DOI:
10.1039/C7RA02201H
(Paper)
RSC Adv., 2017,
7, 21585-21591
Computational assessment of nitrogen-rich peracids: a family of peroxide-based energetic materials†
Received
22nd February 2017
, Accepted 3rd April 2017
First published on 18th April 2017
Abstract
Nitrogen-rich 5- and 6-membered compounds substituted with nitro and peracid groups were designed and investigated using density functional theory (DFT). The calculated energetic properties of peracid compounds were compared with those of some classical explosives such as TNT and RDX. The computed results show that most of the designed peracids possess large positive heats of formation (HOF) and much higher densities (1.79–1.89 g cm−3). The predicted detonation properties impart performance superior to that of TNT and comparable to that of RDX. The relatively better oxygen balance results in higher heat of detonation and more gaseous combustion products. Based on the predicted energetic properties of the designed compounds, the present study emphasizes the potential of the peracid group in developing new energetic materials.
Introduction
The search and development of energetic materials with tunable properties is an unalterable goal for researchers working in this field as these materials belong to the class of hazardous materials. The importance of energetic materials in the civil and military field has led to significant growth in environmentally friendly compounds in recent years.1–9 Furthermore, designing a new compound and estimating its energetic properties is a typical practice before synthesis to reduce the cost and associated hazardous effects.10–13 Covalent peroxides (O–O) are classified as peracid (RC(O)OOH), perester (RC(O)OOR′), perether (ROOR′), and peracetal (ROOR′OOR) compounds.14 TATP (triacetone triperoxide) and HMTD (hexamethylene triperoxide diamine) are known compounds of peroxide explosives containing three –O–O– linkages per molecule (see Fig. S1 in the ESI†). They are highly sensitive and brisant in nature, and their blast strength has been reported to be 88% and 60% that of TNT and RDX, respectively. In this study, we focused on the design of peracid (RC(O)OOH) group-containing explosives, which have not been widely explored. In recent studies, Klapötke et al.15,16 have reported peroxide-containing explosives with reasonable sensitivity and performance and suggested the incorporation of peroxide-containing functional groups to improve the oxygen balance. Since peracid group has more oxygen atoms, it is expected that this would help in the rapid self-decomposition of a compound. In this study, we selected nitrogen-rich backbones that have been reported in the literature1,17–22 and introduced the peracid group along with the nitro group. Herein, nitrogen-rich peracids have been described as promising energetic materials and potential propellants. Theoretical methods and correlations were used to investigate their electronic structure, heat of formation (HOF), density, performance properties, and sensitivity. We believe that our theoretical studies of peracid compounds will aid further investigations in the incorporation of peracid functionalities in energetic materials.
Results and discussion
Fig. 1 lists the molecular structures of the designed nitrogen-rich peracid compounds. For each of these, we optimized the structures and computed the surface properties at the B3PW91/6-31G (d,p) level using the Gaussian 09 (ref. 23) and Multiwfn program.24 All of the optimized peracid structures were characterized to be true local energy minima on their potential energy surfaces without imaginary frequencies. The computational methodology used for the calculation of energetic properties is similar to that reported in our earlier studies25–29 and has been illustrated in the ESI.† The nitro compounds P4, P6, P10, P13, P16, P19, P25, and P28 have been reported in the literature30–39 and their energetic properties were compared with those of the designed peracid analogues.
