DOI:
10.1039/C7RA02242E
(Paper)
RSC Adv., 2017,
7, 23083-23092
Multi-wavelength excited white-emitting K2Gd(1−x)(PO4)(WO4):xDy3+ phosphors with satisfactory thermal properties for UV-LEDs
Received
23rd February 2017
, Accepted 13th April 2017
First published on 26th April 2017
Abstract
A series of novel phosphate–tungstate phosphors, K2Gd(1−x)(PO4)(WO4):xDy3+ (0 ≤ x ≤ 0.07), were synthesized successfully via a conventional solid-state reaction method for the first time. XRD, GSAS Rietveld refinement, FT-IR, Raman, diffuse reflectance spectra, luminescent spectra, and thermal quenching were applied to investigate the phase, structure, luminescence and thermal stability properties. The results showed that the Dy3+-activated K2Gd(PO4)(WO4) phosphor could absorb wavelengths from the UV to the blue region, and emit blue (∼480 nm), yellow (∼570 nm) and red (∼650 nm) light from the f–f transitions of Dy3+ ions. The dominant energy transfer mechanism for Dy3+ ions was dipole–dipole interaction. An analysis of the thermal quenching indicated that the obtained phosphors have excellent thermal stability. As the temperature increased to 423 K (150 °C), the emission intensity of the KGPW:0.05Dy3+ phosphor with 388 nm excitation still retained about 76.8% of its initial intensity at room temperature. In addition, the color purity of K2Gd0.995(PO4)(WO4):0.005Dy3+ was calculated to be 90% with a correlated color temperature of 5569 K. Therefore, these results suggest that multi-wavelength excited K2Gd(1−x)(PO4)(WO4):xDy3+ phosphors may have potential to be candidates for white LEDs for lighting and optical display applications.
1. Introduction
During the past few decades, solid state white light-emitting diodes (w-LEDs) have been drawing worldwide attention as next generation lighting devices due to their extraordinary advantages, such as high electro-optical conversion efficiency, high brightness, low power consumption and environmental benefits.1–5 Up until now, the most common strategy of w-LEDs is the combination of a blue InGaN chip (450–470 nm) and yellow emitting Y3Al5O12:Ce3+ (YAG:Ce3+) phosphor.6 However, this strategy couldn't meet the general illumination requirements long-term due to the low color rendering index (CRI) and the high correlated color temperature (CCT), which restrict its further application.4 This problem may be solved by the combination of a near-UV LED chip with tri-color (red, green and blue) phosphors.7,8 Nevertheless, in this tri-color phosphor system, the luminescence efficiency is relatively low since the blue emission is strongly reabsorbed by the green and red phosphors.9 Besides, the device of multiple emitting components in this system is very complicated and expensive, and it is also difficult to control the color balance. Compared with these methods, doping a single rare earth ion into an appropriate host is still the simplest and most straightforward method to obtain white light.
As we know, among rare earth ions, trivalent dysprosium (Dy3+) ion, which has a 4f9 electron configuration, can achieve most easily white light emission since the emission properties usually consist of three characteristic emission bands: one in the blue region (∼480 nm), one in the yellow region (∼580 nm), and last one in the red region (∼650 nm), originating from 4F9/2 → 6H15/2, 4F9/2 → 6H13/2, and 4F9/2 → 6H11/2 transitions, respectively. Therefore, by suitably tuning the yellow-to-blue (Y/B) intensity ratio, Dy3+ ions have significant potentialities to obtain white light emission. Owing to the prominence of white light emittance from Dy3+ ions, many researchers have spent a large amount of manpower and materials to explore the luminescence properties of Dy3+ ions in various hosts. Recently, we have manufactured some novel and promising Dy3+-activated single phase white-emitting phosphors matched with near-UV chips possessing potential application prospects in display and lighting.10–12
To date, a novel phosphate–tungstate family compound, M2Ln(PO4)(WO4) (M = Na, K; Ln = Y, Gd, Lu), has been used as host materials due to its easy synthesis, low sintering temperature, good thermal stability and high quenching concentration.1,13 In K2Ln(PO4)(WO4) crystal structure, the [Ln3+–O2−–Ln3+] unit is isolated by the surrounding [PO4]3− and [WO4]2− units in the three-dimensional network. This special lattice arrangement reveals that the interaction between the Ln3+–Ln3+ layers might be blocked, which cause the high quenching concentration phenomenon. For K2Ln(PO4)(WO4):Tb3+,Eu3+ (Ln = Y, Gd and Lu), the critical concentration of Tb3+ or Eu3+ is as high as 40–50% in the singly doped phosphor.1 However, in K2Y(WO4)(PO4):Tm3+,Dy3+, the critical concentration of Tm3+ or Dy3+ is 1% and 5%, respectively.14 Such a large quenching difference in the same type of structure is not only the problem of the host but also the rare earth ions. Additionally, there are one independent K+ site, one independent P5+ site, one independent W6+ site and one independent Ln3+ site in K2Ln(PO4)(WO4) unit cell. Considering the valence states and ionic radius of Ln3+ and Dy3+ ions, it is deduced that the Dy3+ ions easy to occupy Ln3+ sites.14
To the best of our knowledge, the studies on the luminescent properties of Dy3+-activated K2Gd(PO4)(WO4) phosphor are sparse.1,14 In this work, the structural, detailed luminescent properties, and thermal stabilities were applied to characterize the obtained phosphors. The emission color were located in the warm white-emitting region under near-UV excitation. In addition, compared with some other inorganic materials, the obtained phosphors exhibit a more excellent thermal stability. It is noteworthy that co-doped phosphor system severely affected by part of the energy loss due to the role of energy transfer, which is common in co-doped system. However, single-doped phosphor system can suppress the above situation, meanwhile it may have potential use in w-LEDs or some other areas.
