DOI:
10.1039/C7RA02122D
(Paper)
RSC Adv., 2017,
7, 21268-21272
A new mfj-type metal–organic framework constructed from a methoxyl derived V-shaped ligand and its H2, CO2 and CH4 adsorption properties†
Received
20th February 2017
, Accepted 10th April 2017
First published on 13th April 2017
Abstract
Based on a new V-shaped tetracarboxylic ligand with a methoxyl group, a new mfj-type microporous metal–organic framework [Cu6(MBDPB)3(H2O)6]·12DMF·10H2O (NJFU-3, NJFU for Nanjing Forestry University; H4MBDPB for 5-methoxyl-1,3-bis(3,5-dicarboxylphenyl)-benzene) was successfully synthesized and structurally characterized. With moderately high porosity, appropriate pore aperture and unsaturated metal sites, the activated NJFU-3a exhibits high H2 (2.56 wt% at 77 K and 1 bar) and CO2 uptakes (15.8 wt% at 298 K and 1 bar), and a moderately high CH4 storage capacity of 155 cm3 cm−3 at 35 bar and 298 K. Meanwhile, it shows a comparably high delivery amount of 135 cm3 cm−3 when taking 5 bar as a specific lower pressure limit and 65 bar as the upper limit.
Introduction
Metal–organic frameworks (MOFs) or porous coordination polymers have emerged as a new type of porous materials with regular arrays of metal ions, or metal clusters and organic linkers.1–6 Because the pore sizes can be finely tuned and the functional sites can be deliberately immobilized on the pore surfaces, MOFs have unique properties and wide applications in gas storage, molecule separation, chemical sensing, catalysis, and drug delivery. Among them, gas storage concerning H2, CO2 and CH4 is the most investigated in recent years.7–16
Due to their intriguing structures and excellent gas adsorption properties, MOFs constructed from tetracarboxylic ligands have attracted great attention and are widely investigated by many research groups.17–27 NbO-type MOFs, built from linear tetracarboxylic ligands and dicopper paddlewheel clusters, show their advantages in hydrogen adsorption as well as methane storage.10,24 The NOTT-MOFs synthesized by the Schröder group not only exhibit high H2 uptake capacities, but were further proved to be excellent candidates for methane storage. Therefore, based on this type of structure, deeper and thorough tuning towards better performance in gas storage has been reported thereafter. Besides NbO-MOFs, building other types of MOFs from non-linear tetracarboxylic ligands, V-shaped ligands for instance, is another field of endeavour.28–33 These non-NbO-type MOFs also demonstrated their great potential in hydrogen adsorption and methane storage, as illustrated by PCN-12 (ref. 28) and PCN-14.23 However, the dynamic nature of ligands endows these MOFs great structural variability, which causes difficulties in property optimization. Therefore, a stable structural platform for isoreticular optimization is of great importance. PCN-306, as a rare MOF with (3,3,4,4)-connected mfj-type network, represents a suitable structural prototype for further tailoring among MOFs constructed by V-shaped tetracarboxylic ligands.34 Our previous work on NJU-Bai10, an analogue of PCN-306, exhibits high hydrogen uptake and high methane storage property.35
In order to achieve high performance of gas adsorption in MOFs, isorecticular optimization based on a stable structural platform by ligand functionalization is widely applied. Among them, polar functional groups are most preferred.36 Methoxy group, as a common polar functional group, has been proved effective in some cases in the adsorption of gases like CO2 and hydrocarbon.37–39 Inspired by these works, herein we designed and incorporated the bulky methoxy groups (Scheme 1) into the framework of PCN-306 to form an another mfj-type MOF [Cu6(MBDPB)3(H2O)6]·12DMF·10H2O (NJFU-3, NJFU for Nanjing Forestry University; H4MBDPB for 5-methoxyl-1,3-bis(3,5-dicarboxylphenyl)-benzene). Interestingly, the incorporation of methoxy groups was able to improve the gas adsorption property of NJFU-3 not by its polarity, but through optimizing the inner textural property of NJFU-3. As a result, the activated NJFU-3a exhibits a high hydrogen adsorption of 2.56 wt% at 77 K and 1 bar and a high CO2 uptake of 15.8 wt% at 298 K and 1 bar. Meanwhile, it shows a moderately high CH4 storage of 155 cm3 cm−3 at 35 bar and 298 K and a quite large CH4 delivery amount of 135 cm3 cm−3, which indicates it as a potential material for CH4 storage.