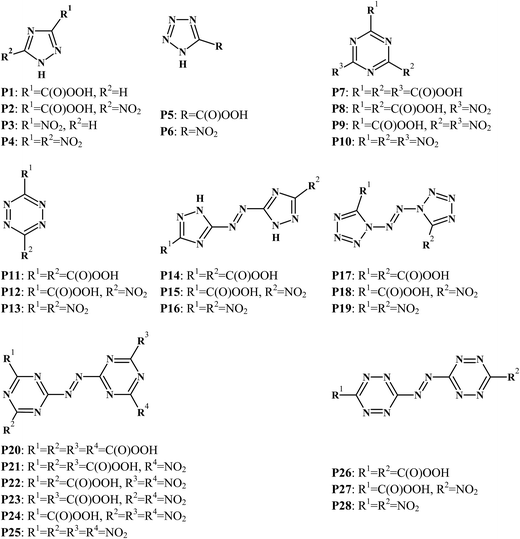 |
| Fig. 1 Molecular structures of the peracid compounds. | |
Heat of formation
The heat of formation (HOF) is an important thermochemical property for an explosive, and it plays a significant role in the prediction of the heat of explosion, heat of combustion, and other performance parameters. In the present study, HOFgas was predicted using an isodesmic reaction approach with single point energies calculated at the B3PW91/6-31G(d,p) level. Detailed information of the designed isodesmic reactions, carried out to obtain HOFgas of peracid derivatives, has been provided in the ESI (Fig. S2†). HOFsolid for all the peracid derivatives were obtained by subtracting the heat of sublimation (HOFsub) from HOFgas, according to the Politzer approach. Table 1 summarizes the calculated oxygen balance, nitrogen content, HOFgas, HOFsub, and HOFsolid of the peracid derivatives. It was observed that the calculated HOFgas of P6, P13, P19, and P25 are close to the reported values, indicating that our calculated results are reliable. Compared with other nitrogen-rich peracid derivatives, P27 has an extremely high HOFsolid of 867 kJ mol−1, may stem from the rich N–N and C–N bonds of the s-tetrazine ring and azo bond in the compound. All the peracid substituted derivatives possess lower HOFsolid when compared to the corresponding nitro derivatives, indicating that the peracid groups decrease the HOF. We observed that the peracid group was unfavourable for increasing the HOF, resulting in the negative values. All the designed peracid derivatives possess negative HOFsolid except for P12, P15, P17, P18, P24, P26, and P27. Among P14–15 and P17–18, HOFsolid of the compounds P17–18 were greater, owing to higher energy contribution from the tetrazole rings. This may be due to difference in the HOFs of triazine and tetrazine peracid derivatives.
Table 1 Calculated oxygen balance (OB, %), nitrogen content (NC, %), density (ρ, g cm−3), heat of formation in gas phase (HOFgas, kJ mol−1) and solid phase (HOFsolid, kJ mol−1), and heat of sublimation (HOFsub, kJ mol−1) of the peracid compoundsa
Compd |
OB (%) |
NC (%) |
ρ (g cm−3) |
HOFgas (kJ mol−1) |
HOFsub (kJ mol−1) |
HOFsolid (kJ mol−1) |
The parenthesis values indicate the reported HOFgas. |
P1 |
−56 |
33 |
1.79 |
−80 |
99 |
−179 |
P2 |
−18 |
32 |
1.85 |
−71 |
97 |
−168 |
P3 |
−42 |
49 |
1.80 |
192 |
92 |
100 |
P4 |
−05 |
44 |
1.87 |
204 |
88 |
116 |
P5 |
−25 |
43 |
1.82 |
58 |
90 |
−32 |
P6 |
−07 |
61 |
1.85 |
338 (363)32 |
74 |
264 |
P7 |
−28 |
16 |
1.85 |
−534 |
144 |
−678 |
P8 |
−20 |
23 |
1.86 |
−270 |
126 |
−396 |
P9 |
−10 |
30 |
1.88 |
−4 |
108 |
−112 |
P10 |
00 |
39 |
1.89 |
265 |
92 |
173 |
P11 |
−24 |
28 |
1.83 |
−11 |
103 |
−114 |
P12 |
−13 |
37 |
1.84 |
260 |
86 |
174 |
P13 |
00 |
49 |
1.85 |
534 (533)34 |
70 |
464 |
P14 |
−45 |
39 |
1.81 |
144 |
180 |
−36 |
P15 |
−39 |
47 |
1.83 |
394 |
166 |
228 |
P16 |
−31 |
55 |
1.84 |
645 |
150 |
495 |
P17 |
−17 |
49 |
1.83 |
642 |
157 |
485 |
P18 |
−09 |
57 |
1.84 |
934 |
140 |
794 |
P19 |
00 |
66 |
