DOI:
10.1039/C7RA03264A
(Paper)
RSC Adv., 2017,
7, 24215-24220
Perylene dye-functionalized silver nanoparticles serving as pH-dependent metal sensor systems†
Received
20th March 2017
, Accepted 19th April 2017
First published on 3rd May 2017
Abstract
Lysine-functionalized perylene was used to modify nanoparticles. Due to the benefits from a synergetic effect that originated between the perylene and silver nanoparticles, color-based metal sensor systems were established. At pH = 12.6, Cr6+ with a concentration of 100 nM could be detected by a color change from deep yellow to orange; after decreasing the pH to 12.0, the detection limit decreased to 2 μM. Interestingly, after further decreasing the pH to 11.0, another kind of metal ion (Cd2+) could be recognized with a detection concentration of 10 μM. At pH = 10.0, Pb2+ with a concentration of 2 μM could be detected.
1. Introduction
Recently, perylene diimide derivatives have received extensive attention due to their various applications in self-assembly,1 supramolecular polymerization,2 polymer solar cells,3 single wall carbon nanotubes,4 and organic photovoltaics.5 The properties of these materials are related not only to their own characteristics but also to their interactions with other materials.6 Therefore, the fabrication of perylene-based composites with tunable functions is of particular importance for making high performance materials.7 In addition to designing and synthesizing new compounds, improving and optimizing material compositions via nanotechnology is an effective and efficient strategy for maximizing material performance using available functional molecules.8 Nanometer-sized metal materials have attracted a great deal of attention because of their unique chemical and physical properties.9 Normally, the size, shape and surface modification of metal nanoparticles comprise some of the most important factors that can dramatically affect their properties.10 To improve their properties, many kinds of molecules have been used for the modification of nanoparticles, including surfactants,11 amino acids,12 and macromolecules.13–15 By virtue of these modification, nanoparticles possess various functions, including heavy metal ion detection.16 As we know, heavy metal ions pose a serious threat to human health and need to be detected.17,18 Among them, Cr6+ is considered as a severe environmental pollutant due to its carcinogenic nature.19 The major toxic effects of Cr6+ include chronic ulcers, dermatitis, and local effects in lungs.20 As another kind of toxic heavy metal ion, Cd2+ is extremely toxic even in low concentrations.21,22 Similarly, Pb2+ is a toxic substance in the environment due to its wide distribution and use in batteries, gasoline and pigments.23,24 Even a low level exposure to Pb2+ can cause memory loss, muscle paralysis and brain development inhibition, particularly in children, causing various neurotoxic effects.25 Currently, silver nanoparticles (AgNPs) provide a simple and low-cost solution for the rapid detection of heavy ions.26–30 However, in most cases, only one kind of heavy metal ion is detected using the above strategy. Moreover, a series of other reagents is also necessary for the selective detection of heavy metal ions.31–35 To the best of our knowledge, the use of perylene for modifying nanoparticles is still in its initial stages.36–41 Considering that the optical properties of perylenes depends on their state of aggregation, we envisage that the synergy between perylene and AgNPs will amplify the detection of AgNP-mediated color sensors. Here, the introduction of perylene results in pH-dependent metal sensor selectivity. It is found that the use of other reagents is not required. By simply adjusting the pH of the perylene solution, the as-prepared AgNPs can be used to detect toxic heavy metal ions. Cr6+ with a concentration of 100 nM can be detected by the naked eye at a pH = 12.6. Furthermore, Cd2+ (pH = 11.0, 10 μM) and Pb2+ (pH = 11.0, 2 μM) can be detected selectively by adjusting the pH.
2. Experiments
2.1 Chemicals and characterization
All the glassware used was thoroughly washed with fresh water and then rewashed with Milli-Q water and air dried. 1,6,7,12-Tetrachloroperylene 3,4,9,10-tetracarboxylic acid dianhydride was purchased from commercial sources and was of analytical or reagent grade. Lysine was purchased from Macklin. Other reagents were purchased from Sinopharm Chemical Reagent Co, Ltd (Shanghai) and used without further purification unless otherwise specified. Deionized water was used throughout the experiments. All the heavy metal salts were used to prepare stock solutions and were serially diluted to obtain lower concentrations. UV-vis absorption spectra were recorded using a UV-2501 spectrophotometer. The TEM images were obtained using a Philips TECNAI-12 instrument with an accelerating voltage of 120 kV. HRTEM and elemental mapping images were obtained using a Tecnai G2 F30 S-TWIN instrument. Light scattering measurements were conducted on a Malvern Nanosizer S instrument at room temperature.