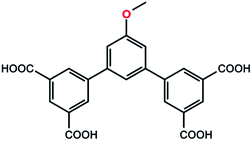 |
| Scheme 1 The ligand of H4MBDPB. | |
Experimental
Materials and methods
All chemical reagents were obtained from commercial sources and, unless otherwise noted, were used as received without further purification. Elemental analyses (C, H, and N) were performed on a Perkin-Elmer 240 analyzer. The IR spectra were recorded in the 400–4000 cm−1 on a Bruker VERTEX 80V spectrometer using KBr pellets. 1H NMR spectra were recorded on a Bruker DRX-500 spectrometer with tetramethylsilane as an internal reference. Thermal gravimetric analyses (TGA) were performed under N2 atmosphere (100 mL min−1) with a heating rate of 5 °C min−1 using a 2960 SDT thermogravimetric analyzer. Powder X-ray diffraction (PXRD) data were collected on a Bruker D8 ADVANCE X-ray diffractometer with Cu/Kα radiation.
Gas sorption measurements
Low pressure gas sorption measurements were conducted using a Micromeritics ASAP 2020 surface area and pore size analyzer up to saturated pressure at different temperatures. High pressure gravimetric CH4 adsorption measurements were performed on an ISOSORP HyGpra + V adsorption analyzer (Rubotherm, Germany) over 0–65 bar range at 298 K. Before the gas sorption measurement, more than 150 mg (about 100 mg for high pressure gas adsorption) as-synthesized samples of NJFU-3 were washed with DMF and methanol, respectively. Fresh anhydrous methanol was then added, and the samples were allowed to soak for 3 days for solvent-exchange. During this period, methanol was refreshed every 8 hours. After then, the sample was charged into a sample tube and activated at 90 °C for 20 hours under vacuum.
X-ray collection and structure determination
Single crystal suitable for X-ray structure determination were selected and sealed in a capillary under a microscope. The X-ray diffraction intensity data were measured on a BRUKER D8 VENTURE PHOTON diffractometer at room temperature using graphite monochromated Mo/Kα radiation (λ = 0.71073 Å). Data reduction was made with the Bruker Saint program. The structures were solved by direct methods and refined with full-matrix least squares technique using the SHELXTL package. Non-hydrogen atoms were refined with anisotropic displacement parameters during the final cycles. Hydrogen atoms were placed in calculated positions with isotropic displacement parameters set to 1.2 × Ueq of the attached atom. The unit cell includes a large region of disordered solvent molecules, which could not be modeled as discrete atomic sites. We employed PLATON/SQUEEZE to calculate the diffraction contribution of the solvent molecules and, thereby, to produce a set of solvent-free diffraction intensities; structures were then refined again using the data generated. Crystal data and refinement conditions are shown in Table S1.† The crystal data for NJFU-3‡ have been deposited in CSD database, and labeled as 1532967.
Synthesis and characterization
The organic linker 5-methoxyl-1,3-bis(3,5-dicarboxylphenyl)-benzene (H4MBDPB) (Scheme 1) was readily synthesized according to ref. 6. 1H NMR (500 MHz, DMSO-d6): 13.31 (bs, 4H), 8.40 (s, 2H), 8.36 (s, 4H), 7.49 (s, 1H), 7.22 (s, 2H), 3.86 (s, 3H). Selected FTIR (neat, cm−1): 3442, 3187, 3085, 2625, 2533, 1701, 1592, 1439, 1389, 1349, 1210, 1139, 1095, 1051, 917, 853, 756, 675. A mixture of H4MBDPB (5.23 mg, 0.012 mmol) and CuCl2·2H2O (13.6 mg, 0.08 mmol) was dissolved in DMF/H2O (2 mL, 5
:
1, v/v) in a screw-capped vial. A concentrated HNO3 (0.05 mL) (65%, aq.) was added to the mixture, the vial was capped and placed in an oven at 65 °C for 2 days. The resulting blue pyramid-shaped crystals were filtered and washed with DMF several times to give NJFU-3 materials. Yield: 72%. Elemental analysis: calcd for activated Cu2(MBDPB), %: C, 49.34; H, 2.15; N, 0; found: C, 49.16; H, 2.07; N, 0.02. Selected FTIR (neat, cm−1): 3443, 3071, 2932, 2866, 1671, 1635, 1587, 1498, 1436, 1410, 1368, 1304, 1255, 1216, 1154, 1093, 1052, 935, 864, 807, 776, 731, 661.