2. Experimental
2.1 Materials and synthesis
The powder samples of the phosphate-tungstate K2Gd(1−x)(PO4)(WO4):xDy3+ (x = 0, 0.005, 0.01, 0.02, 0.03, 0.05 and 0.07) compound were prepared by solid state synthesis as follows. In the synthesis, all raw materials K2CO3 (99.90%), Gd2O3 (99.99%), (NH4)2HPO4 (99.90%), WO3 (99.99%) and Dy2O3 (99.99%) were used as received and weighed following the stoichiometric ratio. Firstly, the mixture were ground together about 30 min in an agate mortar. Secondly, the mixture based precursors were slowly pre-sintered at 500 °C (in air) and kept this temperature for 3 h to remove the chemically combined water and ammonium gas, as well as decompose the carbonate. After that, the samples were re-sintered at 950 °C (in air) for 3 h. At last, the obtained samples were ground well into powder for further characterization. The Dy3+-activated K2Gd(PO4)(WO4) phosphor was supplied by the display and Lighting Phosphor Bank at Pukyong National University.
2.2 Material characterization
The phase purity was carefully verified by using powder X-ray diffraction (XRD) measurement collected on a D8 Advanced diffractometer operated at 40 kV, 30 mA with Ni-filtered Cu-Kα irradiation (λ = 1.5406 Å). The powder diffraction patterns of K2Gd0.995(PO4)(WO4):0.005Dy3+ for Rietveld analysis was collected with a PANalytical X'Pert3 Powder instrument, the step size of 2θ was 0.013° over the angular range 10° ≤ 2θ ≤ 80°. Crystal structure refinement employed the Rietveld method as implemented in the General Structure Analysis System (GSAS) software suite.15 Transmission electron microscopy observations (TEM) were performed on JEM-2010 electron microscope with an accelerating voltage of 200 kV. The diffuse reflectance spectra (DRS) were recorded on a V-670 UV-vis spectrophotometer (JASCO Corp., Japan) ranging from 200 to 700 nm. The Fourier transform infrared spectroscopy (FT-IR) spectra were collected on a FT-IR spectrophotometer (FT/IR-4000, JASCO, Japan) over a frequency range 650–4000 cm−1. The Raman spectrometry measurements were carried out with a 532 nm laser JASCO NRS-5100 instrument. The photoluminescence excitation (PLE) and emission (PL) spectra were measured by using a Photon Technology International (PTI, USA) fluorimeter equipped with a 60 W xenon lamp excitation source. The fluorescence lifetime curves were measured with a phosphorimeter attached to the fluorescence spectrophotometer with a 25 W xenon flash lamp. The PL quantum efficiency (QE) was measured by using a spectrofluorometer (JASCO, FP-8500, Japan) equipped with an integrating sphere attachment (ISF-834). The color coordinates in Commission Internationale de l'Eclairage (CIE) 1931 chromaticity diagram were calculated from emission spectra by the color calculator software. The temperature-dependent luminescence properties were measured on a fluorescence spectrophotometer (SCINCO FS-2) with a heating apparatus (NOVA ST540).
3. Results and discussion
3.1 Phase formation and structural characteristics of K2Gd(PO4)(WO4)
The XRD patterns of K2Gd(1−x)(PO4)(WO4):xDy3+ (abbreviated as KGPW:xDy3+) phosphors, which the standard PDF file is not available, with different contents of Dy3+ ions are displayed in Fig. 1(a). By comparing all the diffraction peaks, no more impurities or raw materials were detected, indicating that those solid solutions are of phase purity and the single-doped Dy3+ ions do not cause any detectable change in KGPW host structure. Fig. 1(b) shows the observed and calculated XRD patterns of the GSAS Rietveld refinement for KGPW:0.005Dy3+ sample. The initial structural model for the refinement was established by the standard crystallographic data of K2Ho(PO4)(WO4) (ICSD#206654) compound which has an orthorhombic structure with space group Iabc.16 All the atom positions, fraction factors, thermal vibrational parameters, and background were converged and refined. The crystallographic data is presented in Table 1 and the refined atomic coordinate parameters are presented in Table 2. It can be observed that the final refined R factors converged to Rwp = 9.38%, RP = 6.35%, indicating that the solid solution KGPW:0.005Dy3+ shares the same structure with K2Ho(PO4)(WO4). In addition, the lattice parameters converged to a = 6.8794(2) Å, b = 12.1534(9) Å, c = 19.6921(4) Å, and V = 1646.442 Å3. Fig. 2 shows the elemental mapping of KGPW:0.03Dy3+ phosphor, revealing that the elements of K, Gd, P, W, O and Dy are uniformly distributed over the whole particle.