2.2 Synthesis of silver nanoparticles at different pH values
The synthesis and structure characterization of the (lysine-perylene-lysine) LPL was reported elsewhere.42 LPL was initially dissolved in a NaOH solution to form a 200 μM stock solution at pH = 11. Subsequently, 0.15 mL of LPL (200 μM) dissolved in 3.85 mL of aqueous solution with pH values of 12.6, 12.0, 11.0, and 10.0. LPL-AgNPs were synthesized by the reduction of AgNO3 in the presence of LPL. In a typical experiment, AgNO3 (0.5 mL, 10 mM) was added into the solution of LPL (20.0 mL, 7.5 μM) at given pH. Subsequently, a fresh NaBH4 solution (0.5 mL, 0.1 M) was added into the solution with rapid stirring.
2.3 Colorimetric detection of various heavy metal ions
A variety of aqueous solutions of ions were added into the as-prepared LPL-AgNP solutions. At pH = 12.6, the final concentration of the individual metal ion solutions was 100 nM. At pH = 12.0, the final concentration of the individual metal ion solutions was 2 μM. At pH = 11.0, the final concentration of the individual metal ion solutions was 10 μM. At pH = 10.0, the final concentration of the individual metal ion concentrations was 2 μM.
3. Results and discussion
3.1 Fabrication of perylene capped silver nanoparticles
Scheme 1 shows the chemical structure of the lysine-functionalized perylene (LPL). LPL possess a bolaamphiphile structure with a hydrophobic rigid perylene backbone as the skeleton and pH-responsive lysine residue as the hydrophilic head. LPL-capped AgNPs (LPL-AgNPs) were prepared by NaBH4 reduction, using LPL as the protective ligand. After the synthesis of the LPL-AgNPs, one lysine residue end of the LPL was linked to the AgNPs through –COO− and –NH2 groups, and another lysine residue from the LPL protected them from aggregation due to the negatively charged –COO− electrostatic repulsions under alkaline conditions.
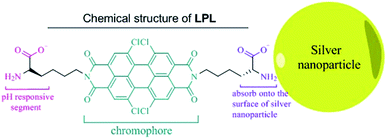 |
| Scheme 1 Chemical structure of the LPL at pH > 10. | |
Fig. 1 presents TEM images and histograms of the size distribution of the LPL-AgNPs at different pH levels, revealing that the obtained AgNPs were formed and well dispersed at pH 12.6, 12.0, 11.0, and 10.0 (Fig. 1d). The average diameters and standard deviations determined from their histograms were 7.5 ± 2.4 nm, 6.3 ± 2.3 nm, 3.2 ± 1.7 nm, and 7.0 ± 2.2 nm, respectively (Fig. 1 inset). The UV-vis spectra show the absorption band was centered at ca. 390 nm, confirming the formation of LPL-modified AgNPs, which are consistent with TEM results. The LPL-AgNPs were not obtained at pH > 12.6. Thus, pH = 12.6 was the highest value in our study (Fig. S1†).