1.85 |
1229 (1186)37 |
123 |
1106 |
P20 |
−37 |
26 |
1.80 |
−289 |
303 |
−592 |
P21 |
−33 |
30 |
1.81 |
−28 |
283 |
−311 |
P22 |
−28 |
35 |
1.82 |
239 |
261 |
−22 |
P23 |
−28 |
35 |
1.82 |
237 |
260 |
−23 |
P24 |
−23 |
40 |
1.83 |
506 |
239 |
267 |
P25 |
−17 |
46 |
1.84 |
774 (770)38 |
217 |
557 |
P26 |
−36 |
45 |
1.80 |
762 |
184 |
578 |
P27 |
−30 |
52 |
1.81 |
1032 |
165 |
867 |
P28 |
−23 |
60 |
1.83 |
1304 |
147 |
1157 |
Density and oxygen balance
Density is an essential physical parameter to calculate the detonation performance of an energetic material.40–43 A higher density value also ensures the packing of more material per unit volume, thereby results in a greater explosion. The densities of the peracid derivatives were predicted using the Politzer et al.44 approach (Table 1) and was found in the range of 1.79–1.88 g cm−3, which were much higher than those of TNT (1.65 g cm−3) and comparable to those of RDX (1.80 g cm−3). Note that replacement of peracid group by nitro does not show a significant change in the density. However, nitro group has a slight advantage over peracid functionality in terms of improved density. The hydrogen atoms in peracid groups and N–H of the azole rings may help in building strong inter- and intra-molecular hydrogen bond network, thereby contributing in improving the density at the same time. It was observed that densities of triazine peracid derivatives (P7–9 and P20–24) were higher than those of tetrazine peracid derivatives (P11–12 and P26–27). This could be attributed to the substitution of peracid and nitro groups in triazine derivatives. Along with density, oxygen balance (OB) is also an essential parameter that indicates the index of oxygen in a compound required to convert all the carbon and hydrogen atoms into CO2 and H2O, respectively, releasing most of the energy. The designed peracids have a nitrogen-rich backbone, which is beneficial to achieve better OB. Among the designed compounds, P10, P13, and P19 possess neutral oxygen balance, indicating that oxygen is sufficient for their complete combustion. Moreover, other compounds such as P2, P4, P6, P8, P9, P12, P17, P18, and P25 have good OB, better than those of RDX (−21.6%) and HMX (−21.6%). The deceased C–H group in the backbone of the tetrazole peracid derivatives (P5, P17, and P18) helped to achieve better OB than that of the corresponding triazole derivatives (P1, P14, and P15). The high densities and OB were apparently ascribed to the high nitrogen content, and peracid and nitro groups in these compounds.
Performance parameters
Detonation velocity (D), pressure (P), heat of detonation (Q), explosive power (EP), power index (PI), brisance, Gurney velocity
