DOI:
10.1039/C7RA02386C
(Paper)
RSC Adv., 2017,
7, 25549-25559
Substituent-tuned structure and luminescence sensitizing towards Al3+ based on phenoxy bridged dinuclear EuIII complexes†
Received
26th February 2017
, Accepted 29th April 2017
First published on 12th May 2017
Abstract
To develop a LnIII complex-supported chemsensor, two new phenoxy bridged dinuclear EuIII complexes, [Eu2(H2L)3(NO3)3]·3CH3CN (EuL) and [Eu2(H2L′)2(NO3)4]·3CH3CN (EuL′), constructed by two new structurally related salicylamide salen-like ligands, 1-(2-hydroxy-benzamido)-2-(2-hydroxy-5-nitro-benzylideneamino)-ethane (H3L) and 1-(2-hydroxy-benzamido)-2-(2-hydroxy-4-diethylamino-benzylideneamino)-ethane (H3L′), have been synthesized and structural analysis shows that the different substitution groups on 2-(iminomethyl)phenol moiety have significant effects on their structures. Upon excitation of the ligand-centered absorption band at 375 nm, emissions both originating from ligands and EuIII ions were observed in the two EuIII compounds with the EuIII-centered emission intensity more than three times higher than that of ligand-centered emission. The capability of EuL and EuL′ for selective detection Al3+ ions were evaluated and the results indicate EuL exhibits a turn-on luminescent enhancement as high as 5.7 fold with Kd = 1.53 × 10−4 in CH3CN, but comparable compound EuL′ could not detect Al3+ among various cations. The considerably ‘turn-on’ luminescence response of EuL concomitantly led to the apparent color change from reddish to brilliant red, which could also be identified by naked eyes easily under UV lamp. This luminescence enhanced response can be explained in terms of the decrease of non-radiative transitions in EuL in addition to excited-state intra-molecular proton transfer (ESIPT) and photo-induced electron transfer suppression upon Al3+ coordination which is also rationalized by a theoretical calculation.
1 Introduction
Luminescent lanthanide complexes display unrivalled spectroscopic properties, which place them in a special category in the luminescent toolbox. The attractive luminescence properties, especially, their characteristic line-like sharp emission bands which are unaffected by the LnIII coordination environment make LnIII luminescence appealing for a broad range of practical applications such as bioanalysis and biological imaging,1 light conversion devices,2 display materials,3 and luminescent probes.4 Since most of these f → f transitions are forbidden by the Laporte rule, the direct excitation of luminescent lanthanide(III) cations is inefficient. However, this limitation can be overcomed with the help of a highly absorbing “antenna” located in a sufficiently close proximity to the LnIII.5 Once a LnIII-centred excited state has been reached via the antenna effect, the rules for radiative de-excitation are the same and the probability is weak. As a consequence, the excited-state lifetimes of LnIII can be extremely long, up to a few milliseconds for visible EuIII and TbIII emitters.
Aluminum, a group 3 metal, is the third most abundant metal element in the crust of the earth, accounting for approximately 8% of its mass. It is well-known that aluminum is extensively used in our daily life and is also one important metal element applied in industry production. However, Al3+ is toxic and environmentally harmful. Due to the frequent use of aluminum vessels and foil, the risk of Al3+ ions absorption by the human body is increasing. For example, the iron-binding protein can carry Al3+ ions to the brain, which can further hurt the central nervous system. Al3+ has been implicated as the fatal factor in Alzheimer's disease, and it also prevents plant growth on acid grounds. The World Health Organization (WHO) prescribed the average human intake of aluminium is about 3–10 mg per day with a weekly dietary intake of 7 mg per day bodyweight.6 Thus, it is urgent for development of selective and sensitive Al3+ chemsensors. Up to now, some fluorescent chemosensors have been designed for detection of Al3+.7 However, the fluorescence lifetimes of typical organic compounds, including common aluminum-selective fluorescent sensor molecules, are in the nanosecond region. On the other hand, among numerous sensors, luminescent lanthanide complexes, in particular EuIII and TbIII complexes receiving enormous interest due to their excellent optical properties in aid of an “antenna effect”, such as large Stokes shifts and high color purity. An efficient strategy to prepare lanthanide complexes for detecting metal ion is to use lanthanide compounds with nonbonded functional ligand sites, where the lanthanide compounds provides luminescence, and the nonbonded functional sites interact with the metal ions. However, immobilization of functional site within the lanthanide compounds has been challenging due to their high reactivity during the synthesis. Up to now, only a few investigations on LnIII complex based chemsensors with luminescence enhancement have been carried out to detect Zn2+,8 Ag+,9 K+,10 as well as some anions.11 These chemosensors were designed profiting from an analyte-induced alteration of antenna, shortening distance between the antenna and LnIII or ligand exchange in solution. To our best knowledge, chemosensors based on LnIII complexes are unprecedented for Al3+ because of the poor coordination ability and strong hydration aptitude of Al3+. Inspired by these cases together with a continuation of our interest in the design of luminescent LnIII complexes,12 we dedicate to develop a chemsensor based on LnIII complexes of salicylamide salen-like ligands by ingenious ligand design. Considering nitryl and diethamino group possess weaker binding abilities toward metal atoms than imine and amide group, when it situated within a highly electron-rich conjugate system, the lone pair electrons of N atoms can be spread and thereby realize the effective transfer of electrons and further influence the optical properties through environmental perturbation. Therefore, introduction of nitryl or diethylamino into salen-like salicylamide ligands may be a highly promising platform for construction of luminescent lanthanide chemsensor. We expect that metal ion binding to unoccupied coordination site of a LnIII complex constructed by the salicylamide salen-like ligand would be conducive to a large luminescent response through the modulation of the sensitization process. Therefore, in this contribution, two new phenoxy bridged dinuclear EuIII compounds, [Eu2(H2L)3(NO3)3]·3CH3CN (EuL) and [Eu2(H2L′)2(NO3)4]·2CH3CN (EuL′) based on two structurally related salicylamide salen-like ligands were prepared by solvent evaporation method. Both the structural and luminescent investigations revealed that the different push–pull electronic substitution group on 2-(iminomethyl)phenol moiety of the salicylamide salen-like ligands have significant effect on their structures as well as luminescence responses toward Al3+. We found that EuL exhibits a turn-on luminescent enhancement as high as 5.7 fold with Kd = 1.53 × 10−4 in CH3CN, but comparable compound EuL′ could not detect Al3+ under the same experimental conditions. To the best of our knowledge, EuL is the first luminescence-enhanced EuIII complex-supported Al3+ sensor. The sensing mechanism is presumably due to the formation of EuL·Al which result in effective suppression of non-radiative transitions, excited-state intra-molecular proton transfer (ESIPT) and photo induced electron transfer.