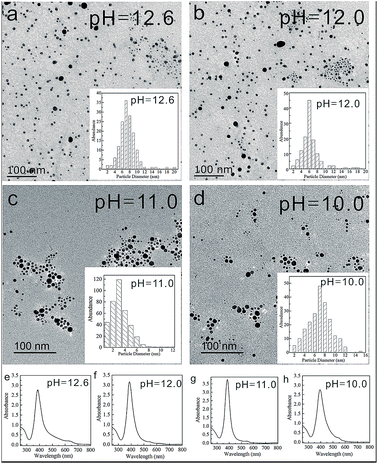 |
| Fig. 1 TEM images of the LPL-AgNPs at pH (a) 12.6, (b) 12.0, (c) 11.0, and (d) 10.0. (Inset) Corresponding histograms of the size distribution and (e)–(h) corresponding UV-vis spectra. | |
3.2 LPL-AgNPs as metal ion sensors at different pH values
When metal ions were added to the LPL-AgNPs, metal ions could bind to lysine residue easily and act as a crosslinking agent for the LPL-AgNPs, thereby inducing aggregation of the AgNPs. Subsequently, the metal ion detection ability of the as-prepared LPL-AgNPs at different pH values was studied. At pH = 12.6, the LPL-AgNPs were studied separately for each of the metal ions, including monovalent ions (Na+ and K+), divalent ions (Mn2+, Cd2+, Pb2+, Ba2+, Zn2+ and Ca2+), trivalent ions (Al3+) and hexavalent ions (Cr6+) at a fixed concentration (100 nM). As shown in Fig. 2a, the LPL-AgNPs turned from orange brown to orange after the addition of Cr6+, whereas the LPL-AgNPs remained orange brown upon interacting with interfering ions. Compared to the other ions, which had no obvious effect on the absorbance of the LPL-AgNPs, the absorbance after the addition of Cr6+ was dramatic decreased (Fig. S2a,† red line). The only difference is that the ratio of A545/384 for the Cr6+ was close to 0.30, which was higher than that of other metal ions (Fig. S2e†). After decreasing the pH to 12.0, a similar selectivity for Cr6+ was observed but the detection limit decreased to 2 μM (Fig. 2b).
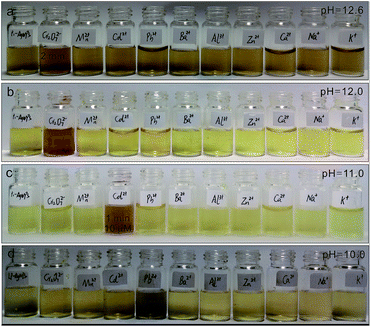 |
| Fig. 2 (a) Digital images of the LPL-AgNPs after the addition of different metal ions (100 nM) at pH = 12.6. (b) Digital images of the LPL-AgNPs after the addition of different metal ions (2 μM) at pH = 12.0. (c) Digital images of the LPL-AgNPs after the addition of different metal ions (10 μM) at pH = 11.0. (d) Digital images of LPL-AgNPs after the addition of different metal ions (2 μM). | |
The peak at approximately 390 nm decreased obviously, and a new peak at approximately 500 nm appeared (Fig. S2b,† red line). The ratio of A503/390 for Cr6+ (ca. 0.60) was much higher than others (Fig. S2f†), demonstrating that the LPL-AgNPs had a very high specificity toward Cr6+ in strong basic conditions. Further decreasing the pH to 11.0, the LPL-AgNPs showed a selective color change from yellow to dark orange after the addition of Cd2+ (Fig. 2c, detection limit: 10 μM), and the peak at approximately 388 nm decreased slightly (Fig. S2c,† pink line). It was found that while the ratio change existed for the other metal ions (Fig. S2g†), the ratio of A551/388 for Cd2+ was approximately 0.16, which was much higher than that of other metal ions (below 0.07). For the LPL-AgNPs at pH = 10.0, the color changed to dark after the addition of Pb2+ (Fig. 2d, detection limit: 2 μM), and the peak at approximately 390 nm decreased obviously (Fig. S2d,† green line). The ratio of A548/388 for Pb2+ was approximately 0.15 (higher than that of the other metal ions), revealing that the LPL-AgNPs had a very high specificity toward Pb2+ (Fig. S2h†). It should be noted that, as long as the action time is long enough, the addition of most ions can cause discoloration.
To obtain further insight into the color change at a given pH, Cr6+ solutions with different concentrations were added into the LPL-AgNP solutions (pH = 12.6). As shown in Fig. 3a, a fine gradation in the color development from brown to dark orange was recorded. TEM images of the LPL-AgNPs after the addition of Cr6+ with concentrations of 20 μM, 5 μM, 2 μM, 500 nM, 200 nM, and 100 nM, as shown in Fig. 3b–g, further confirm that the observed color changes were the result of LPL-AgNP aggregation induced by Cr6+ (pink circles). The DLS data clearly indicate that the hydrodynamic diameter increased as a function of the Cr6+ concentration (Fig. 3h), which was consistent with color changes. The UV-vis spectra were examined at 0, 5, 10, 30, and 60 min, as shown in Fig. 3i. As the incubation time increased, the absorption at 384 nm continuously decreased and a new absorption band appeared at approximately 545 nm, which was caused by the coordination between Cr6+ and the LPL-AgNPs.
