAM–C6H5 surface, it does not exhibit the strongest hydrophobic property while exhibits the strongest adhesion of DOPA-surface among all the modified surfaces, because there may be a superposition resulting from two kinds of binding forces of quadrupole–quadrupole and hydrophobic interactions. While in the case of SAM–NH2 or SAM–CF3 surfaces, it is proposed to be a mixed superposition configuration, because DOPA residues can interact with surface in perpendicular or parallel, respectively, as shown in Fig. 6c. Among the hydrophilic surfaces, the attractive force reaches the strongest one in the case of NH2-terminated surface, probably because catechol groups can form hydrogen bonding or cation–π interaction with the surface. Among the hydrophopbic surfaces, the attractive force reaches the weakest one in the case of CF3-terminated surface, because catechol groups can form hydrophobic interaction or hydrogen bonding with the surface, and each of the catechol groups cannot take part in the two interactions at the same time.
Conclusions
We developed a novel approach to investigate the interactions between DOPA and specific functional group surfaces with variable wettability. When the DOPA-terminated tip retracted from each surface, the adhesion increases with the enhancement of surface hydrophobicity from SAM–OH to SAM–C6H5; when the surface hydrophobicity continues to increase, the adhesion gradually decreases, indicating that the hydrophobic interaction does not play a decisive role, instead, other forms of binding play a key role. We found that DOPA can adhere to different functional surfaces, because aromatic ring or o-hydroxy structure of catechol group can separately dominate the adhesion of DOPA-surface. It was proposed two kinds of configurations of the DOPA residue on the surface: one is the plane of the aromatic ring being perpendicular on the surface and binding to it via o-hydroxy of catechol, such as OH- and COOH-terminated surfaces, and the other is the plane of the aromatic ring being parallel to the surface and binding to it via aromatic ring of catechol, such as C6H5- and CH3-terminated surfaces. Furthermore, there may be two forms of binding in a single configuration, such as SAM–C6H5 surface, due to a superposition of two kinds of binding forces (quadrupole–quadrupole and hydrophobic interactions). For NH2- and CF3-terminated surfaces, two kinds of configurations of the DOPA residue were proposed on the surface, separately. DOPA exhibits a stronger adhesion to the NH2-terminated surface than that to other hydrophilic surfaces, due to cation–π interaction which is considered to make a strong contribution to the attractive force. While, DOPA exhibits a weakest adhesion to the CF3-terminated surface than that to other hydrophobic surfaces, due to hydrogen bond interaction which is considered to make a weak contribution to the attractive force. Compared with surface wettability, surface chemical composition determines different types of DOPA-surface interactions, being a key factor in DOPA adhesion process. This research can be applied to the design and fabrication of adhesion or anti-adhesion materials.
Conflict of interest
The authors declare no competing financial interest.
Acknowledgements
This work was funded by the projects of PetroChina Exploration and Development Research Institute (2015-40222-000006 and RIPED-2017-JS-88), the Important National Science and Technology Specific Project of China (2017ZX05013003004 and 2016ZX05025003009), and the National Natural Science Foundation of China (21603240).
References
- H. G. Silverman and F. F. Roberto, Understanding marine mussel adhesion, Mar. Biotechnol., 2007, 9(6), 661–681 CrossRef CAS PubMed
. - H. J. Cha, D. S. Hwang and S. Lim, Development of bioadhesives from marine mussels, Biotechnol. J., 2008, 3(5), 631–638 CrossRef CAS PubMed