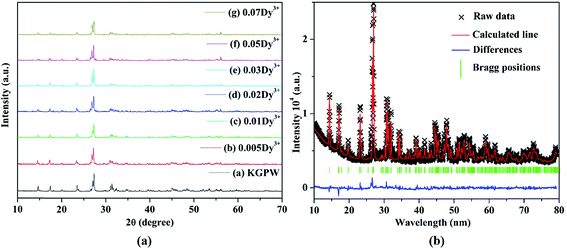 |
| Fig. 1 (a) XRD patterns of KGPW phosphors with different contents of Dy3+ ions. (b) Observed (crosses) and calculated (solid lines) XRD patterns of the Rietveld refinement for KGPW:0.005Dy3+ sample. | |
Table 1 The crystallographic data and selected bond lengths of KGPW:0.005Dy3+ sample
Crystallographic data of KGPW:0.005Dy3+ |
Selected bond length (Å) |
X-ray source |
CuKα |
Ln–O(1, 1′) |
2.3819(25) |
K–O(2) |
2.9844(6) |
T/K |
295 |
Ln–O(3, 3′) |
2.3850(8) |
K–O(2′) |
3.1908(6) |
Symmetry |
Orthorhombic |
Ln–O(4, 4′) |
2.3123(11) |
K–O(2′′) |
2.7177(6) |
Space group |
Ibca |
Ln–O(4′′, 4′′′) |
2.5290(8) |
K–O(3) |
2.7225(4) |
a/Å |
6.8794(2) |
P–O(3, 3′) |
1.54447(28) |
K–O(3′) |
2.7887(4) |
b/Å |
12.1534(9) |
P–O(4′′, 4′′′) |
1.58154(27) |
K–O(4′′) |
3.0945(7) |
c/Å3 |
19.6921(4) |
W–O(1, 1′) |
1.8400(4) |
Ln–Ln |
4.0330(6) |
Volume/Å3 |
1646.442 |
W–O(2, 2′) |
1.7540(4) |
|
|
Rp |
6.35% |
Ln–P1 |
3.2160(9) |
|
|
Rwp |
9.38% |
Ln–P2 |
2.9710(9) |
|
|
χ2 |
4.24% |
Ln–K |
3.8427(28) |
|
|
Table 2 Refined atomic coordinate parameters for KGPW:0.005Dy3+ sample as determined by Rietveld refinement of the powder XRD data at room temperature
Atom |
Wyck |
x/a |
y/b |
z/c |
W1 |
8e |
0.5000(0) |
0.2500(0) |
0.3325(4) |
Ln1 |
8d |
0.7500(0) |
0.3303(7) |
0.5000(0) |
K1 |
16f |
0.9687(2) |
0.0799(2) |
0.3438(9) |
P1 |
8d |
0.7500(0) |
0.0704(2) |
0.5000(0) |
O1 |
16f |
0.7088(0) |
0.2796(0) |
0.3853(6) |
O2 |
16f |
0.4420(0) |
0.3643(0) |
0.2845(0) |
O3 |
16f |
0.7308(0) |
−0.0047(0) |
0.4383(4) |
O4 |
16f |
0.9229(0) |
0.1514(0) |
0.4921(5) |
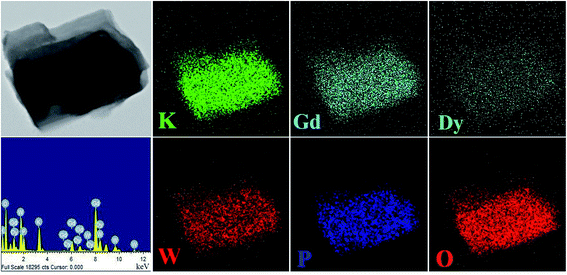 |
| Fig. 2 Elemental mapping of the KGPW:0.03Dy3+ phosphor. | |
Fig. 3 shows the crystal structure of KGPW:0.005Dy3+ obtained from GSAS refinement file. In KGPW:0.005Dy3+, two successive [WO4]2− layers form a tungstate zigzag viewed from the a-axis, Ln3+ layer and [PO4]3− layer are ordered alternately along the b-axis as shown in Fig. 3(a and b). Therefore, the Ln3+ layer is isolated by two successive [WO4]2− layers on the a-axis and [PO4]3− layer on the b-axis in this three-dimensional network. This special lattice arrangement reveals that the interaction between the Ln3+–Ln3+ layers might be blocked. However, Fig. 3(b and c) show the Ln3+–Ln3+(Dy3+–Dy3+) unit sharing two oxygen atoms (O4′ and O4′), which is close to each other. This will causes the interaction among Ln3+–Ln3+ units greatly enhanced as the doping concentration increasing. For KGPW:0.005Dy3+ sample, the selected bond lengths from the GSAS refinement are listed in Table 1. The Ln cation located at 8d position, which contains a mixture of 99.5% Gd and 0.5% Dy, is coordinated by eight oxygen atoms with two WO4 units and four PO4 units as shown in Fig. 3(d). The Ln–O bond lengths range from 2.3 to 2.5 Å, resulting in an irregular polyhedron.
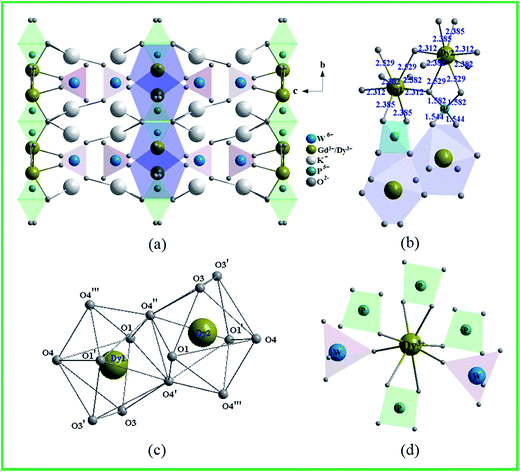 |
| Fig. 3 Crystal structure of KGPW:0.005Dy3+. (a) Viewed from the a-axis, (b) the polyhedral links between DyO8 and PO4 units, (c) two DyO8 units with eight-oxygen coordination atoms, (d) the coordination environment of Dy3+ site. | |
3.2 Photoluminescence characteristics
In order to characterize the existence of [PO4]3− and [WO4]2− units in our obtained samples, the FT-IR spectrum of the pure KGPW is measured and showed in Fig. 4(a). One can see that there are four strong infrared absorption peaks located at 786, 843, 962 and 1081 cm−1. In general, the IR absorption bands of [PO4]3− are located at 650–540 cm−1 and 1120–940 cm−1, originating from the symmetric stretching mode of the [PO4]3− units.17 Thus, two infrared absorption peaks at 962 and 1081 cm−1 are coming from [PO4]3− units. Besides, the other peaks at 786 and 843 cm−1 are assigned to the stretching modes of [WO4]2− units.18 These results indicate that [PO4]3− and [WO4]2− units exist in our obtained sample, which can be deduced in all samples.