Results and discussion
The structure of NJFU-3 was characterized by single-crystal X-ray diffraction measurement, and the phase purity of the bulk material was independently confirmed by powder X-ray diffraction (PXRD). The similarity of the simulated PXRD pattern from single-crystal data to those for both as-synthesized and activated samples reveals that the single crystal is representative of the pure bulk sample, and the framework is robust and its crystallinity can be retained after the removal of guest molecules and the coordinated water molecules (Fig. S1, ESI†). Single-crystal structure determination reveals that NJFU-3 crystallizes in orthorhombic space group Cmc21, and the symmetric unit consists of one and a half MBDPB-ligand, three crystallographically Cu2+ ions and three coordinated water molecule (Fig. S2†). Two types of MBDPB-ligands existed in the structure possess different dihedral angles between central and terminal benzene rings, one (Fig. 1a) with about 43.4° and 35.6° and the other (Fig. 1b) approximately 63.4° and 63.4°. Each MBDPB-ligand links with four 4-connected dicopper paddlewheel SBUs. By simplifying MBDPB-ligands as two 3-connected nodes and regarding the dicopper paddlewheels SBUs as 4-connected nodes, the whole structure adopts a (3,3,4,4)-c 4-nodal mfj-topology (Fig. 1c). Just as its isoreticular analogues, there are two types of cages existed in the structure: one is surrounded by seven dicopper SBUs and five MBDPB-ligands (Cage A, Fig. 2a), and the other is assembly by eleven dicopper SBUs and eleven MBDPB-ligands (Cage B, Fig. 2b). The cavity inside Cage A and Cage B is with diameters of about 11 Å (excluding van der Waals radii) and 12 Å, respectively. The inner cavity of NJFU-3 endows it high porosity, as calculated using PLATON routine, with the solvent accessible volume of 74.1% in the dehydrated structure of NJFU-3.
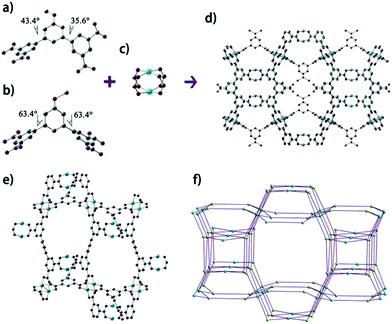 |
| Fig. 1 (a)–(d) are the assembly of two different configurations of ligands and dicopper paddlewheel-clusters into three-dimensional structure; (e) the structure of NJFU-3 viewed along a direction; (f) mfj-type network simplified from NJFU-3. | |
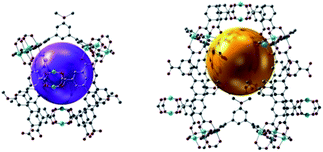 |
| Fig. 2 Two types of cages in NJFU-3: Cage A (purple) and Cage B (orange). | |
Its permanent porosity as well as gas adsorption properties was further investigated. The phase purity of the bulk sample was confirmed by powder X-ray diffraction (PXRD) (Fig. S1, ESI†) and a weight loss of about 41% at 30–300 °C was observed from the TG curve of NJFU-3, which corresponds to 12 DMF and 16·H2O molecules (calcd 41.1%) (Fig. S11, ESI†). The methanol solvent-exchanged sample was degassed under high vacuum at 90 °C for 20 hours to obtain the fully activated sample NJFU-3a. A color change from pale-blue to deep-purple-blue occurred, indicating the exposure of unsaturated metal sites in NJFU-3a. The type-I isotherm of N2 adsorption at 77 K indicates that NJFU-3a is a microporous material. Based on the N2 adsorption isotherm, the Brunauer–Emmett–Teller (BET) surface area and Langmuir surface area of NJFU-3a are calculated to be 2531.1 m2 g−1 and 2671.9 m2 g−1, respectively. The total pore volume obtained from N2 isotherm is 0.94 cm3 g−1.