. - D. J. Crisp, G. Walker, G. A. Young and A. B. Yule, Adhesion and substrate choice in mussels and barnacles, J. Colloid Interface Sci., 1985, 104(104), 40–50 CrossRef
. - J. H. Waite, Adhesion a la moule, Integr. Comp. Biol., 2002, 42(6), 1172–1180 CrossRef CAS PubMed
. - M. A. Even, J. Wang and Z. Chen, Structural information of mussel adhesive protein mefp-3 acquired at various polymer/mefp-3 solution interfaces, Langmuir, 2008, 24(11), 5795–5801 CrossRef CAS PubMed
. - J. H. Waite and M. L. Tanzer, Polyphenolic substance of mytilus edulis: novel adhesive containing L-Dopa and hydroxyproline, Science, 1981, 212(4498), 1038–1040 CAS
. - J. H. Waite, N. H. Andersen, S. Jewhurst and C. J. Sun, Mussel adhesion: finding the tricks worth mimicking, J. Adhes., 2005, 81(3–4), 297–317 CrossRef CAS
. - K. G. Neoh and E. T. Kang, Combating bacterial colonization on metals via polymer coatings: relevance to marine and medical applications, ACS Appl. Mater. Interfaces, 2011, 3(8), 2808–2819 CAS
. - J. Ren, P. P. Han, H. L. Wei and L. Y. Jia, Fouling-resistant behavior of silver nanoparticle-modified surfaces against the bioadhesion of microalgae, ACS Appl. Mater. Interfaces, 2014, 6(6), 3829–3838 CAS
. - M. Donkerwolcke, F. Burny and D. Muster, Tissues and bone adhesives—historical aspects, Biomaterials, 1998, 19(16), 1461–1466 CrossRef CAS PubMed
. - B. J. Kim, D. X. Oh, S. Kim, J. H. Seo, D. S. Hwang, A. Masic, D. K. Han and H. J. Cha, Mussel-mimetic protein-based adhesive hydrogel, Biomacromolecules, 2014, 15(5), 1579–1585 CrossRef CAS PubMed
. - R. Y. Floriolli, L. J. von and J. H. Waite, Marine surfaces and the expression of specific byssal adhesive protein variants in mytilus, Mar. Biotechnol., 2000, 2(4), 352–363 CAS
. - J. H. Waite and X. X. Qin, Polyphenolic polyphosphoprotein from the adhesive pads of mytilus edulis, Biochemistry, 2001, 40(9), 2887–2893 CrossRef CAS PubMed
. - H. Lee, N. F. Scherer and P. B. Messersmith, Single-molecule mechanics of mussel adhesion, Proc. Natl. Acad. Sci. U. S. A., 2006, 103(35), 12999–13003 CrossRef CAS PubMed
. - V. V. Papov, T. V. Diamond, K. Biemann and J. H. Waite, Hydroxyarginine-containing polyphenolic proteins in the adhesive plaques of the marine mussel mytilus edulis, J. Biol. Chem., 1995, 270(34), 20183–20192 CrossRef CAS PubMed
. - N. R. Martinez Rodriguez, S. Das, Y. Kaufman, J. N. Israelachvili and J. H. Waite, Interfacial pH during mussel adhesive plaque formation, Biofouling, 2014, 31(2), 221–227 CrossRef PubMed
. - M. Yu and T. J. Deming, Synthetic polypeptide mimics of marine adhesives, Macromolecules, 1998, 31(15), 4739–4745 CrossRef CAS PubMed
. - M. Yu, J. H. Wang and T. J. Deming, Role of L-3,4-dihydroxyphenylalanine in mussel adhesive proteins, J. Am. Chem. Soc., 1999, 121(24), 5825–5826 CrossRef CAS
. - Q. Lin, D. Gourdon, C. Sun, N. Holten-Andersen, T. H. Andersen, J. H. Waite and J. N. Israelachvili, Adhesion mechanisms of the mussel foot proteins mfp-1 and mfp-3, Proc. Natl. Acad. Sci. U. S. A., 2007, 104(10), 3782–3786 CrossRef CAS PubMed
. - J. J. Wang, M. N. Tahir, M. Kappl, W. Tremel, N. Metz, M. Barz, P. Theato and H. J. Butt, Influence of binding-site density in wet bioadhesion, Adv. Mater., 2008, 20(20), 3872–3875 CrossRef CAS
. - Z. A. Levine, M. V. Rapp, W. Wei, R. G. Mullen, C. Wu, G. H. Zerze, J. Mittal, J. H. Waite, J. N. Israelachvili and J. E. Shea, Surface force measurements and simulations of mussel-derived peptide adhesives on wet organic surfaces, Proc. Natl. Acad. Sci. U. S. A., 2016, 113(16), 4332–4337 CrossRef CAS PubMed
. - Y. R. Li, M. Qin, Y. Li, Y. Cao and W. Wang, Single molecule evidence for the adaptive binding of DOPA to different wet surfaces, Langmuir, 2014, 30(15), 4358–4366 CrossRef CAS PubMed