, and heat of combustion (ΔHc) are important measures of the performance of detonating explosives. All these performance parameters have been listed in Table 2. Fig. 2 and 3 compare the energetic properties of the peracid compounds with those of TNT and RDX. The designed peracids have detonation velocities ranging from 7.14 (P1) to 8.96 km s−1 (P18) and pressures were found in the range from 22.53 (P1) to 36.06 GPa (P18), which were higher than those of TNT (D = 6.94 km s−1 and P = 22.0 GPa). The compounds P12 and P18 have D and P comparable with RDX (D = 8.60 km s−1 and P = 33.92 GPa). Among these, P18 has the highest D and P of 8.96 km s−1 and 36.06 GPa, respectively. It can be seen from Table 2 that all peracids show Q values higher than 1100 cal g−1, except for P1, P7, P14, and P20. The Q values of these compounds are comparable or higher than those of the common explosives TNT (1043 cal g−1) and RDX (1138 cal g−1). Power index (PI) is an important parameter to represent the strength of the explosives, and it depends on the volume of the gas liberated during the explosion and the heat of detonation. In peracid derivatives, P18 (170%) surpassed the power index of RDX (169%) due to more volume of gaseous explosion products, whereas P2, P9, P11, P12, P17, P26, and P27 were found to be more powerful than TNT (116%). Most of the designed peracids have negative OB, eventually reducing the gaseous explosion products and performance. Detonation performance is also linked with brisance, and superior brisance indicates effectiveness of an explosion in the fragmentation of shells and casings.45 The estimated brisance values relative to that of TNT (∼100) for peracid derivatives were found in the range from 96 to 142. Compared to TNT, designed compounds exhibited higher brisance values (except for P1), whereas those of P18 and P24 were comparable with that of RDX (140). Gurney46 has derived a series of equations to account the effectiveness of an explosive that will accelerate/fragment the surrounding layer of metal or other material when detonated. Gurney velocity is a useful parameter to estimate the energy output of the detonating explosive. In the present study, we used the Kamlet–Finger method47 to calculate Gurney velocity of designed peracid derivatives. To validate the consistency of Kamlet–Finger method, Gurney velocities were also calculated using the Hardesty–Kennedy method48 (see Table 2). It was found that both these methods resulted in comparable values. From the computed
values (in Table 2), P18 (2.96 km s−1) showed highest value among the peracids and RDX (2.93 km s−1) due to the higher kinetic energy of its explosion products. All the other designed peracids possess better
when compared to TNT (2.37 km s−1), ranging from 2.38 to 2.96 km s−1. Overall, the designed peracids have performance parameters between TNT and RDX. ΔHc mainly depends on the composition of the molecule and are required to account the total combustion energy of explosives and decomposition products. The higher oxygen balance (OB) ensures the supply of oxygen during the explosion process and results in a large amount of gaseous combustion products. In the present study, ΔHc is predicted to account the heat released during the combustion and has been summarized in Table 2. ΔHc values were always higher than those of Q as oxygen was supplied to the explosive and complete conversion of C and H atoms to CO2 and H2O occurred, respectively. The detonation products of the designed peracids have been listed in Table S4.† Among the designed peracids, P1, P14, P15, P26, and P27 possess superior ΔHc when compared to RDX (2255 cal g−1).