2 Experimental
2.1 Materials and physical measurements
Carbon, nitrogen and hydrogen analyses were performed using an EL elemental analyzer. Melting point were determined on a Kofler apparatus. 1H NMR spectra of the ligands were recorded in d6-DMSO solution at room temperature on a Bruker 400 instrument operating at a frequency of 400 MHz and referenced to tetramethylsilane (0.00 ppm) as an internal standard. Chemical shift multiplicities are reported as s = singlet, d = doublet, t = triplet and m = multiplet. Infrared spectra were obtained with KBr discs on a Nicolet FT-170SX instrument in the wavenumber range of 4000–400 cm−1 with an average of 128 scans and 4 cm−1 of spectral resolution. ESI-MS were determined on a HRMS spectrometer (model: QTOF Micro YA263). UV-vis absorption spectra were determined with a Varian UV-Cary100 spectrophotometer. Luminescence spectra were recorded on a F-7000 fluorescence spectrophotometer (Japan Hitachi company) at room temperature. The lifetime and quantum yield were measured at room temperature on FLS920 Steady State &Time-resolved fluorescence Spectrometer (Edinburgh Instrument).
2.2 Synthesis of the ligand
The synthesis of 1-(2-hydroxy-benzamido)-2-(2-hydroxy-5-nitro-benzylideneamino)-ethane (H3L) and 1-(2-hydroxy-benzamido)-2-(2-hydroxy-4-diethylamino-benzylideneamino)-ethane (H3L′) are according to literature13 with minor modification and using 2-hydroxy-5-nitrobenzaldehyde and 2-hydroxy-4-diethylaminobenzaldehyde instead (Scheme 1).
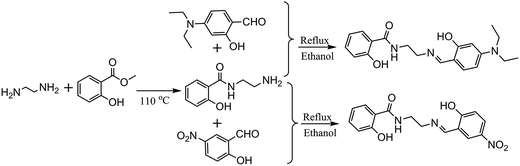 |
| Scheme 1 The synthesis route of the ligand H3L and H3L′. | |
H3L. Yellow solid. Mp 212–213 °C. Analytical data, calc. for C16H15N3O5: C, 58.36; H, 4.59; N, 12.76; found: C, 58.81, H, 5.57, N, 12.79; IR (KBr, υ, cm−1): 3381 (w), 1658 (s), 1636 (s), 1517 (s), 1452 (m), 1373 (m), 1306 (s), 1236 (m), 1092 (m), 821 (m), 748 (m), 495 (m). 1H NMR (d6-DMSO, 400 MHz): δ: 3.66 (m, 2H, CH2), 3.87 (m, 2H, CH2), 6.67 (d, 1H, ArH), 6.89 (m, 2H, ArH), 7.40 (m, 1H, ArH), 7.81 (m, 1H, ArH), 8.05 (m, 1H, ArH), 8.41 (s, 1H, CH
N), 9.00 (t, 1H, NH, J = 4 Hz), 12.33 (s, 1H, OH), 14.19 (s, 1H, OH).
H3L′. Pale solid. Mp 145–146 °C. Analytical data, calc. for C20H25N3O3: C, 67.58; H, 7.09; N, 11.82; found: C, 67.57, H, 7.07, N, 11.84; IR (KBr, υ, cm−1): 3292 (w), 3081 (w), 2974 (m), 2924 (m), 1656 (s), 1517 (s), 1354 (s), 1267 (s), 1215 (s), 1143 (s), 1010 (s), 954 (w), 864 (w), 761 (m), 648 (m), 603 (m), 594 (m). 1H NMR (d6-DMSO, 400 MHz): δ: 1.04 (t, 6H, CH3), 3.29 (m, 4H, CH2), 3.51 (m, 2H, CH2), 3.62 (t, 2H, CH2), 5.90 (d, 1H, ArH), 6.13 (dd, 1H, ArH), 6.84 (m, 2H, ArH), 7.04 (d, 1H, ArH), 7.33 (m, 1H, ArH), 7.79 (dd, 1H, ArH), 8.18 (s, 1H, CH
N), 8.89 (t, 1H, NH).
2.3 Synthesis of EuL and EuL′
General procedure. To a acetonitrile solution of 0.1 mmol H3L/H3L′, 27 uL (0.2 mmol) triethylamine was added to make a clear solution. Then 0.1 mmol Eu(NO3)3·6H2O (0.446 mg) was added as a solid, and the solution was stirred for another 4 h to obtain a clear solution which was filtered into a sealed 10–20 mL glass vial for crystallization at room temperature. After about three weeks, pale yellow single crystals suitable for crystal analysis were obtained and collected by filtration, washed with cold acetonitrile, and dried in the air.[Eu2(H2L)3(NO3)3]·3CH3CN (EuL): (yield: 36.7 mg, 52% based on Eu(NO3)3·6H2O). Analytical data (%), calcd: C, 41.47; H, 2.90; N, 11.64; found: C, 41.22; H, 2.88; N, 11.68; IR (KBr, υ, cm−1): 3456 (w), 1606 (s), 1511 (s), 1470 (s), 1446 (s), 1383 (m), 1217 (s), 1168 (s), 1130 (m), 1074 (m), 734 (m), 594 (m).
[Eu2(H2L′)2(NO3)4]·2CH3CN (EuL′): (yield: 37.6 mg, 56% based on Eu(NO3)3·6H2O). Analytical data (%), calcd: C, 39.35; H, 4.05; N, 12.52; found: C, 39.48; H, 4.08; N, 12.50; IR (KBr, υ, cm−1): 3394 (w), 2974 (w), 1602 (s), 1510 (s), 1474 (s), 1308 (m), 1242 (s), 1145 (m), 1012 (m), 816 (m), 741 (m), 584 (m).
2.4 X-ray crystallographic study
Suitable yellow block crystals of EuL (0.21 × 0.16 × 0.12 mm3) and EuL′ (0.16 × 0.12 × 0. 06 mm3) were coated with perfluoropolyether oil before mounting. Intensity data were recorded with a Bruker Smart Apex II CCD diffractometer with agraphite-monochromated Mo-Kα radiation (λ = 0.071073 Å) using the ω–2θ scan mode. No crystal decay were observed during the data collections. Absorption corrections based on multi-scan using the SADABS software14 were applied. The structure was solved by direct methods15 and refined on F2 by a full-matrix least-squares procedure based on all data minimizing wR = [Σ[w(Fo2 − Fc2)2]/Σ(Fo2)2]1/2, R = Σ||Fo| − |Fc||/Σ|Fo| and S = [Σ[w(Fo2 − Fc2)2]/(n − p)]1/2. SHELXL-2014 was used for both structure solutions and refinements.16 All non-hydrogen atoms were refined anisotropically. The positions of hydrogen atoms were calculated and isotropically fixed in the final refinement [d(C–H) = 0.95 Å, with the isotropic thermal parameter of Uiso(H) = 1.2 Uiso(C)]. The SMART and SAINT software packages17 were used for data collection and reduction respectively. Crystallographic diagrams were drawn using the DIAMOND software package.18 The structures were examined using the Add sym sub routine of PLATON to ensure that no additional symmetry could be applied to the models. Also severely disordered acetonitrile molecules in EuL and EuL′, were removed by SQUEEZE during the structural refinements.19 For details about the squeezed material, see cif data in ESI.† Therefore, three and one crystalline acetonitrile molecules which were determined on the basis of elemental microanalysis and the data treated with the SQUEEZE routine within PLATON were added to the molecular formula of EuL and EuL′ respectively. A summary of the relevant crystallographic data and the final refinement details are given in Table 1, important bond lengths are listed in Table 2.