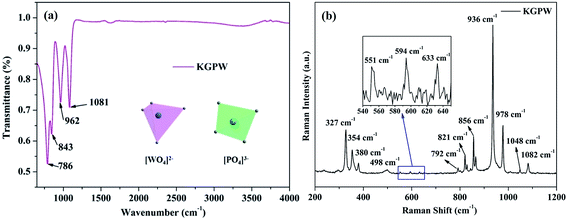 |
| Fig. 4 FT-IR spectrum (a) and Raman spectrum (b) of the pure KGPW sample. | |
Fig. 4(b) shows the Raman spectrum of the pure KGPW sample in the region of 200–1200 cm−1. In free space, [WO4] tetrahedron has Td symmetry.19,20 The basic vibration modes from [WO4]2− units have four internal modes: υ1 (A1), υ2 (E), υ3 (F2) and υ4 (F2). As shown in Fig. 4(b), the strong Raman vibration peak located at 936 cm−1 is assigned to the W–O symmetric stretching vibration υ1 (A1). The Raman peak at 327 cm−1 is attached to O–W–O symmetric bending vibration υ2 (E) while these peaks at 729, 821 and 856 cm−1 are designated as W–O anti-symmetric stretching vibration υ3 (F2), and peaks at 354 and 380 cm−1 should be assigned as O–W–O anti-symmetric bending vibration υ4 (F2). Additionally, [PO4]3− tetrahedron with Td symmetry possesses the same four internal vibration modes. The Raman peak at 978 cm−1 corresponds to the symmetric stretching vibration of P–O, and two weak Raman peaks located at 1048 and 1082 cm−1 are specified as anti-symmetric stretching vibration of P–O. Since [PO4]3− unit is trapped between two DyO8 layers, the symmetric and anti-symmetric bonding vibrations almost limited. As shown in the enlarged image on Fig. 4(b), the weak Raman peak at 551 cm−1 is assigned as O–P–O symmetric bending vibration, and the other two peaks at 594 and 633 cm−1 are classified as W–O anti-symmetric stretching vibration.
The PLE spectrum (monitored at 573 nm) and the PL spectra (excited at 273 and 388 nm) of KGPW:0.005Dy3+ sample, as well as UV-vis DRS are shown in Fig. 5(a). The UV-vis DRS shows a broad absorption band at 200–330 nm and several small absorption bands ranging from 350 to 480 nm. The broad absorption band is attributed to the charge transfer from the oxygen ligands to the central tungsten atom inside the [WO4]2− unit. Besides, several small absorption bands are from f–f transitions of Dy3+ ions, which share the same positions with the results from PLE spectrum as shown in Fig. 5(a). In the PLE spectrum, two sharp bands at 273 and 313 nm are from 8S7/2 → 6I11/2 and 8S7/2 → 6P7/2 transitions of Gd3+ ions, respectively. Other bands at 324, 350, 364, 388, 426, 450 and 472 nm are originating from 6H15/2 → 4P3/2, 6H15/2 → 6P7/2, 6H15/2 → 6P5/2, 6H15/2 → 4I13/2, 6H15/2 → 4G11/2, 6H15/2 → 4I15/2, and 6H15/2 → 4F9/2 f–f transitions of Dy3+ ions.21 The energy levels of Dy3+-activated KGPW sample is presented in Fig. 6(a). All observed peaks of the sample could be found. It is noteworthy that those solid solutions match well with the commercial near-UV chips, suggesting its promising application in phosphor-converted white LEDs. Under 388 nm excitation, the PL spectrum shows three emission bands at 483, 573, and 660 nm originating from 4F9/2 → 6H15/2, 4F9/2 → 6H13/2, and 4F9/2 → 6H11/2 transitions of Dy3+ ions, respectively. It is well-known that the 4F9/2 → 6H15/2 transition following the selection rules which is insensitive to the crystal field strength around the Dy3+ ions. While the 4F9/2 → 6H13/2 transition is attributed to forced electric dipole transition which is strongly influenced by the surrounding environment.22,23 The 4F9/2 → 6H13/2 transition is only allowed at low symmetry with no inversion center. Therefore, when Dy3+ ions occupy low symmetry local site, the yellow prevails. In contrast, the blue emission is dominant. From the PL spectra (see Fig. 5(b)), it is clear that yellow emission intensity is much stronger than that of the blue emission, indicating that Dy3+ ions reside in a low symmetry site. In addition, under 273 nm excitation the PL spectrum shows the duplicate three emission bands, demonstrating the energy transfer from Gd3+ to Dy3+ ions could be occurred.