Table 2 Calculated detonation velocity (D, km s−1), detonation pressure (P, GPa), chemical energy of detonation (Q, cal g−1), explosive power (EP, kJ dm3 g−2), power index (PI, %), heat of combustion (ΔHc, cal g−1), brisance, and Gurney velocity (
, km s−1) of peracid compounds
Compd |
D (km s−1) |
P (GPa) |
Q (cal g−1) |
EP (kJ dm3 g−2) |
PI (%) |
ΔHc (cal g−1) |
Brisance |
 (km s−1) |
K–F methoda |
H–K methodb |
Kamlet–Finger method [ref. 47]. Hardesty–Kennedy method [ref. 48]. |
P1 |
7.14 |
22.53 |
886 |
2111 |
78 |
2639 |
96 |
2.38 |
2.44 |
P2 |
8.15 |
29.97 |
1181 |
3304 |
122 |
1777 |
107 |
2.69 |
2.69 |
P3 |
7.84 |
27.28 |
1129 |
3118 |
115 |
2451 |
102 |
2.61 |
2.62 |
P4 |
8.76 |
34.78 |
1391 |
3910 |
145 |
1569 |
113 |
2.88 |
2.84 |
P5 |
8.02 |
28.72 |
1108 |
2846 |
105 |
1905 |
105 |
2.66 |
2.66 |
P6 |
8.90 |
35.72 |
1413 |
4315 |
160 |
1659 |
111 |
2.94 |
2.88 |
P7 |
7.66 |
26.45 |
1061 |
2279 |
84 |
1928 |
104 |
2.53 |
2.56 |
P8 |
8.02 |
29.06 |
1186 |
2850 |
106 |
1799 |
110 |
2.64 |
2.65 |
P9 |
8.44 |
32.40 |
1332 |
3403 |
126 |
1658 |
116 |
2.77 |
2.76 |
P10 |
8.87 |
35.89 |
1497 |
3900 |
144 |
1497 |
122 |
2.91 |
2.86 |
P11 |
8.16 |
29.80 |
1315 |
3274 |
121 |
2061 |
107 |
2.70 |
2.69 |
P12 |
8.63 |
33.49 |
1508 |
4039 |
150 |
1912 |
113 |
2.85 |
2.81 |
P13 |
9.17 |
37.90 |
1738 |
4737 |
175 |
1738 |
119 |
3.02 |
2.95 |
P14 |
7.52 |
25.12 |
1038 |
2466 |
91 |
2431 |
119 |
2.50 |
2.54 |
P15 |
7.85 |
27.60 |
1136 |
2870 |
106 |
2326 |
125 |
2.60 |
2.62 |
P16 |
8.18 |
30.07 |
1248 |
3344 |
124 |
2215 |
131 |
2.70 |
2.70 |
P17 |
8.57 |
32.91 |
1428 |
3971 |
147 |
1956 |
136 |
2.84 |
2.80 |
P18 |
8.96 |
36.06 |
1587 |
4586 |
170 |
1866 |
142 |
2.96 |
2.90 |
P19 |
9.38 |
39.63 |
1767 |
5178 |
192 |
1768 |
148 |
3.09 |
3.00 |
P20 |
7.38 |
24.17 |
1037 |
1940 |
72 |
2181 |
124 |
2.46 |
2.50 |
P21 |
7.60 |
25.70 |
1110 |
2331 |
86 |
2114 |
130 |
2.52 |
2.56 |
P22 |
7.83 |
27.40 |
1194 |
2740 |
101 |
2047 |
136 |
2.60 |
2.61 |
P23 |
7.83 |
27.40 |
1194 |
2737 |
101 |
2046 |
136 |
2.60 |
2.61 |
P24 |
8.08 |
29.25 |
1285 |
3144 |
116 |
1974 |
142 |
2.67 |
2.68 |
P25 |
8.34 |
31.27 |
1383 |
3541 |
131 |
1895 |
148 |
2.76 |
2.74 |
P26 |
8.08 |
28.98 |
1390 |
3513 |
130 |
2483 |
131 |
2.69 |
2.68 |
P27 |
8.39 |
31.33 |
1517 |
4007 |
148 |
2410 |
137 |
2.79 |
2.76 |
P28 |
8.76 |
34.35 |
1659 |
4563 |
169 |
2331 |
143 |
2.90 |
2.85 |
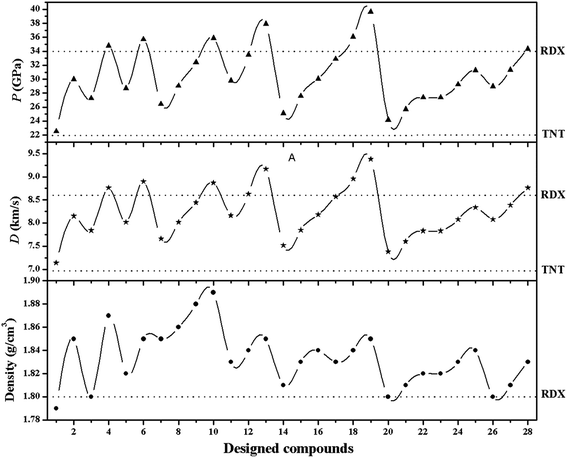 |
| Fig. 2 Correlation between density, detonation velocity (D), and pressure (P) of the peracid compounds and their comparison with those of TNT and RDX. | |
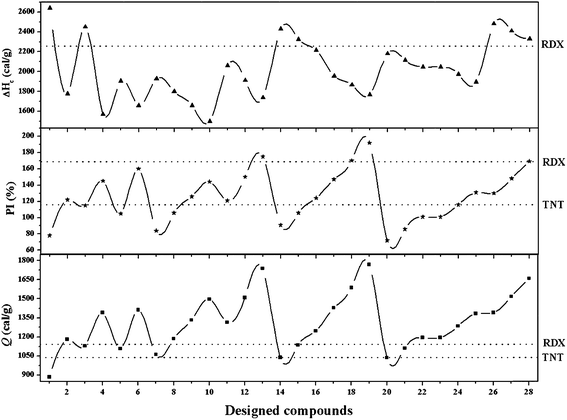 |
| Fig. 3 The heat of detonation (Q), power index (PI), and the heat of explosion (ΔHe) of the peracid compounds. | |
Sensitivity correlations