Table 1 Crystal data and structure refinement parameters for EuL and EuL′
Empirical formula |
C48H42Eu2N12O24 |
C42H51Eu2N11O18 |
Crystal system, space group |
Trigonal, R![[3 with combining macron]](https://www.rsc.org/images/entities/char_0033_0304.gif) |
Monoclinic, C2/c |
Unit cell dimensions |
a = 17.4511(7) Å, α = 90° |
a = 28.3358(12) Å, α = 90° |
b = 17.4511(7) Å, β = 90° |
b = 20.1143(7) Å, β = 118.325(4)° |
c = 40.5949(16) Å, γ = 120° |
c = 20.98177(7) Å, γ = 120° |
Volume |
10 706.5(10) Å3 |
10 526.8(8) Å3 |
Z, calculated density |
6, 1.732 kg m−3 |
8, 1.643 kg m−3 |
Absorption coefficient |
1.795 mm−1 |
2.441 mm−1 |
F(000) |
4392 |
4530 |
Crystal size |
0.21 × 0.16 × 0.12 mm |
0.16 × 0.12 × 0.06 mm |
Theta range for data collect |
3.60 to 25.50° |
3.46 to 26.00° |
Limiting indices |
−21 ≤ h ≤ 11, −16 ≤ k ≤ 16, −49 ≤ l ≤ 30 |
−34 ≤ h ≤ 33, −24 ≤ k ≤ 18, −25 ≤ l ≤ 24 |
Reflections collected/unique |
7665/4442 [R(int) = 0.0358] |
21 725/10 272 [R(int) = 0.0452] |
Completeness to theta = 25.01 |
99.7% |
99.2% |
Data/restraints/parameters |
4442/45/269 |
10 272/79/672 |
Goodness-of-fit on F2 |
1.036 |
1.053 |
Final R indices [I > 2 sigma(I)] |
R1 = 0.0539, wR2 = 0.1675 |
R1 = 0.0514, wR2 = 0.1221 |
R indices (all data) |
R1 = 0.0772, wR2 = 0.1820 |
R1 = 0.0780, wR2 = 0.1388 |
Largest diff. peak and hole |
1.861 and −1.410 e Å−3 |
2.622 and −1.354 e Å−3 |
Table 2 Selected bond lengths (Å) for EuL and EuL′
[Eu2(H2L)3(NO3)3]·3CH3CN (EuL) |
Eu1–O1 2.311(5) Mn1–O1 1.846(2) |
Eu1–O7 2.498(6) |
Eu1–O6 2.558(5) |
Eu2–O3 2.401(5) |
Eu2–O1 2.480(4) |
Eu2–O2 2.510(5) |
|
|
|
|
![[thin space (1/6-em)]](https://www.rsc.org/images/entities/char_2009.gif) |
[Eu2(H2L′)2(NO3)4]·2CH3CN (EuL′) |
Eu1–O3 2.169(6) |
Eu1–O1 2.267(5) |
Eu1–O4 2.286(4) |
Eu1–O2 2.302(5) |
Eu1–O7 2.400(5) |
Eu1–O8 2.406(5) |
Eu1–O11 2.423(5) |
Eu1–O10 2.492(5) |
Eu2–O6 2.210(5) |
Eu2–O5 2.260(5) |
Eu2–O1 2.270(4) |
Eu2–O4 2.304(5) |
Eu2–O14 2.387(5) |
Eu2–O13 2.396(6) |
Eu2–O17 2.423(6) |
Eu2–O16 2.437(5) |
|
|
|
|
2.5 Sensing experiment
Luminescence spectra were recorded on a Hitachi F-7000 spectrophotometer. Spectroscopic measurements were recorded in acetonitrile solution. Stock solutions (0.01 M) of the nitrate salts of Li+, Na+, Mg2+, Ca2+, Ba2+, Mn2+, Fe2+, Fe3+, Co2+, Ni2+, Cu2+, Cd2+, Hg2+, Zn2+ and Al3+ in distilled water were prepared. Stock solutions of EuL (0.1 mM) were prepared in acetonitrile solution. Test solutions were prepared by placing 2 mL of the probe stock solution into a test tube and adding an appropriate aliquot of each ions stock. The mixture solution was stirred for 30 seconds, and waited about one minute. Complex formation was monitored following the absorption spectral changes. Both the excitation and emission slit widths were 5.0 and 5.0 nm, respectively.
3 Result and discussion
3.1 Crystal structure of EuL
Signal-crystal X-ray diffraction analysis revealed that EuL is a dinuclear complex with a ligand metal stoichiometric ratio of 3
:
2. As shown in Table 1, EuL crystallizes in the trigonal system space group R
, which allows a 3-fold rotational axis passes through EuIII ions and thus to give a C3 molecular symmetry. In the asymmetry unit of EuL, there are two crystallographically independent EuIII ions (Eu1 and Eu2) with one third occupancy, one partly deprotoned H2L− and one nitrate anions (Fig. 1a). Eu1 is coordinated by three phenoxy atoms (O1) of salicylamide group from three crystallgraphically equivalent H2L− ligands and six oxygen atoms (O6 and O7) from three crystallgraphically equivalent nitrate anions. Meanwhile, Eu2 is coordinated by three amide oxygen atoms (O2), three phenoxy oxygen atoms (O1) and three phenolic hydroxyl atoms (O3) from three crystallgraphically equivalent H2L− ligands. Exact geometry analysis by SHAPE 2.1 software shows that the inner coordination sphere of the two nine-coordinated EuIII ions are residing in two distorted tricapped trigonal prism sharing one bottom with a deviation of 13.096 for Eu1 and 12.686 for Eu2 from the ideal D3h symmetry (Fig. S1 and Table S1†). The Eu–O bond distances vary from 2.311(5) to 2.558(5) Å and the O–Eu–O bond angles fall in the range of 51.69(5)–158.72(9)°, which are exactly comparable to those reported in other EuIII compounds.20 As shown in Fig. 1b, the H2L− ligands in EuL adopt a chelating-bridging tetradentate μ4-η1:η1:η2 coordination mode, and as a result, Eu1 and Eu2 ions are linked by three phenoxy atoms (O1) engendering a [Eu2(μ-O)3] core with a Eu1⋯Eu2 separation of 3.576 (2) Å and a Eu1–O1–Eu2 angle of 96.46(6)°. Upon further investigation, we find there are hydrogen bonds between imine and phenolic hydroxyl groups with imine groups being hydrogen bonding acceptor and phenolic hydroxyl groups being hydrogen bonding donor. The existence of these intramolecular hydrogen bonds could prevent imine nitrogen atom from coordination. What deserves special note is the preorganized cavity existed in EuL, where the three phenolic hydroxyl groups on 2-(iminomethyl)phenol moiety are arranged in a radial manner anchored with Lewis base sites which can be very useful for binding suitable metal ions.