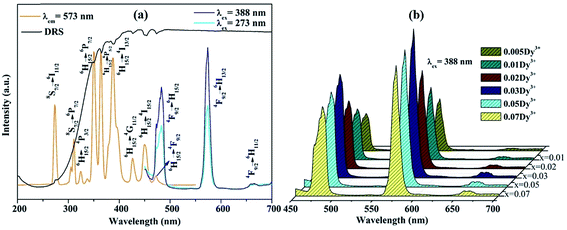 |
| Fig. 5 (a) The PLE and PL spectra, as well as UV-vis DRS of KGPW:0.005Dy3+, (b) the PL spectra of KGPW:xDy3+ (x = 0.005, 0.01, 0.02, 0.03, 0.05 and 0.07). | |
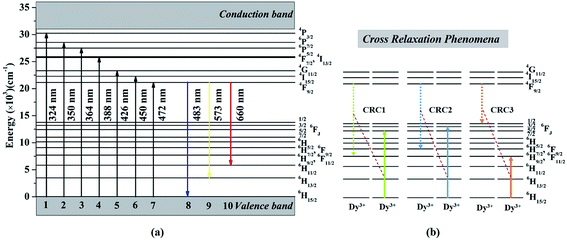 |
| Fig. 6 (a) Energy levels of Dy3+ ions, (b) three possible cross-relaxation processes among Dy3+ ions. | |
In order to further explore the effect of different Dy3+ doping concentrations, a series of KGPW:xDy3+ (x = 0.005, 0.01, 0.02, 0.03, 0.05 and 0.07) phosphors were synthesized and measured as shown in Fig. 5(b). We can see that the emission intensity of Dy3+ rises first as gradual increase Dy3+ doping concentration until the maximum value x at 0.03 and then drops with the similar profile. Fig. 7 shows the optimum concentration for Dy3+-activated KGPW samples is 0.03 mol. This low concentration quenching phenomenon is not consistent with the previously reported by Wen et al.1 As far as we know, the possible reason for the low concentration quenching phenomenon in the obtained phosphors is the cross-relaxation mechanism between neighboring Dy3+ ions. As shown in Fig. 3(b) and Table 1, the Ln–Ln distance along the b-axis is close enough to 4.033 Å. Considering the energy match rule, Fig. 6(b) presents the following three possible cross-relaxation channels (CRC) among Dy3+, denoted as CRC1, CRC2 and CRC3, which are responsible for population decrease of 4F9/2 level:
4F9/2 + 6H15/2 → 6H9/2/6F11/2 + 6F5/2 |
4F9/2 + 6H15/2 → 6H7/2/6F9/2 + 6F3/2 |
4F9/2 + 6H15/2 → 6F1/2 + 6H9/2/6F11/2 |
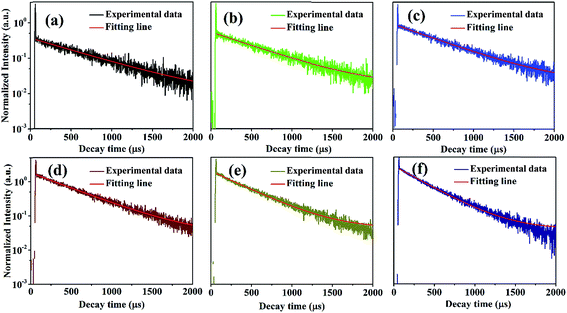 |
| Fig. 7 Decay curves of KGPW:xDy3+ (x = 0.005, 0.01, 0.02, 0.03, 0.05 and 0.07) samples with 388 nm excitation by monitoring at 573 nm. | |
After the above three cross-relaxation processes, the Dy3+ ions at 4F9/2 levels are de-excited to ((6H9/2/6F11/2), 6H7/2/6F9/2) or 6F1/2 levels, and concomitantly the ground states of neighboring Dy3+ ions will accept the energy and be excited to 6F5/2, 6F3/2 or 6H9/2/6F11/2 levels. Finally, all the Dy3+ ions involved in these three cross-relaxation processes reach their ground states, resulting in the luminescence of the 4F9/2 level quenched.
The concentration quenching start an effective role when exceed this optimum concentration causing decreased luminous intensity. In general, the non-radiative energy transfer among Dy3+ ions causing the concentration quenching is dominant, following three types of mechanisms: radiation reabsorption, exchange interaction, or a multipolar–multipole interaction.24 In order to identify the mechanism of energy transfer, the critical distance (RC) between the activated ions for energy transfer can be evaluated according to the equation given by Blasse:25
|
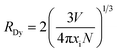 | (1) |
where
N is the number of available sites of Dy
3+ occupying per unit cell,
xi is the critical concentration of Dy
3+, and
V is the volume per unit cell. For KGPW phosphor,
N = 8,
V = 1646.442 Å
3,
x = 0.03, then the
RDy is calculated to be 23.57 Å. Clearly stated, the value of
RC is significantly larger than 5 Å, indicating that multipolar–multipolar interaction type is dominated for the non-radiative energy transfer among Dy
3+ ions.
The representative PL decay curves of the Dy3+ ions were measured with 388 nm excitation by monitoring the emission at 573 nm as shown in Fig. 7. There is a fast decay signal at the initial time in all samples. This signal may be coming from the instrumental response. By fitting the above decay curves, the decay curves in low Dy3+ concentration (0.005 to 0.01, 0.02 mol) are fitted successfully on the single-exponential equation:
|
It = I0 exp(−t/τ)
| (2) |
where
It and
I0 are the PL intensities at time
t and 0,
τ is the decay time. However, the decay curves are non-exponential in KGPW:
xDy
3+ (
x = 0.05 and 0.07) samples. Moreover, their non-exponential increase as the Dy
3+ concentration increases. The nonexponentiality of the decay curves indicates that the probability of energy transfer among Dy
3+ ions increases with increasing Dy
3+ concentration.
26 In order to investigate the mechanisms of Dy–Dy interaction, the evolution of the concentration of the emission decays was analyzed. Assuming that the interaction scheme is a multipolar type, Inokuti–Hirayama's formula is given by:
27 |
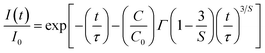 | (3) |
where
τ is the intrinsic lifetime of a single ion,
Γ(1 − 3/
S) is the gamma function,
C is the number of acceptors (quenching sites) per unit volume,
C0 = 3/(4π
Rc3); where
Rc is the critical interaction distance between Dy
3+ and Dy
3+ ions,
S is the electric multipolar series, and the value of
S = 6, 8, and 10 for dipole–dipole (d–d), dipole–quadrupole (d–q), quadrupole–quadrupole (q–q) interactions, respectively. To obtain the value of
S, the above-mentioned
eqn (3) can be rearranged as the following equation:
|
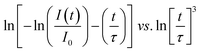 | (4) |
On the basis of the above eqn (4), plots of ln[−ln(I(t)/I0) − (t/τ)] vs. ln[t/τ]3 with the fitting line is given in Fig. 8. The slope of the fitting line is equal to 1/S. The fitting results of KGPW:xDy3+((a) x = 0.05 and (b) x = 0.07) samples are shown in Fig. 8. As can be seen from this figure, the values of S for KGPW:xDy3+(x = 0.05 and 0.07) samples were calculated to be 5.3 and 4.7, both approximated to 6. Therefore, the dominant energy transfer mechanism for Dy3+ ions in KGPW host is d–d interaction.