Along with higher detonation properties, the explosive candidate should be less sensitive towards external stimuli, concomitantly improving chances for practical application. Politzer et al.49 have suggested that the heat of detonation (Q) can be used to assess the sensitivity of energetic materials and sensitivity of energetic material tend to increase as Q becomes higher. The predicted Q values have been listed in Table 2, and it can be seen that P2, P8–9, P11–12, P17–18, and P22–27 have higher Q values in comparison to TNT (1044 cal g−1) and RDX (1138 cal g−1), indicating their sensitivity behavior. It was observed that replacement of peracid group with the nitro group increased the sensitivity of the resultant compounds. Therefore, adjusting the peracid and nitro groups in nitrogen-rich framework is an efficient way to reduce sensitivity without losing too much performance. However, further studies are required to understand the influence of π conjugated system on the stability of the framework.
Conclusions
In this study, 20 peracid-substituted nitrogen-rich compounds were designed, and their energetic properties were investigated using computational methods. Most of the peracid compounds showed comparable/higher density (in the range of 1.79–1.88 g cm−3) than TNT and RDX. In addition, these compounds exhibited good energetic properties (detonation velocity 7.14–8.96 km s−1, detonation pressure 22.5–36.0 GPa, power index 78–170%, brisance 96–142, and Gurney velocity 2.38–2.96 km s−1) that surpassed those of TNT. Moreover, heat of detonation was used as the measure of sensitivity, and most of these compounds exhibited acceptable sensitive behavior. The good energetic properties imply the explosophoric nature of the peracid group and suggest its potential in designing new energetic materials.
Acknowledgements
This work was supported by the grant received from DST-SERB, Government of India (Young Scientists, No. SB/FT/CS-110/2014). Alka Devi would like to thank CSIR for research fellowship (No. 09/1050(0005) 2015-EMR-1).
References
- H. Gao and J. M. Shreeve, Chem. Rev., 2011, 111, 7377–7436 CrossRef CAS PubMed.
- R. D. Chapman, W. S. Wilson, J. W. Fronabarger, L. H. Merwin and G. S. Ostrom, Thermochim. Acta, 2002, 384, 229–243 CrossRef CAS.
- P. F. Pagoria, G. S. Lee, A. R. Mitchell and R. D. Schmidt, Thermochim. Acta, 2002, 384, 187–204 CrossRef CAS.
- A. K. Sikder and N. Sikder, J. Hazard. Mater., 2004, 112, 1–15 CrossRef CAS PubMed.
- D. M. Badgujar, M. B. Talawar, S. N. Asthana and P. P. Mahulikar, J. Hazard. Mater., 2008, 151, 289–305 CrossRef CAS PubMed.
- T. M. Klapötke, High Energy Density Materials, Springer, Berlin, Heidelberg, 2007 Search PubMed.
- H. G. Ang and S. Pisharath, Energetic Polymers, Wiley-VCH Verlag & Co. KGaA, Weinheim, Germany, 2012 Search PubMed.
- J. Zhang, D. Srinivas, L. A. Mitchell, D. A. Parrish and J. M. Shreeve, J. Am. Chem. Soc., 2016, 138, 7500–7503 CrossRef CAS PubMed.
- D. Srinivas, L. A. Mitchell, D. A. Parrish and J. M. Shreeve, Chem. Commun., 2016, 52, 7668–7671 RSC.
- M. H. Keshavarz, J. Hazard. Mater., 2007, 143, 437–442 CrossRef CAS PubMed.