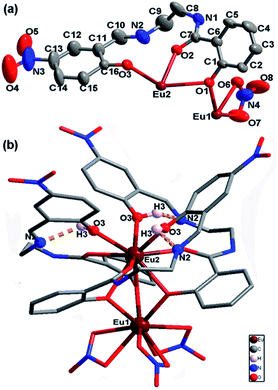 |
| Fig. 1 a) Asymmetric unit of EuL with thermal ellipsoids at 50% probability; (b) molecular structure of EuL showing intramolecular hydrogen bonds between imine and phenolic hydroxyl groups. | |
To confirm the role of different substitution groups in the self-assembly process, H3L′ was used instead of H3L to perform the reaction under the same experimental conditions. As expected, compound EuL′ crystallizes in the monoclinic C2/c space group with a 1
:
1 ligand metal stoichiometric ratio which is quite different from that of EuL due to the substitution of nitryl with diethylamino group. A view of the molecular structure with partly numbering scheme is depicted in Fig. 2a. Two crystallographically independent EuIII ions (Eu1 and Eu2), are doubly bridged by two phenoxy of salicylamide with the remaining coordinating sites fulfilled by carbonyl oxygen atom, phenolic hydroxy group from the same ligand H2L′−, and four oxygen atoms from two bidentate nitrate anions. Contrast to EuIII compound of H3L, two EuIII ions are eight-coordinated and their coordination geometries are distorted square antiprisms sharing one side with a deviation of 5.258 for Eu1 and 7.034 for Eu2 from the ideal D4d symmetry (Fig. S2 and Table S1†) based on exact geometry analysis by SHAPE 2.1 software. Similar to that in EuL, H2L′− also adopts tetradentate coordination modes with one phenolic hydroxy group and one amide oxygen atom chelating to one europium ion as well as one phenoxy atom bridging another europium ion. As a result, imine nitrogen atoms were also deprived of coordination due to the existence of molecular hydrogen bonds between imine and phenolic hydroxy groups (Fig. 2b). Notably, the distance of two phenolic hydroxyl groups on 2-(iminomethyl)phenol moiety in EuL′ is 3.847 Å. Clearly, the structural difference between EuL and EuL′ are owing to the electronic property of nitryl and diethylamino group in spite of their poor coordination abilities. Such differences in structure must have an impact on their luminescence sensing ability to metal ions.
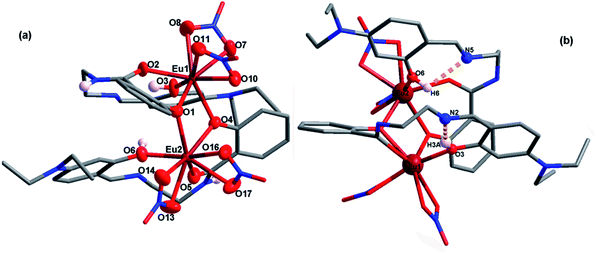 |
| Fig. 2 (a) A view of the molecular structure of EuL′ with partly numbering scheme; (b) molecular structure of EuL′ showing intramolecular hydrogen bonds between imine and phenolic hydroxyl group. | |
3.2. UV-vis absorption studies
The UV-vis titration of EuIII and Al3+ were conducted using a 10.0 μM solution of H3L/H3L′ + triethylamine in CH3CN. As shown in Fig. 3a and c, the absorption spectrum of H3L + triethylamine and H3L′ + triethylamine exhibit different absorption properties owing to the different electronic properties of nitryl and diethylamino group: the former shows three strong absorption bands centered at 208, 238 and 252 nm with several relative weak broad bands ranging from 314 to 404 nm which tail out to 450 nm, while the latter exhibits three relatively weak absorption bands centered at 215, 224 and 335. Upon addition of EuIII, there were clearly five isosbestic points at 237, 249, 276, 321 and 403 nm for H3L + triethylamine, and two isosbestic points at 275 and 348 nm for H3L′ + triethylamine, indicating the formation of the corresponding compound. Obviously, the absorbance of H3L + triethylamine shows a linear increase until the stoichiometric ratio of EuIII
:
L reaches 2
:
3, and no longer obvious changes can be observed with continuously titrated of EuIII, suggesting that the stoichiometry between the ligand and EuIII is 3
:
2. Meanwhile, the change observed in Fig. 3c shows a 1
:
1 ligand metal stoichiometric ratio. All these results are consistent with the structural analysis discussed above. Further incremental addition of Al3+ to the EuL in CH3CN, obvious changes in the absorption bands are also observed (Fig. 3b). The absorbance at 232 nm is almost unchanged with concomitant hypsochromic shift in the absorbance at 349 nm and simultaneous development of a new peak at 260 nm. After addition of 1 equiv. of Al3+, saturation point is reached. However, no such significant change in the absorption spectrum of EuL was observed with other tested metal cations. All these may indicate a formation of a complex between EuL and Al3+. By comparision, addition of Al3+ to the resulted EuL′ solution bring no spectra change (Fig. 3d), indicating there are no electronic interaction between Al3+ and EuL′.