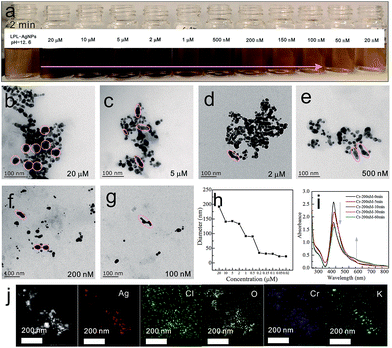 |
| Fig. 3 (a) Digital image of LPL-AgNPs interacting with different concentrations of Cr6+. (b)–(g) TEM images of the LPL-AgNPs after interacting with Cr6+ at concentrations of 10 μM, 2 μM, 200 nM, and 100 nM. (h) Evolution of the diameter of the LPL-AgNPs. (i) UV-vis spectra of the LPL-AgNPs with time. (j) Left: STEM image of the LPL-AgNPs-Cr6+. Right: the corresponding mapping images. | |
It should be mentioned that excess reducing borohydride in the LPL-AgNPs solution was responsible for reducing Cr6+ to Cr3+, which in turn interacted with the LPL-AgNPs. Thus, it was deduced that Cr3+ instead of Cr6+ was participating in the coordination.43 The elemental mapping analysis indicated a homogeneous distribution of Cl and O, which are characteristic elements of the LPL (Fig. 3j), providing further evidence of the LPL-capped AgNP formation. Importantly, Cr6+-induced aggregations were evidenced by the homogeneous distribution of Cr and K (from K2Cr2O7) across the aggregates (Fig. S3†).
After, different concentrations of Cd2+ solutions ranging from 20 μM to 20 nM were added into the LPL-AgNP solutions (pH = 11.0). Fig. 4a shows that the color changed from yellow to brown after the addition of Cd2+, and aggregates were formed after interacting with Cd2+ (Fig. 4b and c). The DLS data indicate that the hydrodynamic diameter increased as a function of the Cd2+ concentration (Fig. 4d); the UV-vis spectra demonstrate that the aggregation behavior of the LPL-AgNPs was highly dependent on the incubation time (Fig. 4e). The elemental mapping analysis indicated a homogeneous distribution of Cl and O, which are characteristic elements of the LPL (Fig. 4f and S4†).
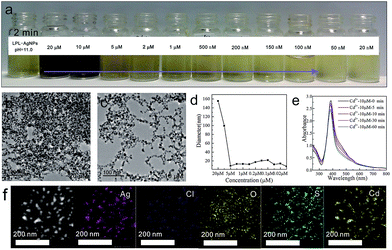 |
| Fig. 4 (a) Digital image of LPL-AgNPs interacting with Cd2+. (b and c) TEM images of the LPL-AgNPs after interacting with Cd2+ at concentrations of 20 μM and 10 μM. (d) Evolution of the diameter of the LPL-AgNPs. (e) UV-vis spectra of the LPL-AgNPs with time. (f) Left: STEM image of the LPL-AgNPs-Cd2+. Right: the corresponding mapping images. | |
More importantly, Cd2+-induced aggregations were indicated by the homogeneous distribution of Cd and S (from CdSO4) across the aggregates. Interestingly, after decreasing the pH to 10.0, the LPL-AgNPs shifted their selective detection ability to Pb2+. As shown in Fig. 5a, a fine gradation in the color development was recorded from yellow to slight brown after the addition of Pb2+. It was observed that after interacting with the Pb2+ solution (20 μM), large aggregates in size range from 90 nm to 100 nm were formed (Fig. 5b and c). The above changes were attributed to the Pb2+ induced aggregation (green circles), which revealed fused particles with sizes that depended on the concentration of Pb2+. The DLS data clearly show aggregation of the LPL-Ag NPs as a function of the Pb2+ concentration (Fig. 5d), which was responsible for the gradation of the color development. The change in the intensity of the absorbance was monitored and captured by the UV–vis spectra, as shown in Fig. 5e. The absorbance centered at 390 nm shifted to the right, forming a distinct second peak at approximately 550 nm. This second peak could be ascribed to the complex formed due to the aggregation of the particles. The elemental mapping analysis demonstrated a homogeneous distribution of Cl and O (Fig. 5f). Furthermore, Pb2+-induced aggregations were evidenced by the homogeneous distribution of Pb2+ across the aggregates (Fig. S5†).