. - D. S. Hwang, M. J. Harrington, Q. Lu, A. Masic, H. Zeng and J. H. Waite, Mussel Foot Protein-1 (Mcfp-1) Interaction with Titania Surfaces, J. Mater. Chem., 2012, 22(31), 15530–15533 RSC
. - J. Yu, W. Wei, E. Danner, J. N. Israelachvili and J. H. Waite, Effects of Interfacial Redox in Mussel Adhesive Protein Films on Mica, Adv. Mater., 2011, 23(20), 2362 CrossRef CAS PubMed
. - J. M. Curran, R. Chen and J. A. Hunt, The Guidance of Human Mesenchymal Stem Cell Differentiation in Vitro by Controlled Modifications to the Cell Substrate, Biomaterials, 2006, 27(27), 4783–4793 CrossRef CAS PubMed
. - J. Yu, Y. Kan, M. Rapp, E. Danner, W. Wei, S. Das, D. R. Miller, Y. Chen, J. H. Waite and J. N. Israelachvili, Adaptive hydrophobic and hydrophilic interactions of mussel foot proteins with organic thin films, Proc. Natl. Acad. Sci. U. S. A., 2013, 110(39), 15680–15685 CrossRef CAS PubMed
. - Q. Lu, E. Danner, J. H. Waite, J. N. Israelachvili, H. Zeng and D. S. Hwang, Adhesion of mussel foot proteins to different substrate surfaces, J. R. Soc., Interface, 2013, 10(79), 20120759 CrossRef PubMed
. - E. W. Danner, Y. J. Kan, M. U. Hammer, J. N. Israelachvili and J. H. Waite, Adhesion of mussel foot protein mefp-5 to mica: an underwater superglue, Biochemistry, 2012, 51(33), 6511–6518 CrossRef CAS PubMed
. - D. S. Hwang, M. J. Harrington, Q. Y. Lu, A. Masic, H. B. Zeng and J. H. Waite, Mussel foot protein-1 (mcfp-1) interaction with titania surfaces, J. Mater. Chem., 2012, 22(31), 15530–15533 RSC
. - J. Yu, W. Wei, M. S. Menyo, A. Masic, J. H. Waite and J. N. Israelachvili, Adhesion of mussel foot protein-3 to TiO2 surfaces: the effect of pH, Biomacromolecules, 2013, 14(4), 1072–1077 CrossRef CAS PubMed
. - H. B. Zeng, D. S. Hwang, J. N. Israelachvili and J. H. Waite, Strong reversible Fe3+-mediated bridging between DOPA-containing protein films in water, Proc. Natl. Acad. Sci. U. S. A., 2010, 107(29), 12850–12853 CrossRef CAS PubMed
. - W. Wei, J. Yu, C. Broomell, J. N. Israelachvili and J. H. Waite, Hydrophobic enhancement of DOPA-mediated adhesion in a mussel foot protein, J. Am. Chem. Soc., 2013, 135(1), 377–383 CrossRef CAS PubMed
. - B. Sivaraman and R. A. Latour, The relationship between platelet adhesion on surfaces and the structure versus the amount of adsorbed fibrinogen, Biomaterials, 2010, 31(31), 832–839 CrossRef CAS PubMed
. - J. M. Curran, R. Chen and J. A. Hunt, The guidance of human mesenchymal stem cell differentiation in vitro by controlled modifications to the cell substrate, Biomaterials, 2006, 27(27), 4783–4793 CrossRef CAS PubMed
. - B. Sivaraman, K. P. Fears and R. A. Latour, Investigation of the effects of surface chemistry and solution concentration on the conformation of adsorbed proteins using an improved circular dichroism method, Langmuir, 2009, 25(5), 3050–3056 CrossRef CAS PubMed
. - H. L. Cao, X. Y. Liu, F. H. Meng and P. K. Chu, Biological actions of silver nanoparticles embedded in titanium controlled by micro-galvanic effects, Biomaterials, 2011, 32(3), 693–705 CrossRef CAS PubMed
. - J. Yu, E. Danner, J. N. Israelachvili and J. H. Waite, Effects of interfacial redox in mussel adhesive protein films on mica, Adv. Mater., 2014, 23(20), 2362–2366 CrossRef PubMed
. - J. Israelachvili, Interfacial forces, J. Vac. Sci. Technol., A, 1992, 10(5), 2961–2971 CAS
. - Y. Liang, N. Hilal, P. Langston and V. Starov, Interaction forces between colloidal particles in liquid: theory and experiment, Adv. Colloid Interface Sci., 2007, 134–135(21), 151–166 CrossRef CAS PubMed
. - J. Gregory, Approximate expressions for retarded van der waals interaction, J. Colloid Interface Sci., 1981, 83(1), 138–145 CrossRef CAS
. - J. N. Israelachvili, Van der waals forces between particles and surfaces, Intermolecular and surface forces, Elsevier, Amsterdam, 3rd edn, 2011, ch. 13, pp. 253–289 Search PubMed