- M. H. Keshavarz, H. Motamedoshariati, R. Moghayadnia, M. Ghanbarzadeh and J. Azarniamehraban, Propellants, Explos., Pyrotech., 2013, 38, 95–102 CrossRef CAS.
- G. X. Wang, C. H. Shi, X. D. Gong and H. M. Xiao, J. Phys. Chem. A, 2009, 113, 1318–1326 CrossRef CAS PubMed.
- P. Politzer, J. S. Murray, J. M. Seminario, P. Lane, M. E. Grice and M. C. Concha, J. Mol. Struct.: THEOCHEM, 2001, 573, 1–10 CrossRef CAS.
- T. M. Klapötke, Chemistry of High-Energy Materials, Walter de Gruyter GmbH & Co. KG, Berlin, New York, 2011 Search PubMed.
- N. D. H. Gamage, B. Stiasny, J. Stierstorfer, P. D. Martin, T. M. Klapötke and C. H. Winter, Chem.–Eur. J., 2016, 22, 2582–2585 CrossRef CAS PubMed.
- N. D. H. Gamage, B. Stiasny, E. G. Kratz, J. Stierstorfer, P. D. Martin, G. A. Cisneros, T. M. Klapötke and C. H. Winter, Eur. J. Inorg. Chem., 2016, 5036–5043 CrossRef CAS.
- M. H. V. Huynh, M. A. Hiskey, C. J. Pollard, D. P. Montoya, E. L. Hartline and R. Gilardi, J. Energ. Mater., 2004, 22, 217–229 CrossRef CAS.
- D. E. Chavez, M. A. Hiskey and D. L. Naud, Propellants, Explos., Pyrotech., 2004, 29, 209–215 CrossRef CAS.
- V. Thottempudi, H. Gao and J. M. Shreeve, J. Am. Chem. Soc., 2011, 133, 6464–6471 CrossRef CAS PubMed.
- C. Qi, R. Zhang and S. P. Pang, RSC Adv., 2013, 3, 17741–17748 RSC.
- X. Zhao, C. Qi, R. Zhang, S. Zhang, S. Li and S. Pang, J. Mol. Model., 2015, 21, 223 CrossRef PubMed.
- T. M. Klapötke and D. G. Piercey, Inorg. Chem., 2011, 50, 2732–2734 CrossRef PubMed.
- M. J. Frisch, G. W. Trucks, H. B. Schlegel, G. E. Scuseria, M. A. Robb, J. R. Cheeseman, G. Scalmani, V. Barone, G.
A. Petersson, H. Nakatsuji, X. Li, M. Caricato, A. Marenich, J. Bloino, B. G. Janesko, R. Gomperts, B. Mennucci, H. P. Hratchian, J. V. Ortiz, A. F. Izmaylov, J. L. Sonnenberg, D. Williams-Young, F. Ding, F. Lipparini, F. Egidi, J. Goings, B. Peng, A. Petrone, T. Henderson, D. Ranasinghe, V. G. Zakrzewski, J. Gao, N. Rega, G. Zheng, W. Liang, M. Hada, M. Ehara, K. Toyota, R. Fukuda, J. Hasegawa, M. Ishida, T. Nakajima, Y. Honda, O. Kitao, H. Nakai, T. Vreven, K. Throssell, J. A. Montgomery Jr, J. E. Peralta, F. Ogliaro, M. Bearpark, J. J. Heyd, E. Brothers, K. N. Kudin, V. N. Staroverov, T. Keith, R. Kobayashi, J. Normand, K. Raghavachari, A. Rendell, J. C. Burant, S. S. Iyengar, J. Tomasi, M. Cossi, J. M. Millam, M. Klene, C. Adamo, R. Cammi, J. W. Ochterski, R. L. Martin, K. Morokuma, O. Farkas, J. B. Foresman and D. J. Fox, Gaussian 09, Revision E.01, Gaussian, Inc., Wallingford, CT, 2013 Search PubMed.
- T. Lu and F. Chen, J. Comput. Chem., 2012, 33, 580–592 CrossRef CAS PubMed.