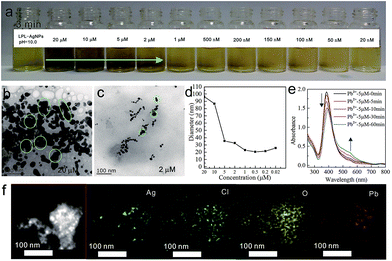 |
| Fig. 5 (a) Digital image of LPL-AgNPs interacting with different concentrations of Pb2+. (b and c) TEM images of LPL-AgNPs after interacting with Pb2+ at the concentrations of 20 μM and 2 μM. (d) Evolution of the diameter of the LPL-AgNPs with the concentration. (e) UV-vis spectra of LPL-AgNPs with time. (f) Left: STEM image of the LPL-AgNPs-Pb2+. Right: the corresponding mapping images. | |
3.3 Mechanism
The driving force for the metal ion-probe affinity might be coordination interaction. Take the interaction between Cd2+ and LPL for example. For the powder sample (have no interaction with heavy metal ions), the peak of C
O and C–N bond was observed at 1660 cm−1 and 1176 cm−1. After the interaction with heavy metal ions, the C
O and C–N bond was decreased to 1626 cm−1 and 1095 cm−1, respectively (Fig. S6†). The decrease of wave numbers demonstrated the interaction of amino and carboxyl with Cd2+.44 More importantly, the selective color changes could be explained well by the metal ion mediated aggregation at a given pH. To determine the essential factor of the as-prepared sensor system, a series of control experiments was conducted, which demonstrated that efficient detection could not be realized by lysine, LPL, or lysine-modified AgNPs alone. As shown in Fig. S7b,† the lysine solution did not show any response before and after the addition of Cr6+ at pH = 12.6 (Fig. S7a†). Similarly, the lysine-functionalized perylene solution exhibited a colorless appearance before and after the addition of Cr6+ at pH = 12.6. Furthermore, the LPL exhibited no selective colorimetric sensing in response to all the ions in an aqueous solution at pH = 12.0. The interactions between Cr6+ with lysine and Cr6+ with LPL at other pH values (12.0, 11.0, and 10.0) was similar to the interactions at pH = 12.6 (Fig. S8–S10†). Furthermore, the lysine-modified AgNPs exhibited no selective colorimetric sensing in response to all the ions in aqueous solutions at pH > 12.0, pH = 11.0, and pH = 10.0. It is should be mentioned that the lysine-AgNPs could not be obtained at pH > 12.0. Thus, the detection ability of the lysine-AgNPs at only pH = 11.0 and pH = 10.0 was investigated. At pH = 11.0, after the addition of metal ions into the lysine-AgNP solution, no selective colorimetric sensing occurred. For a continuous decrease in the concentration of metal ions to 100 nM, no color change was observed among all the different ions (Fig. S11–S12†).
As shown in Fig. 6a, at pH = 12.6, no obvious absorbance decrease was observed, except for Cr6+ and Cr3+, resulting in the selective detection ability for Cr6+ at short time scales. At pH = 12.0, a dramatic decrease was only observed for Cr6+ and Cr3+ (Fig. 6b), demonstrating that the detection arises from the fast kinetic rate. Similarly, at pH = 11.0 and 10.0, when compared with the other metal ions, the LPL-AgNPs-M (M = Cd2+, at pH = 11.0; M = Pb2+, at pH = 10.0) showed a consistent absorbance decrease (Fig. 6c and d), which resulted from the different kinetic rates. The different coordination rates might have resulted from the different states at each given pH. It is well known that the dissociation constant of –COO− is influenced by different pH values. As we know, pKa1 = 2.18, pKa2 = 8.95, and pKa3 = 10.53. Furthermore, it is reported that all carboxyl groups are ionized to –COO− at pH > 10, the difference is that the ionization state of the amine at different pH.45 Based on the literature and calculation, at pH = 10, –NH3+ (40%) and –NH2 (60%) coexisted, increase the pH to 11, it is deduced that –NH3+ (<40%) and –NH2 (>60%) coexisted, at pH > 12, all the existed as –NH2. The fluorescence spectra of the LPL, LPL-AgNPs, and LPL-AgNPs-M (Cr6+, Cd2+, and Pb2+) were recorded to determine the LPL state.