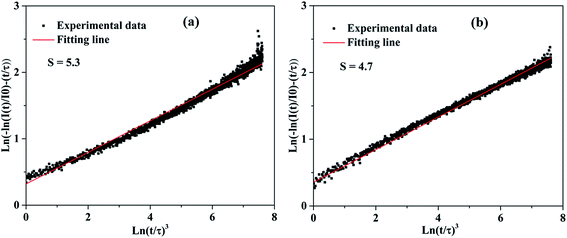 |
| Fig. 8 The plots of ln[−ln(I(t)/I0) − (t/τ)] vs. ln[t/τ]3 with the fitting lines of KGPW:xDy3+ samples ((a) x = 0.05, (b) x = 0.07). | |
Fig. 9 presents the Commission Internationale de l'Eclairage (CIE) chromaticity diagram of KGPW:xDy3+ samples under 388 nm excitation. It is found that the CIE chromaticity coordinates for the Dy3+-activated KGPW samples had warm white emission. The phosphor KGPW:0.005Dy3+ with CIE at (x = 0.33, y = 0.36) is extremely close to the National Television Standards Committee (NTSC) white point (x = 0.33, y = 0.33). The calculated Tcct (Tcct: the temperature at which a radiating black body produces the same color) values located at 5367–5574 K, which are close to that of sunlight at noon. According to the NTSC and European Broadcasting Union (EBU) standards, the color purity of phosphor is an essential technological factor for LEDs display application since it has a major impact on the color rendering index and the light output. The color purity can be estimated by the following expression:28
|
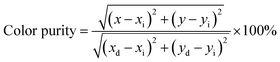 | (5) |
where (
x,
y) is the color coordinate of the sample (
x = 0.331,
y = 0.364), (
xi,
yi) is the CIE color coordinate of white illumination (
xi = 0.333,
yi = 0.333), and (
xd,
yd) is the CIE color coordinate of the dominant wavelength (
xd = 0.305,
yd = 0.350). By using the coordinates of (
x,
y), (
xi,
yi), (
xd,
yd), the color purity is determined to be approximately 90%, suggesting that the Dy
3+-activated KGPW phosphors with high purity and warm white emissions may have promising applications in w-LEDs. The QE of KGPW:0.03Dy
3+ phosphor with the highest PL intensity is measured as 18.98%. The result is compared with the reported phosphors in
Table 3.
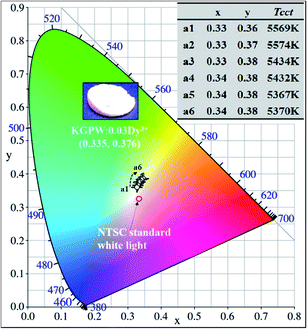 |
| Fig. 9 The CIE chromaticity coordinates of KGPW:xDy3+ samples under 388 nm excitation. Inset shows the calculated CIE chromaticity coordinates and the correlated color temperatures (Tcct). | |
Table 3 The internal quantum efficiency (QE) of KGPW:0.05Dy3+ phosphor compared with the selected results from reported references
Sample |
Excitation wavelength (nm) |
QE (%) |
Note |
KGPW:0.05Dy3+ |
364 |
18.98 |
This work |
Ca2.95Si2O7:0.05Dy3+ |
365 |
15.16 |
33 |
Ca0.98MoO4:0.02Dy3+,0.02K+ |
351 |
8 |
34 |
La1.95W2O9:0.05Dy3+ |
398 |
21.3 |
35 |
MgY3.99Si3O13:0.01Dy3+ |
347 |
21.6 |
36 |
3.3 Thermal properties analysis
In general, phosphors suffer a decrease of emission intensity in the process of phosphors' application with temperature increasing. Therefore, the thermal stability of photoluminescence is of great significance for phosphor as it greatly affects not only the color rendering index but also the light output of w-LEDs. The temperature-dependent luminescence properties of KGPW:0.05Dy3+ phosphor excited at 273 and 388 nm were tracked with temperature increasing from 303 to 463 K as shown in Fig. 10(a and b). One can see that the emission intensities of KGPW:0.05Dy3+ sample decrease progressively with temperature increasing. Moreover, as the temperature increases to 423 K (150 °C), the emission intensities of KGPW:0.05Dy3+ phosphor under 273 nm and 388 nm excitation are 25.3% and 76.8% of their initial intensities at room temperature, respectively, as shown in the inset of Fig. 10(a and b). It is notable that the obtained phosphors have excellent thermal stability with 388 nm excitation, which is an essential condition for practical application.
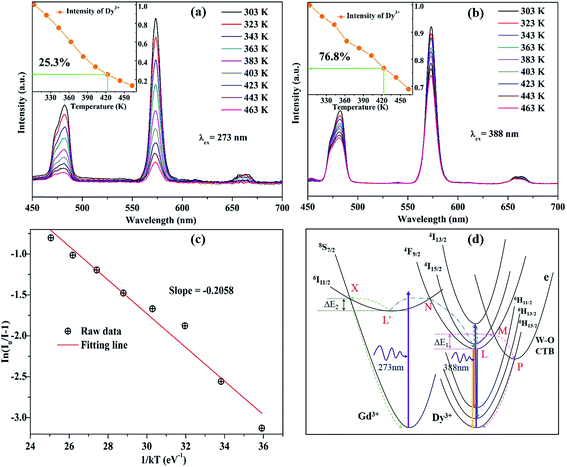 |
| Fig. 10 The temperature-dependent luminescence properties of KGPW:0.05Dy3+ phosphor were tracked with temperature from 303 K to 463 K under 273 nm (a) and 388 nm (b) excitation. (c) Plots of ln[I0/I − 1] against 1/kT of KGPW:0.05Dy3+ phosphor under 388 nm excitation. (d) The configurational coordinate diagram of the ground and excited states of Gd3+ and Dy3+, as well as the CTB of WO4. | |
To further understand the temperature-dependent photoluminescence properties, the activation energy of thermal quenching can be calculated from the Arrhenius equation:29–31
|
 | (6) |
in which
I0 is the initial emission intensity at room temperature and
I is the emission intensity at testing temperature
T (K),
Ea is the activation energy for the thermal quenching,
k is the Boltzmann constant (8.62 × 10
−5 eV K
−1) and
A is a constant for a certain host. The
eqn (6) can be rearranged as follows:
|
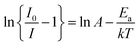 | (7) |
Fig. 10(c) depicts plots of ln[I0/I − 1] against 1/kT of KGPW:0.05Dy3+ phosphor under 388 nm excitation. Through a best-fit using eqn (7), the activation energy Ea for thermal quenching were deduced to be 0.2058 eV for 388 nm excitation.