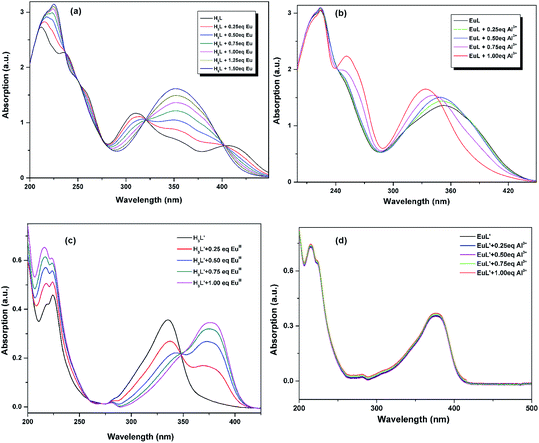 |
| Fig. 3 (a) UV-vis titration of H3L + triethylamine (10.0 μM) with the addition of increasing concentration of EuIII in CH3CN; (b) UV-vis titration of EuL (10.0 μM) upon the addition of increasing concentration of Al3+ in CH3CN; (c) UV-vis titration of H3L′ + triethylamine (10.0 μM) upon the addition of increasing concentration of EuIII in CH3CN; (d) UV-vis titration of EuL′ (10.0 μM) with the addition of increasing concentration of Al3+ in CH3CN. | |
3.3 Luminescence and sensing properties
As shown in Fig. 4, excitation of the absorption bands at 326 nm or 349 nm produce blue fluorescence positioned at 406 and 471 nm for H3L + NEt3, and broad emission band at λmax = 406 nm for H3L′ + NEt3. Upon coordination to EuIII ions, pink emission of EuL and EuL′ were observed by naked eyes following excitation with a standard UV lamp (λex = 365 nm). Excitation of the ligand-centered absorption band at 375 nm, emissions both originating from the ligand and EuIII ions were observed with the EuIII-centered emission (619 nm) intensity more than three times higher than that of ligand-centered emissions (432 nm) for EuL for EuL′, confirming that the energy transfers from ligands' chromophore to EuIII ions occur (Fig. 4). The EuIII-centered emissions shows five characteristic bands originated from the 5D0 state to the 7FJ (J = 0, 1, 2, 3, 4) at about 581, 594, 619, 653 and 698 nm. The first transition (5D0 → 7F0) observed at the wavelength 581 nm is symmetric, indicating only one type of europium were present in all complexes studied.21 The second transition (5D0 → 7F1) with magnetic dipole nature observed at the wavelength 594 nm. The most intensive maxima correspond to the 5D0 → 7F2 transition for EuL and EuL′ are found at wavelength 619 nm. The ratios of intensities corresponding to 5D0 → 7F2 and 5D0 → 7F1 transitions are 5.8 and 2.4 for EuL and EuL′ respectively, showing a strong deviation from central symmetry of EuIII coordination geometry in EuL compared to that in EuL′. The 5D0 → 7F3 transition observed at 653 nm is very weak because it is forbidden. It is well-known that the presence of OH oscillators in the lanthanide first coordination sphere provides an efficient non-radiative path.22 Therefore, owing to the combined effects of excited state intramolecular proton transfer (ESIPT) from phenolic –OH to imine-N and the presence of OH oscillators in the lanthanide first coordination sphere as ascertained by structural analysis, the luminescence of EuL and EuL′ are weak.
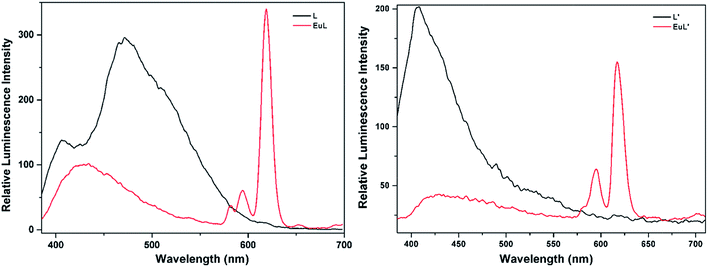 |
| Fig. 4 Emission spectra of H3L/H3L′ (black solid line) and EuL/EuL′ (red solid line) in CH3CN. | |
The selectivity of EuL and EuL′ towards various common metal ions was examined by addition of 1.0 quiv. aqueous solution containing different metal ions such as Li+, Na+, Ca2+, Mg2+, Al3+, Co2+, Fe2+, Ni2+, Fe3+, Mn2+, Cu2+ Zn2+, Cd2+, and Hg2+ to the solution of EuL and EuL′ in CH3CN and tested under the same conditions. As depicted in Fig. 5, upon Al3+ addition, 5.7 times significant luminescence enhancement was observed for EuL. While for EuL′, a 6.2 times enhancement was caused by Li+ addition. We can also see from Fig. 5, that transition metal cations with unpaired d-electrons have a little varying degrees quenching effect on the luminescence intensity of EuL and EuL′ as compared to other transition metal with d10 electron configuration, alkaline metal ions and alkaline earth metal ions. Furthermore, the considerably ‘turn-on’ luminescence response of EuL led to the apparent color change from pink to brilliant red which could also be identified by naked eyes easily under UV lamp (Fig. 6).
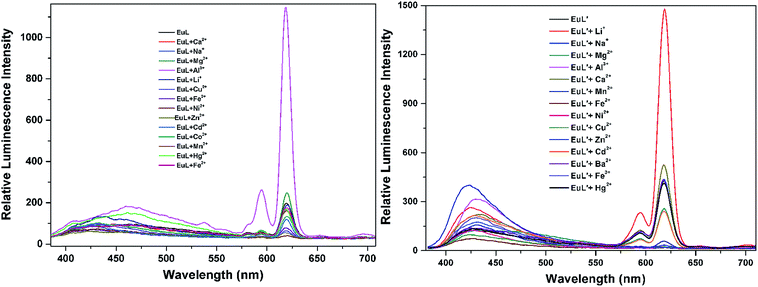 |
| Fig. 5 Comparison of the luminescence intensity of EuL and EuL′ (0.1 mM) upon addition of various metal ions in CH3CN. | |
 |
| Fig. 6 The visual fluorescence response of EuL (0.1 mM) upon addition of metal ions (0.5 mM) in CH3CN under UV lamp at 365 nm. | |
To explore the effective applications of EuL and EuL′, the luminescence response of EuL towards Al3+ and EuL′ towards Li+ in presence of typical competing ions were studied and the results were shown in Fig. 7. Data in Fig. 7 shows that there is little interference of EuL for detection of Al3+ in presence of Li+, Na+, Mg2+, Mn2+, Fe3+, Ni2+, Fe2+, Cu2+, Zn2+, Cd2+, Co2+ as follows. In the case of Cu2+, quenching of the fluorescence signal was observed. The response of EuL for Al3+ detection in the presence of Mn2+, Ni2+, Co2+, Zn2+, Cd2+, and Hg2+ is relatively low but clearly detectable. Thus, EuL can be used as a selective luminescent chemosensor for Al3+ detection in presence of most competing metal ions. Comparably, very significant interference exists in EuL′ for detecting Li+ in presence of Mg2+, Mn2+, Fe3+, Ni2+, Fe2+, Cu2+, Zn2+, Cd2+, Co2+, Ca2+ and Hg2+. These results clearly indicated that EuL rather than EuL′ could be used as a potential metal-selective luminescent sensor even in presence of a large excess of other competitive metal ions in acetonitrile.