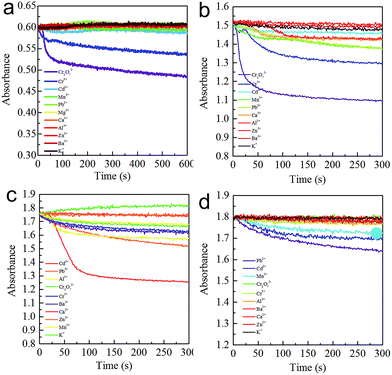 |
| Fig. 6 Aggregation kinetics of the various metal ions at pH (a) 12.6, (b) 12.0, (c) 11.0, and (d) 10.0. | |
As shown in Fig. S13a and b,† the LPL exhibited only one peak at pH = 12.6 and 12.0, which indicated that the complete dissociation of the carboxyl groups occurred and the LPL existed as a free molecule, in turn, facilitating the interaction between metal ions and AgNPs. However, the LPL exhibited two peaks at pH = 11.0 and 10.0, indicating that the LPL existed as a slightly aggregated state42 at this condition, which might have decreased the detection limit of the AgNPs. Based on above results, we deduced the selective color sensor mechanism. As shown in Scheme 2 (Fig. S14†), at strong basic conditions, Cr6+ was reduced to Cr3+, which can act as a crosslinking agent for the LPL-AgNPs. Negative carboxylate anions of the LPL on the AgNP surface can be attracted together by a carboxylate-Cr3-carboxylate linker. One Cr3+ could be chelated with three LPL molecules by one –NH2 and one –COO− group from each LPL molecule at pH 12.6 and 12.0.46 At pH = 10.0, –NH3+ (40%) and –NH2 (60%) coexisted,45 it is deduced that LPL could easily coordinate with Pb2 at this condition. At pH = 11.0, –NH3+ (<40%) and –NH2 (>60%) coexisted (the ratio was calculated based on the literature45), it is supposed that this difference might result in different kinetic when reacting with Cd2+. As mentioned above, the detection limit for Cr6+ is 100 nM at pH = 12.6 and 2 μM at pH = 12.0. Furthermore, the detection limit for Cd2+ is 10 μM at pH = 11.0 and detection limit for Pb2+ is 10 μM at pH = 11.0. Compared with the literature,26,35 different ions could be detected by changing the pH of LPL, but the detection sensitivity is not as good as reported in the literature. Considering various reagents were used in the reported system, complicated adjustment could be conducted for improving the detection limit is different from our system.
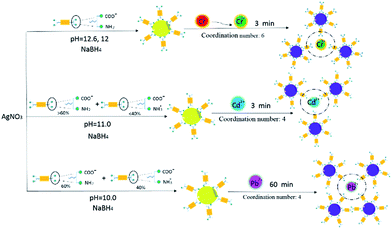 |
| Scheme 2 Possible mechanisms of the interactions between the metal ions and LPL-AgNPs. The ratio (>60%, and <40% at pH = 11.0) was calculated based on the literature.44 | |
4. Conclusions
Herein, we synthesized LPL-AgNPs as probes for toxic metal ions with high sensitivity and selectivity. LPL endowed as-prepared silver nanoparticles with pH-dependent properties, exhibiting selective colorimetric sensing of toxic Cr6+, Cd2+, and Pb2+ metal ions in aqueous solutions at different pH values. More importantly, the synergetic effect between the lysine-functionalized perylene and AgNPs amplified the color change and then improved the pH-dependent selectivity. In view of the simple modification, low cost, user-friendliness and bare-eye visual readout, these perylene modified AgNPs have the potential to be used for the detection of Cr6+, Cd2+, and Pb2+.