. - J. N. Israelachvili, K. Kristiansen, M. A. Gebbie, D. W. Lee, S. H. Donaldson Jr, S. Das, M. V. Rapp, X. Banquy, M. Valtiner and J. Yu, The intersection of interfacial forces and electrochemical reactions, J. Phys. Chem. B, 2013, 117(51), 16369–16387 CrossRef CAS PubMed
. - R. Hogg, T. W. Healy and D. W. Fuerstenau, Mutual coagulation of colloidal dispersions, Trans. Faraday Soc., 1966, 62, 1638–1651 RSC
. - J. N. Israelachvili, Electrostatic forces between surfaces in liquids, Intermolecular and surface forces, Elsevier, Amsterdam, 3rd edn, 2011, ch. 14, pp. 291–340 Search PubMed
. - H. J. Butt, Measuring electrostatic, van der waals, and hydration forces in electrolyte solutions with an atomic force microscope, Biophys. J., 1991, 60(6), 1438–1444 CrossRef CAS PubMed
. - H. Guo, A simple algorithm for fitting a Gaussian function, IEEE Signal Process. Mag., 2011, 28(5), 134–137 CrossRef
. - J. Z. Wu, F. H. Liu, G. Chen, X. Wu, D. S. Ma, W. Zhang, H. Yang and J. B. Wang, Effect of ionic strength on the interfacial forces between oil/brine/rock interfaces: a chemical force microscopy study, Energy Fuels, 2016, 30(1), 273–280 CrossRef CAS
. - H. Wang, S. F. Chen, L. Y. Li and S. Y. Jiang, Improved method for the preparation of carboxylic acid and amine terminated self-assembled monolayers of alkanethiolates, Langmuir, 2005, 21(7), 2633–2636 CrossRef CAS PubMed
. - P. M. Dietrich, N. Graf, T. Gross, A. Lippitz, B. Schupbach, A. Bashir, C. Woll, A. Terfort and W. E. Unger, Self-assembled monolayers of aromatic ω-aminothiols on gold: surface chemistry and reactivity, Langmuir, 2010, 26(6), 3949–3954 CrossRef CAS PubMed
. - D. Nilsson, S. Watcharinyanon, M. Eng, L. Li, E. Moons, L. S. O. Johansson, M. Zharnikov, A. Shaporenko, B. Albinsson and J. Martensson, Characterization of self-assembled monolayers of oligo(phenyleneethynylene) derivatives of varying shapes on gold: effect of laterally extended pi-systems, Langmuir, 2007, 23(11), 6170–6181 CrossRef CAS PubMed
. - Y. Harada, T. Koitaya, K. Mukai, S. Yoshimoto and J. Yoshinobu, Spectroscopic characterization and transport properties of aromatic monolayers covalently attached to Si (111) surfaces, J. Phys. Chem. C, 2013, 117(15), 7497–7505 CAS
. - D. Y. Petrovykh, H. Kimurasuda, M. J. Tarlov and L. J. Whitman, Quantitative characterization of DNA films by X-ray photoelectron spectroscopy, Langmuir, 2004, 20(2), 429–440 CrossRef CAS PubMed
. - Y. Razvag, V. Gutkin and M. Reches, Probing the interaction of individual amino
acids with inorganic surfaces using atomic force spectroscopy, Langmuir, 2013, 29(32), 10102–10109 CrossRef CAS PubMed
. - A. Ooka and R. L. Garrell, Surface-enhanced Raman spectroscopy of DOPA-containing peptides related to adhesive protein of marine mussel, mytilus edulis, Biopolymers, 2000, 57(2), 92–102 CrossRef CAS
. - S. K. Burley and G. A. Petsko, Weakly polar interactions in proteins, Adv. Protein Chem., 1988, 39(2), 125–189 CrossRef CAS PubMed
. - P. D. M. Hatfield, Y. N. Palermo, J. Csontos, F. R. Murphy and S. Lovas, Quantum Chemical quantification of weakly polar interaction energies in the TC5b miniprotein, J. Phys. Chem. B, 2008, 112(11), 3503–3508 CrossRef PubMed
. - M. J. Plevin, D. L. Bryce and J. Boisbouvier, Direct detection of CH/pi interactions in proteins, Nat. Chem., 2010, 2(6), 466–471 CrossRef CAS PubMed
. - S. R. Chaudhari, S. Mogurampelly and N. Suryaprakash, Engagement of CF3 group in N–H⋯F–C hydrogen bond in the solution state: NMR spectroscopy and MD simulation studies, J. Phys. Chem. B, 2013, 117(4), 1123–1129 CrossRef CAS PubMed
.
Footnote |
† Electronic supplementary information (ESI) available. See DOI: 10.1039/c7ra04228k |
|
This journal is © The Royal Society of Chemistry 2017 |