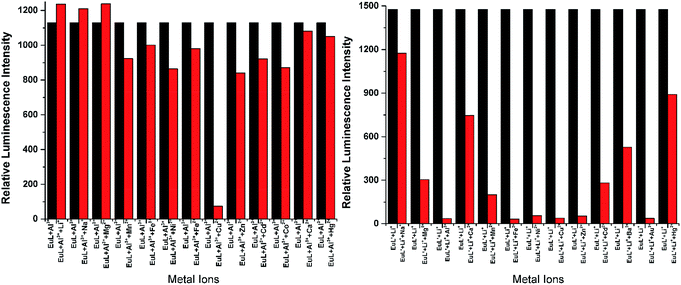 |
| Fig. 7 Bar chart illustrating luminescence response of EuL + Al3+ and EuL′ + Li+ at 619 nm towards different cations in CH3CN (λex = 375 nm). Conditions: EuL = 0.1 mM, EuL′ = 0.1 mM, Al3+ = 0.1 mM, Mn+ = 0.01 M; where, Mn+ = Li+, Na+, Mg2+, Mn2+, Fe3+, Ni2+, Fe2+, Zn2+, Cd2+, Co2+, Cu2+, Ca2+ and Hg2+. | |
Further determination of sensitivity was carried out by gradually adding Al3+ ion aqueous solution to EuL in acetonitrile solution. As shown in Fig. 8, the sequential enhancing of emission intensity at 619 nm of EuL was recorded as a result of gradual increased concentration of Al3+ aqueous solution. The emission intensity at 619 nm increases gradually with the increase in concentration of Al3+ up to a mole ratio EuL
:
Al3+ = 1
:
1 and then becomes saturated. A luminescence enhancement of 5.7-fold at 619 nm with the quantum yield increasing from Φ = 4.5% to Φ = 16.9% can be realized upon addition of 1.0 equiv. Al3+. The lifetimes of EuL and its complex with Al3+ in acetonitrile were also measured. The lifetime of EuL is quite short (0.16 ms). Upon addition of 1.0 equiv. Al3+, the lifetime of EuL increased to 0.94 ms. Unfortunatly, no crystalline product was obtained upon Al3+ addition.
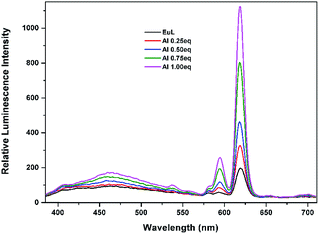 |
| Fig. 8 Luminescence titration spectra of EuL (0.1 mM) upon gradual addition of Al3+ (0.1 mM) in CH3CN (λex = 375 nm). | |
Job's method was further employed to determine the composition of the complex, the luminescence emission intensity of EuIII for complexation between EuL and Al3+ has a maximum at a mole fraction of 0.5, thus indicative of the formation of a 1
:
1 complex.23 (Fig. S3†). As shown in Fig. 9, Benesi–Hiderband plot of [Imax − I0)/(I − I0)] vs. 1/[Al3+] gives straight line with slope Kd = 1.53 × 10−4 M with R2 = 0.99, indicating a 1
:
1 moderately strong binding of EuL towards Al3+. This conclusion has also been certified by electrospray ionization mass spectrum (ESI-MS). The positive ion mass spectrum of EuL upon addition of 1.0 equiv. of Al3+ exhibited intense peaks at m/z = 1473.76 and m/z = 1642.09, which can be assigned to the ion of [EuL − H]+, [EuL − 2H + Al + 3H2O]+ respectively (Fig. S4†). The LOD of Al3+ calculated by using 3σ method was found to be 0.33 μM. (Fig. S5†) which is lower than the maximum tolerable limit of Al3+. Such a low detection limit as well as the selective emission enhancement reach to a conclusion that EuL is an effective sensor for selective detection of Al3+ in acetonitrile.
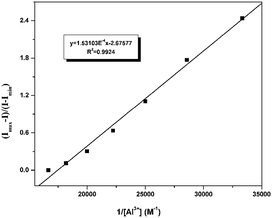 |
| Fig. 9 Benesi–Hiderband plot of EuL toward Al3+. | |
To explore the mechanism of the luminescence turn-on behavior for Al3+ ions, we performed HCl titrations where the possibility of the formation of EuL·Al is expected to reduce. For this purpose, 20 μL of 2 M HCl solution was added sequentially to the acetonitrile solution of EuL after addition of 1.0 equiv. Al3+ ions. As shown in Fig. 10, the luminescence intensity of EuIII decreased a lot upon gradual addition of HCl, which may be due to the dissociation of EuL·Al as the result of the protonation of phenolic hydroxy group. This strongly supports the state that the interaction between Al3+ and EuL is through the formation of EuL·Al at the expense of destroying intramolecular hydrogen bonding between imine and phenolic hydroxy groups. Therefore, the enhancement of the luminescent intensity of EuL upon Al3+ incorporation is presumably due to metal binding to the cavity formed by three phenolic hydroxyl group in EuL followed by excited-state intra-molecular proton transfer (ESIPT) and photo induced electron transfer suppression.
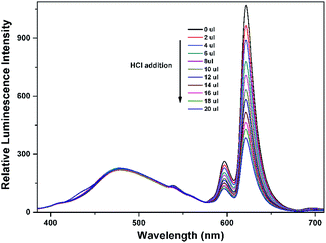 |
| Fig. 10 Concentration-dependent luminescence quenching of compound EuL·Al (0.1 mM) after adding different amounts of 2 M HCl at room temperature. | |
According to the analysis of the structural features, we found that in the molecular structure of EuL, three phenolic hydroxyl groups provide a cavity with hard Lewis base chelating sites together with the geometric uniqueness of the cavity leads to a high affinity for Al3+ (Scheme 2). Among common metal ions examined herein, charge-to-size ratio of Al3+ ions is so high that it is expected to destroy the triple intramolecular N⋯H–O hydrogen bonds in EuL to form EuL·Al, where the three phenolic hydroxy groups of EuL act as simple electron donor to Al3+ ions through Lewis acid-base type interactions. Based on the analysis above, Al3+ binds to EuL through three hydroxy groups and the hexacoordination of Al3+ can be satisfied by three water molecules. Obviously, nitryl groups with strong electron withdrawing effect in EuL plays a key role in constructing this distinctive architecture and the consequent detection of Al3+ ion.