Acknowledgements
This work was supported by the National Natural Science Foundation of China (21503185 and 21273195), China Postdoctoral Science Foundation (137070124). Electronic Supplementary Information (ESI) is available: characterizations of nanoparticles detection.
References
- M. Frank, J. Ahrens, I. Bejenke, M. Krick, D. Schwarzer and G. H. Clever, J. Am. Chem. Soc., 2016, 138, 8279–8287 CrossRef CAS PubMed.
- S. Ogi, V. Stepanenko, J. Thein and F. Würthner, J. Am. Chem. Soc., 2016, 138, 670–678 CrossRef CAS PubMed.
- L. Nian, W. Zhang, N. Zhu, L. Liu, Z. Xie, H. Wu, F. Würthner and Y. Ma, J. Am. Chem. Soc., 2015, 137, 6995–6998 CrossRef CAS PubMed.
- Y. Tsarfati, V. Strauss, S. Kuhri, E. Krieg, H. Weissman, E. Shimoni, J. Baram and D. Guldi, J. Am. Chem. Soc., 2015, 137, 7429–7440 CrossRef CAS PubMed.
- S. Holliday, R. S. Ashraf, C. B. Nielsen, M. Kirkus, J. A. Röhr and C. H. Tan, J. Am. Chem. Soc., 2015, 137, 898–904 CrossRef CAS PubMed.
- Y. Sun, C. G. Yan, Y. Yao, Y. Han and M. Shen, Adv. Funct. Mater., 2008, 18, 3981–3990 CrossRef CAS.
- Y. Sun, W. Fu, Z. Li and Z. Wang, Langmuir, 2014, 30, 8615–8620 CrossRef CAS PubMed.
- Y. Sun, F. Guo, T. Zuo, J. Hua and G. Diao, Nat. Commun., 2016, 7, 12042 CrossRef CAS PubMed.
- A. Henglein, Chem. Rev., 1989, 89, 1861–1873 CrossRef CAS.
- Z. Niu and Y. Li, Chem. Mater., 2014, 26, 72–83 CrossRef CAS.
- M. Shen, Y. Sun, Y. Han, R. Yao and C. G. Yan, Langmuir, 2008, 24, 13161–13167 CrossRef CAS PubMed.
- D. Li, Y. Dong, B. Y. Li, Y. Wu, K. Wang and S. C. Zhang, Analyst, 2015, 140, 7672–7677 RSC.
- Y. Sun, Y. Yao, C. G. Yan, Y. Han and M. Shen, ACS Nano, 2010, 4, 2129–2141 CrossRef CAS PubMed.
- Y. Yao, M. Xue, M. Z. Zhang, Y. Wang and F. Huang, Chem. Sci., 2013, 4, 3667 RSC.
- Y. Yao, Y. Wang and F. Huang, Chem. Sci., 2014, 5, 4312 RSC.
- Y. Gao, J. Xin, Z. Shen, W. Pan, X. Li and A. Wu, Sens. Actuators, B, 2013, 181, 288–293 CrossRef CAS.
- L. Zhang, C. Xu and B. Li, Microchim. Acta, 2009, 166, 61–68 CrossRef CAS.
- B. Valeura and L. Leray, Coord. Chem. Rev., 2000, 205, 3–40 CrossRef.
- H. G. Preuss, B. Echard, N. V. Perricone, D. Bagchi, T. Yasmin and S. J. Stohs, J. Inorg. Biochem., 2008, 102, 1986–1990 CrossRef CAS PubMed.
- R. F. M. Herber and M. Stoeppler, Trace Element Analysis in Biological Specimens, Vol. 15,(Techniques and Instrumentation in Analytical Chemistry), Elsevier Science, 1st edn, 1994 Search PubMed.
- S. Haouema, N. Hmada, F. M. Najjarb, E. A. Hania and R. Saklyc, Exp. Toxicol. Pathol., 2007, 59, 77–80 CrossRef PubMed.
- C. D. Klaassen, J. Liu and S. Choudhuri, Annu. Rev. Pharmacol., 1999, 39, 267 CrossRef CAS PubMed.