The configurational coordinate diagram of the ground and excited states of Gd3+ and Dy3+, as well as the charge transfer band (CTB) of WO4 are displayed in Fig. 10(d). The curves of 8S7/2 and 6I11/2 represent the ground and excited states of Gd3+, respectively. The curves of 6H15/2–6H13/2–6H11/2 and 4F9/2–4I15/2–4I13/2 represent the ground and excited states of Dy3+, respectively. The curves e represents the excited state of CTB of WO4 units. Points L and L′ are the lowest states positions of 4F9/2 and 6I11/2, respectively. X and N are the crossing points of 6I11/2 to 8S7/2 and to 4F9/2 states, M and P are the crossing points of 4F9/2 to e and e to 6H15/2, respectively. Under the excitation of 388 nm, the electrons in 6H15/2 ground state absorb the energy and transfer it to 4I13/2 excited state at room temperature. Then, the electrons of Dy3+ at 4I13/2 state undergo a non-radiative process relaxing to the 4F9/2, from where the electrons return to the ground state 6H15/2–6H13/2–6H11/2 by radiative transition. With the increase of temperature, the phonon vibration is strengthened and the electrons at the lowest excited state (4F9/2) L position can absorb the phonon energy ΔE1. Simultaneously, the electrons excited from point L to M, then drops to P, from where the electrons undergo a non-radiative process relaxing to 6H15/2, leading to the rapid decrease of electron number. Besides, under the excitation of 273 nm, the electrons in 8S7/2 ground state of Gd3+ absorb the energy and be excited to 6I11/2 state at room temperature. Then, the electrons cross point N showing energy transfer from Gd3+ to Dy3+ ions. As the temperature increases, the phonon vibration is strengthened and the electrons at the lowest excited state (6I11/2) L′ position will be likely to overcome the phonon energy ΔE2 relaxed to the ground state (8S7/2). This process greatly reduced the energy transfer from Gd3+ to Dy3+ causing the rapid luminescence quenching of Dy3+, which indicates that energy transfer efficiency is significantly influenced by temperature. The energy transfer efficiency (ηT) from the Gd3+ to Dy3+ ions can be approximated calculated by the following equation:32
|
 | (8) |
where
It and
I0 are the luminescence intensity of the Dy
3+ ion at different temperature and at room temperature, respectively. By calculation, the
ηT from the Gd
3+ to Dy
3+were decreased from 86.7%, 73.1%, 59.7%, 43.5%, 32.6%, 25.3%, 18.7%, to 13.5% as the temperature increases from 323 K, 343 K, 363 K, 383 K, 403 K, 423 K, 443 K, to 463 K, respectively.
In the above discussion, we have elaborated that there are significant differences in temperature-dependent photoluminescence between 273 and 388 nm excitations. Obviously, the emission intensity at 388 nm excitation is more stable than that at 273 nm excitation. When excited at 273 nm, the direct reason for the rapid luminescence quenching of Dy3+ is that the energy migrated from Gd3+ to Dy3+ ions greatly reduced as the temperature increases. Specifically, in the energy distribution, the level of electrons overcoming ΔE2 increases with the temperature increasing, resulting that the number of electrons from Gd3+ to Dy3+ rapid decreases in KGPW host.
4. Conclusion
In conclusion, multi-wavelength excited white-light emitting Dy3+-activated KGPW phosphors were synthesized successfully by using the solid state method. Photoluminescence properties were systematically investigated, the PLE spectrum is consisted of a series of sharp peaks ranging from 270 nm to 500 nm, which match well with the commercial near-UV chips suggesting its promising application in phosphor-converted white LEDs. Under the excitation of 388 nm, the PL spectrum shows three emission bands from 4F9/2 → 6H15/2 (483 nm), 4F9/2 → 6H13/2 (573 nm) and 4F9/2 → 6H15/2 (660 nm) transitions of Dy3+ ions. In addition, the PL spectrum shows the duplicate three emission bands upon 273 nm excitation, demonstrating the energy transfer from Gd3+ to Dy3+ ions could be occurred. The quenching concentration was found to be 0.03 mol. It is notable that, as the temperature increases to 423 K (150 °C) the emission intensities of KGPW:0.05Dy3+ phosphor under 273 nm and 388 nm excitation are 25.3% and 76.8% of their initial intensities at room temperature, respectively, which show the obtained phosphors have excellent thermal stability with 388 nm excitation. In addition, the Dy3+-activated KGPW phosphors show high purity (90%) warm white emission. All the results indicate that the multi-wavelength excited white-emitting Dy3+-activated KGPW phosphors have a potential application for white light LEDs.
Acknowledgements
This research was supported by the Basic Science Research Program through the National Research Foundation of Korea (NRF) funded by the Ministry of Science, ICT and Future Planning (No. 2015060315). The phosphate–tungstate K2Gd(1−x)(PO4)(WO4):xDy3+ phosphor was supplied by the Display and Lighting Phosphor Bank at Pukyong National University.
References
- D. Wen, J. Feng, J. Li, J. Shi, M. Wu and Q. Su, J. Mater. Chem. C, 2015, 3, 2107–2114 RSC.
- Y. Guo, B. K. Moon, B. C. Choi, J. H. Jeong and J. H. Kim, J. Lumin., 2017, 181, 96–102 CrossRef CAS.