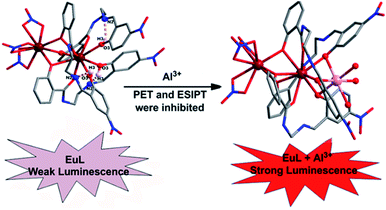 |
| Scheme 2 Sensing mechanism of EuL towards Al3+. | |
To further understand the relationship between the structure of EuL and the respective optical response to Al3+, we carried out density function theory (DFT) calculations with the B3LYP/6-31 G(d) basis set using the Gaussian 09 program. As displayed in Fig. 11, the HOMO of EuL is distributed on salicylamide moiety of three H2L− ligands, meanwhile, the LUMO of EuL is localized on 2-(iminomethyl)phenol moiety, indicating the presence of PET process from salicylamide group of the molecule to the 2-(iminomethyl)-5-nitrophenol moiety. The optimized structure of EuL·Al complex shows that the Al3+ ion binds to EuL at phenolic oxygen atoms of 2-(iminomethyl)-5-nitrophenol moiety, with three water molecules to satisfy the need of saturated coordination. In the EuL·Al complex, the electron density of HOMO is also localized on salicylamide moieties, while the electron density of LUMO is localized on the 2-(iminomethyl)-5-nitrophenol moiety. For the electron density as well as energy gap of EuL·Al compared with that of EuL only changed slightly, which excludes the possibility that the increasing of EuIII luminescence is due to a change of the nature of the antenna triplet state. Therefore we can suggest that the observed increase in luminescence intensity, lifetime and quantum yield of the 5D0 level of EuIII is mainly related to the decrease in non-radiative transitions due to the absent of OH oscillators in the EuIII first coordination shell upon Al3+ coordination followed by excited-state intra-molecular proton transfer (ESIPT) and photo induced electron transfer suppression.
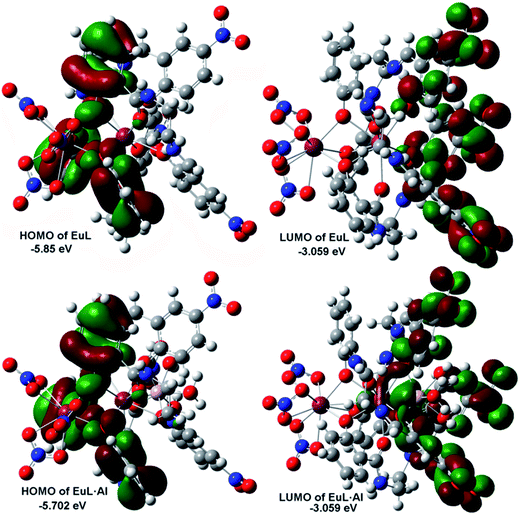 |
| Fig. 11 HOMO–LUMO energy levels and the molecular orbital plots of EuL and EuL·Al. | |
4 Conclusions
In summary, a novel luminescence-enhanced EuIII complex-supported chemosensor EuL was developed by tuning the substitution group on 2-(iminomethyl)phenol moiety of salicylamide salen-like ligands. The strong electron withdrawing capability of nitryl group led to the formation of the phenoxy bridged dinuclear EuIII complex anchored with Lewis base sites which is distinctive for binding Al3+ ions. Its sensing ability for a wide range of metal ions (Li+, Na+, Mg2+, Ca2+, Mn2+, Fe2+, Fe3+, Co2+, Ni2+, Cu2+, Zn2+, Cd2+, Hg2+ and Al3+) was tested and the results indicated that EuL exhibited high selectivity and sensitivity for Al3+ in the presence of various metal cations. Job's plot and mole–ratio curves revealed a 1
:
1 stoichiometry between EuL and Al3+. To the best of our knowledge, this is the first example of EuIII based luminescent sensor for the detection of Al3+ that demonstrated significant luminescence enhancement. The detection limit was sufficiently low to determine the micromolar levels of Al3+and EuL could be served as an excellent lanthanide chemsensor for highly toxical aluminum ion. The sensing mechanism can be explained in terms of the decrease of non-radiative transitions in EuL in addition to excited-state intra-molecular proton transfer (ESIPT) and photo-induced electron transfer suppression upon Al3+ coordination which is also rationalized by a theoretical calculation. However, EuL is not ideal luminescent sensors for it can't be used in pure aqueous solution.
Acknowledgements
This work was supported by the National Natural Science Foundation of China (Grant 21661019).
Notes and references
-
(a) M. Sy, A. Nonat, N. Hildebrandt and L. J. Charbonniére, Chem. Commun., 2016, 52, 5080 RSC;
(b) A. Foucault-Collet, C. M. Shade, I. Nazarenko, S. Petoud and S. V. Eliseeva, Angew. Chem., 2014, 53, 2927 CrossRef CAS PubMed;
(c) A. J. Palmer, S. H. Ford, S. J. Butler, T. J. Hawkins, P. J. Hussey, R. Pal, J. W. Walton and D. Parker, RSC Adv., 2014, 4, 9356 RSC;
(d) A. J. Amoroso and S. J. A. Pope, Chem. Soc. Rev., 2015, 44, 4723 RSC.
-
(a) Y. Suffren, D. Zare, S. V. Eliseeva, L. Guenee, H. Nozary, T. Lathion, L. Aboshyan-Sorgho, S. Petoud, A. Hauser and C. Piguet, J. Phys. Chem. C, 2013, 117, 26957 CrossRef CAS;
(b) S. F. H. Correia, V. de Z. Bermudez, S. J. L. Ribeiro, P. S. André, R. A. S. Ferreira and L. D. Carlos, J. Mater. Chem. A, 2014, 2, 5580 RSC;
(c) J.-C. G. Bunzli and S. V. Eliseeva, Chem. Sci., 2013, 4, 1939 RSC.
-
(a) H. Suzuki, J. Photochem. Photobiol., A, 2004, 166, 155 CrossRef CAS;
(b) Y. Hasegawa and T. Nakanishi, RSC Adv., 2015, 5, 338 RSC;
(c) S. Z. Zou, Q. P. Li and S. W. Du, RSC Adv., 2015, 5, 34936 RSC;
(d) H. B. Wei, G. Yu, Z. F. Zhao, Z. W. Liu, Z. Q. Bian and C. H. Huang, Dalton Trans., 2013, 42, 8951 RSC.
- P. Montgomery, B. S. Murray, E. J. New, R. Pal and D. Parker, Acc. Chem. Res., 2009, 42, 925 CrossRef PubMed.
- J. C. G. Bünzli, Chem. Rev., 2010, 110, 2729 CrossRef PubMed.
-
(a) J. Barcelo and C. Poschenrieder, Environ. Exp. Bot., 2002, 48, 75 CrossRef CAS;
(b) B. Valeur and I. Leray, Chem. Rev., 2000, 205, 3 CAS;
(c) Z. Krejpcio and R. W. P. J. Wojciak, Environ. Stud., 2002, 11, 251 CAS;
(d) P. Nayak, Environ. Res., 2002, 89, 111 CrossRef.