- W. Jedrychowski, F. Perera, J. Jankowski, V. Rauh, E. Flaka, K. L. Caldwelld, R. L. Jonesd, A. Paca and I. Lisowska-Miszczyk, Int. J. Hyg. Environ. Health, 2008, 211, 345–351 CrossRef PubMed.
- B. Valeura and L. Leray, Coord. Chem. Rev., 2000, 205, 3–40 CrossRef.
- H. Needleman, Annu. Rev. Med., 2004, 55, 209–222 CrossRef CAS PubMed.
- Y. Zhou, H. Zhao, C. Li, P. He, W. B. Peng, L. F. Yuan, L. X. Zeng and Y. J. He, Talanta, 2012, 97, 331–335 CrossRef CAS PubMed.
- N. Singh, N. Kaur, C. N. Choitir and J. F. Callan, Tetrahedron Lett., 2006, 50, 4201–4204 CrossRef.
- R. Hu, L. Zhang and H. B. Li, New J. Chem., 2014, 38, 2237–2240 RSC.
- Y. Gao, J. Xin, Z. Shen, W. Pan, X. Li and A. Wu, Sens. Actuators, B, 2013, 181, 288–293 CrossRef CAS.
- F. Kim, J. H. Song and P. Yang, Photochemical, J. Am. Chem. Soc., 2002, 124, 14316 CrossRef CAS PubMed.
- M. Zhang, Y. Q. Liu and B. C. Ye, Analyst, 2012, 137, 601–607 RSC.
- Z. Chen, L. Chu, C. Liu and S. Luo, J. Nanosci. Nanotechnol., 2015, 15, 1480–1485 CrossRef CAS PubMed.
- J. Xin, F. Zhang, Y. Gao, Y. Feng, S. Chen and A. Wu, Talanta, 2012, 101, 122–127 CrossRef CAS PubMed.
- T. Wu, C. Liu, K. J. Tan, P. P. Hu and C. Z. Huang, Anal. Bioanal. Chem., 2010, 397, 1273–1279 CrossRef CAS PubMed.
- W. Jin, P. Huang, F. Wu and L. H. Ma, Analyst, 2015, 140, 3507–3513 RSC.
- D. L. Liao, J. Chen, H. P. Zhou, Y. Wang, Y. X. Li and C. Yu, Anal. Chem., 2013, 85, 2667–2672 CrossRef CAS PubMed.
- Y. Wang, J. Chen, Y. Chen, W. Y. Li and C. Yu, Anal. Chem., 2014, 86, 4371–4378 CrossRef CAS PubMed.
- H. P. Jiao, J. Chen, W. Y. Li, F. Y. Wang, H. P. Zhou, Y. X. Li and C. Yu, ACS Appl. Mater. Interfaces, 2014, 6, 1979–1985 CAS.
- R. Hu, T. Liu, X. B. Zhang, S. Y. Huan, C. Wu, T. Fu and W. H. Tan, Anal. Chem., 2014, 86, 5009–5016 CrossRef CAS PubMed.
- A. K. Dwivedi, M. Pandeeswar and T. Govindaraju, ACS Appl. Mater. Interfaces, 2014, 6, 21369–21379 CAS.
- Y. Y. Lin, R. Chapman and M. M. Stevens, Anal. Chem., 2014, 86, 6410–6417 CrossRef CAS PubMed.
- Y. Sun, Z. Li and Z. Wang, J. Mater. Chem., 2012, 22, 4312–4318 RSC.
- A. Ravindrana, M. Elavarasia, T. C. Prathna, A. M. Raichur, N. Chandrasekarana and A. Mukherjee, Sens. Actuators, B, 2012, 365–371 CrossRef.
- K. Fujiwara, A. Ramesh, T. Maki, H. Hasegawa and K. Ueda, J. Hazard. Mater., 2007, 146, 39–50 CrossRef CAS PubMed.
- N. Kitadai, T. Yokoyama and S. Nakashima, J. Colloid Interface Sci., 2009, 329, 31–37 CrossRef CAS PubMed.
- C. A. McAuliffe, J. V. Quagliano and L. M. Vallarino, Inorg. Chem., 1966, 5, 1997–2003 CrossRef.
Footnote |
† Electronic supplementary information (ESI) available. See DOI: 10.1039/c7ra03264a |
|
This journal is © The Royal Society of Chemistry 2017 |