- C. Li, J. Dai, J. Huang, D. Deng, H. Yu, L. Wang, Y. Ma, Y. Hua and S. Xu, Ceram. Int., 2016, 42, 6891–6898 CrossRef CAS.
- X. Fu, W. Lü, M. Jiao and H. You, Inorg. Chem., 2016, 55, 6107–6113 CrossRef CAS PubMed.
- T. Selvalakshmi, S. Sellaiyan, A. Uedono and A. C. Bose, RSC Adv., 2014, 4, 34257–34266 RSC.
- H. Chen, H. Lin, J. Xu, B. Wang, Z. Lin, J. Zhou and Y. Wang, J. Mater. Chem. C, 2015, 3, 8080–8089 RSC.
- P.-P. Dai, C. Li, X.-T. Zhang, J. Xu, X. Chen, X.-L. Wang, Y. Jia, X. Wang and Y.-C. Liu, Light: Sci. Appl., 2016, 5, e16024 CrossRef CAS.
- J. Zhao, C. Guo, X. Su, H. M. Noh and J. H. Jeong, J. Am. Ceram. Soc., 2014, 97, 1878–1882 CrossRef CAS.
- M. Shang, C. Li and J. Lin, Chem. Soc. Rev., 2014, 43, 1372–1386 RSC.
- Q. Liu, Y. Liu, Y. Ding, Z. Peng, X. Tian, Q. Yu and G. Dong, Ceram. Int., 2014, 40, 10125–10129 CrossRef CAS.
- Z. Ci, Q. Sun, S. Qin, M. Sun, X. Jiang, X. Zhang and Y. Wang, Phys. Chem. Chem. Phys., 2014, 16, 11597–11602 RSC.
- G. Zhu, Y. Wang, Q. Wang, X. Ding, W. Geng and Y. Shi, J. Lumin., 2014, 154, 246–250 CrossRef CAS.
- M. Daub, A. J. Lehner and H. A. Höppe, Dalton Trans., 2012, 41, 12121–12128 RSC.
- L. Han, X. Xie, J. Lian, Y. Wang and C. Wang, J. Lumin., 2016, 176, 71–76 CrossRef CAS.
- B. H. Toby, J. Appl. Crystallogr., 2001, 34, 210–213 CrossRef CAS.
- K. V. Terebilenko, I. V. Zatovsky, V. N. Baumer, N. S. Slobodyanik and O. V. Shishkin, Acta Crystallogr., Sect. E: Struct. Rep. Online, 2008, 64, i75 CAS.
- K. Li, Y. Zhang, X. Li, M. Shang, H. Lian and J. Lin, Dalton Trans., 2015, 44, 4683–4692 RSC.
- K. K. Rasu, D. Balaji and S. M. Babu, J. Lumin., 2016, 170, 547–555 CrossRef CAS.
- S. Nishigaki, S. Yano, H. Kato, T. Hirai and T. Nonomura, J. Am. Ceram. Soc., 1988, 71, C11–C17 CrossRef CAS.
- P. Pereira, A. De Moura, I. Nogueira, M. Lima, E. Longo, P. de Sousa Filho, O. Serra, E. Nassar and I. Rosa, J. Alloys Compd., 2012, 526, 11–21 CrossRef CAS.
- D. Wang, J. Fan, M. Shang, K. Li, Y. Zhang, H. Lian and J. Lin, Opt. Mater., 2016, 51, 162–170 CrossRef CAS.
- G. B. Nair and S. Dhoble, RSC Adv., 2015, 5, 49235–49247 RSC.
- P. S. Babu, P. P. Rao, S. Mahesh, T. L. Francis and T. Sreena, Mater. Lett., 2016, 170, 196–198 CrossRef CAS.
- Y. Guo, B. K. Moon, B. C. Choi, J. H. Jeong, J. H. Kim and H. Choi, Ceram. Int., 2016, 42, 18324–18332 CrossRef CAS.
- G. Blasse, Phys. Lett. A, 1968, 28, 444–445 CrossRef CAS.
- S.-D. Jee, J. K. Park and S.-H. Lee, J. Mater. Sci., 2006, 41, 3139–3141 CrossRef CAS.
- M. Inokuti and F. Hirayama, J. Chem. Phys., 1965, 43, 1978–1989 CrossRef CAS.
- W. Xiao, X. Zhang, Z. Hao, G.-H. Pan, Y. Luo, L. Zhang and J. Zhang, Inorg. Chem., 2015, 54, 3189–3195 CrossRef CAS PubMed.
- Y. Chen, F. Pan, M. Wang, X. Zhang, J. Wang, M. Wu and C. Wang, J. Mater. Chem. C, 2016, 4, 2367–2373 RSC.
- L. Tian, J. Shen, T. Xu, L. Wang, L. Zhang, J. Zhang and Q. Zhang, RSC Adv., 2016, 6, 32381–32388 RSC.
- Z.-C. Wu, H.-H. Fu, J. Liu, S.-P. Kuang, M.-M. Wu, J.-G. Xu and X.-J. Kuang, RSC Adv., 2015, 5, 42714–42720 RSC.
- F. Du, W. Zhuang, R. Liu, Y. Liu, J. Zhong, P. Gao, X. Zhang, W. Gao and L. Shao, RSC Adv., 2017, 7, 1075–1081 RSC.
- X. Zhang, Z. Lu, F. Meng, L. Hu, X. Xu, J. Lin and C. Tang, Mater. Lett., 2012, 79, 292–295 CrossRef CAS.
- S. Dutta, S. Som and S. Sharma, RSC Adv., 2015, 5, 7380–7387 RSC.
- T. Nakajima, H. Hanawa and T. Tsuchiya, J. Ceram. Process. Res., 2016, 17, 485–488 Search PubMed.
- Z. Ci, R. Guan, L. Jin, L. Han, J. Zhang, J. Ma and Y. Wang, CrystEngComm, 2015, 17, 4982–4986 RSC.
|
This journal is © The Royal Society of Chemistry 2017 |