-
(a) S. Malkondu, Tetrahedron, 2014, 70, 5580 CrossRef CAS;
(b) A. S. M. Islam, R. Bhowmick, H. Mohammad, A. Katarkar, K. Chaudhuri and M. Ali, New J. Chem., 2016, 40, 4710 RSC;
(c) D. Maity and T. Govindaraju, Inorg. Chem., 2010, 49, 7229 CrossRef CAS PubMed;
(d) H. Xu, M. Fang, C. S. Cao, W. Z. Qiao and B. Zhao, Inorg. Chem., 2016, 55, 4790 CrossRef CAS PubMed;
(e) L. Y. Wang, H. H. Li and D. R. Cao, Sens. Actuators, B, 2013, 181, 749 CrossRef CAS;
(f) S. H. Kim, H. S. Choi, J. Kim, S. J. Lee, D. T. Quang and J. S. Kim, Org. Lett., 2010, 12, 560 CrossRef CAS PubMed;
(g) Y. W. Wang, M.-X. Yu, Y.-H. Yu, Z.-P. Bai, Z. Shen, F.-Y. Li and X.-Z. You, Tetrahedron Lett., 2009, 50, 6169 CrossRef CAS;
(h) D. Maity and T. Govindaraju, Chem. Commun., 2010, 46, 4499 RSC;
(i) T. H. Ma, M. Dong, Y. M. Dong, Y. W. Wang and Y. Peng, Chem.–Eur. J., 2010, 16, 10313 CrossRef CAS PubMed;
(j) Y. S. Kim, G. J. Park, J. J. Lee, S. Y. Lee, S. Y. Lee and C. Kim, RSC Adv., 2015, 5, 11229 RSC.
- K. Hanaoka, K. Kikuchi, H. Kojima, Y. Urano and T. Nagano, J. Am. Chem. Soc., 2004, 126, 12470 CrossRef CAS PubMed.
- W. S. Liu, T. Q. Jiao, Y. Z. Li, Q. Z. Liu, M. Y. Tan, H. Wang and L. F. Wang, J. Am. Chem. Soc., 2004, 126, 2280 CrossRef CAS PubMed.
- A. Thibon and V. C. Pierre, J. Am. Chem. Soc., 2009, 131, 434 CrossRef CAS PubMed.
-
(a) N. Shao, J. Y. Jin, G. L. Wang, Y. Zhang, R. H. Yang and J. L. Yuan, Chem. Commun., 2008, 9, 1127 RSC;
(b) J. W. Wang, J. Wu, Y. M. Chen, H. P. Wang, Y. R. Li, W. S. Liu, H. Tian, T. Zhang, J. Xu and Y. Tang, Dalton Trans., 2012, 41, 12936 RSC;
(c) C. G. Gulgas and T. M. Reineke, Inorg. Chem., 2008, 47, 1548 CrossRef CAS PubMed;
(d) M. S. Tremblay, M. Lee and D. Sames, Org. Lett., 2008, 10, 5 CrossRef CAS PubMed;
(e) H. Akiba, J. Sumaoka, K. Tsumoto and M. Komiyama, Anal. Chem., 2015, 87, 3834 CrossRef CAS PubMed;
(f) F. Piccinelli, M. Leonzio, M. Bettinelli, M. Monari, C. Grazioli, A. Melchior and M. Tolazzi, Dalton Trans., 2016, 45, 3310 RSC.
-
(a) X. Q. Song, G. Q. Cheng, X. R. Wang, W. Y. Xu and P. P. Liu, Inorg. Chim. Acta, 2015, 425, 145 CrossRef CAS;
(b) X. Q. Song, G. Q. Cheng and Y. A. Liu, Inorg. Chim. Acta, 2016, 450, 386 CrossRef CAS;
(c) X. Q. Song, Y. K. Lei, X. R. Wang, M. M. Zhao, Y. Q. Peng and G. Q. Cheng, J. Solid State Chem., 2014, 208, 212 Search PubMed.
-
(a) P. P. Liu, L. Sheng, X. Q. Song, W. Y. Xu and Y. A. Liu, Inorg. Chim. Acta, 2015, 434, 252 CrossRef CAS;
(b) X. Q. Song, P. P. Liu, Z. R. Zhou, X. Li and Y. A. Liu, Inorg. Chim. Acta, 2015, 438, 232 CrossRef CAS;
(c) X. Q. Song, P. P. Liu, Y. A. Liu, J. J. Zhou and X. L. Wang, Dalton Trans., 2016, 45, 8154 RSC.
- SADABS, version 2.03, Bruker AXS Inc, Madison, WI, 2002 Search PubMed.
- G. M. Sheldrick, Acta Crystallogr., Sect. A: Found. Crystallogr., 1990, 46, 467 CrossRef.
- G. M. Sheldrick, SHELXL-2014, University of Gottingen, Gottingen, Germany, 2014 Search PubMed.
- SAINT, version 6.02, Bruker AXS Inc, Madison, WI, 2002 Search PubMed.
- DIAMOND, Visual Crystal Structure Information System, version 3.1, Crystal Impact, Bonn, Germany, 2004 Search PubMed.
- A. L. Spek, The calculation of the solvent-accessible was performed by using the PLATON software (similarly herein after), J. Appl. Crystallogr., 2003, 36, 7 CrossRef CAS.
-
(a) K. Norton, T. J. Emge and J. G. Brennan, Inorg. Chem., 2007, 46, 4060 CrossRef CAS PubMed;
(b) X. Q. Song, L. Wang, M. M. Zhao, G. Q. Cheng, X. R. Wang and Y. Q. Peng, Inorg. Chim. Acta, 2013, 408, 71 CrossRef CAS.
- N. E. Borisova, A. V. Kharcheva, S. V. Patsaeva, L. A. Korotkov, S. Bakaev, M. D. Reshetova, K. A. Lyssenko, E. V. Belova and B. F. Myasoedov, Dalton Trans., 2017, 46, 2238 RSC.
- W. D. Horrocks Jr, J. Am. Chem. Soc., 1979, 101, 334 CrossRef.
-
(a) M. Lan, J. Wu, W. Liu, H. Zhang, W. Zhang, X. Zhuang and P. Wang, Sens. Actuators, B, 2011, 156, 332 CrossRef CAS;
(b) L. Fan, X. H. Jiang, B. D. Wang and Z. Y. Yang, Sens. Actuators, B, 2014, 205, 249 CrossRef CAS.
Footnote |
† Electronic supplementary information (ESI) available: A brief statement in CIF files, coordination polyhedron of EuL and EuL′ (Fig. S1 and S2), Job's plot between EuL and Al3+ (Fig. S3), ESI-MS spectra of EuL·Al (Fig. S4) and LOD curves (Fig. S5). CCDC 1534744 and 1534745. For ESI and crystallographic data in CIF or other electronic format see DOI: 10.1039/c7ra02386c |
|
This journal is © The Royal Society of Chemistry 2017 |