- V. D. Ghule, J. Phys. Chem. A, 2012, 116, 9391–9397 CrossRef CAS PubMed.
- S. Deswal, V. D. Ghule, T. Ram Kumar and S. Radhakrishnan, Comput. Theor. Chem., 2015, 1054, 55–62 CrossRef CAS.
- A. Devi, S. Deswal, D. Srinivas and V. D. Ghule, J. Mol. Model., 2015, 21, 298 CrossRef PubMed.
- V. D. Ghule, S. Deswal, A. Devi and T. Ram Kumar, Ind. Eng. Chem. Res., 2016, 55, 875–881 CrossRef CAS.
- A. Devi and V. D. Ghule, Can. J. Chem., 2016, 94, 738–743 CrossRef CAS.
- R. Haiges, G. Bélanger-Chabot, S. M. Kaplan and K. O. Christe, Dalton Trans., 2015, 44, 7586–7594 RSC.
- P. Ravi, S. K. Shee, G. M. Gore, S. P. Tewari and A. K. Sikder, Struct. Chem., 2011, 22, 661–669 CrossRef CAS.
- M. A. M. Rashid, S. G. Cho, T. H. Choi and C. H. Choi, Theor. Chem. Acc., 2015, 134, 126 CrossRef.
- P. Ravi and S. P. Tewari, Struct. Chem., 2012, 23, 487–498 CrossRef CAS.
- T. Wei, W. H. Zhu, X. W. Zhang, Y. F. Li and H. M. Xiao, J. Phys. Chem. A, 2009, 113, 9404–9412 CrossRef CAS PubMed.
- Q. Wu, W. Zhu and H. M. Xiao, J. Chem. Eng. Data, 2013, 58, 2748–2762 CrossRef CAS.
- L. Türker, T. Atalar, S. Gümüş and Y. Ҫamur, J. Hazard. Mater., 2009, 167, 440–448 CrossRef PubMed.
- W. H. Zhu, C. C. Zhang, T. Wei and H. M. Xiao, J. Comput. Chem., 2011, 32, 2298–2312 CrossRef CAS PubMed.
- Y. Pan, W.
H. Zhu and H. M. Xiao, J. Mol. Model., 2012, 18, 3125–3138 CrossRef CAS PubMed.
- D. L. Naud, M. A. Hiskey and H. H. Harry, J. Energ. Mater., 2003, 21, 57–62 CrossRef CAS.
- C. L. Mader, in Organic Energetic Compounds, ed. P. L. Markinas, Nova Science Publishers, Commack, New York, 1996 Search PubMed.
- C. Ye and J. M. Shreeve, J. Chem. Eng. Data, 2008, 53, 520–524 CrossRef CAS.
- B. M. Rice and D. M. Sorescu, J. Phys. Chem. B, 2004, 108, 17730–17739 CrossRef CAS.
- P. Politzer and J. S. Murray, Cent. Eur. J. Energ. Mater., 2014, 11, 459–474 Search PubMed.
- P. Politzer, J. Martinez, J. S. Murray, M. C. Concha and A. Toro-Labbé, Mol. Phys., 2009, 107, 2095–2101 CrossRef CAS.
- M. H. Keshavarz, F. Seif and H. Soury, Propellants, Explos., Pyrotech., 2014, 39, 284–288 CrossRef CAS.
- R. W. Gurney, The Initial Velocities of Fragments from Bombs, Shells and Grenades, Ballistic Research Laboratories, Aberdeen, Maryland, BRL Report No. 405, 1943 Search PubMed.
- M. J. Kamlet and M. Finger, Combust. Flame, 1979, 34, 213–214 CrossRef CAS.
- D. R. Hardesty and J. E. Kennedy, Combust. Flame, 1977, 28, 45–59 CrossRef CAS.
- P. Politzer and J. S. Murray, J. Mol. Model., 2015, 21, 262 CrossRef PubMed.
Footnote |
† Electronic supplementary information (ESI) available. See DOI: 10.1039/c7ra02201h |
|
This journal is © The Royal Society of Chemistry 2017 |