The moderately high surface area, large pore volume and unsaturated metal sites within the structure of NJFU-3a encouraged us to study its gas adsorption capacity. Low-pressure hydrogen adsorption measurements of NJFU-3a were performed at 77 K and 87 K. The adsorption isotherms are fully reversible (Fig. S5†), and 2.56 wt% of H2 uptake for NJFU-3a was observed under the condition of 77 K and 1 bar, which is a bit higher than that of PCN-306 (ref. 34) (2.50 wt%) (Fig. 3b). Meanwhile, the H2 uptake of NJFU-3a is also higher than that of most of the NOTT-series MOFs constructed by linear tetracarboxylic ligands, such as NOTT-101 (ref. 22) (2.46 wt%), NOTT-109 (ref. 22) (2.28 wt%), NOTT-106 (ref. 22) (2.24 wt%), making it one of the MOFs with highest H2 uptake capacity at 77 K and 1 bar. The CO2 adsorption property of NJFU-3a was also measured at 273 K and 298 K. As shown in Fig. 3c, at 298 K and 1 bar, NJFU-3a can absorb 95.6 cm3 g−1 (or 15.8 wt%) of CO2, which is the highest among all the mfj-MOFs such as PCN-306 (ref. 34) (13.8 wt%) and PCN-308 (ref. 34) (15.4 wt%), and is also higher than some well-known MOFs with open metal sites and/or Lewis basic sites, such as en-CuBTTri40 (5.5 wt%), MOF-505 (ref. 41) (14.4 wt%), Bio-MOF-1 (ref. 42) (14.3 wt%), SNU-21S43 (11.1 wt%), PCN-61 (ref. 14 and 44) (13.9 wt%), PCN-68 (ref. 14 and 44) (4.84 wt%), MIL-102 (ref. 45) (13.6 wt%), MAF-X7 (ref. 46) (5.13 wt%) and NU-100 (ref. 47) (12 wt%). To further understand the interaction between gases molecules and the framework, we calculated the H2 and CO2 adsorption enthalpies of NJFU-3a by virial method, and the zero-coverage values are 6.27 kJ mol−1 and 24.0 kJ mol−1 for H2 and CO2 respectively. Compared with those of the PCN-306, the analogue without methoxyl group, no obvious change was found (H2: 6.37 kJ mol−1; CO2: 23.997 kJ mol−1). Therefore, the existence of methoxyl groups in NJFU-3a does not contribute to the enhancement of interactions between gas molecules and framework, but optimizes the inner textural property that leads to the improvement of gas adsorption property at 1 bar. Furthermore, the CH4 adsorption isotherm of NJFU-3a at 273 K (Fig. S9, ESI†) was also measured to investigate the influence of methoxy group on CO2/CH4 selectivity. By applying the Ideal Adsorbed Solution Theory (IAST), the CO2/CH4 selectivity of NJFU-3a was predicted to be 7.9 (Fig. S9†), which is a bit higher than that of PCN-306 (7.52). Although not significant, such improvement still indicates the positive effect of methoxy groups on the selective adsorption of CO2 in this type of MOF.
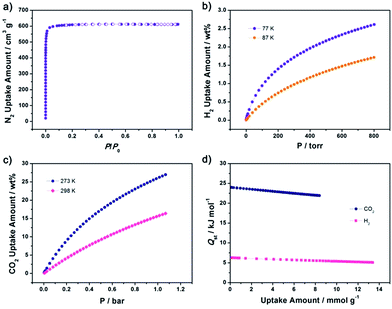 |
| Fig. 3 (a) N2 adsorption isotherm of NJFU-3a at 77 K; (b) H2 adsorption isotherms of NJFU-3a at 77 K and 87 K; (c) CO2 adsorption isotherms of NJFU-3a at 273 K and 298 K; (d) CO2 and H2 adsorption enthalpies of NJFU-3a. | |
We further measured the CH4 storage capacity of NJFU-3a at 298 K. As shown in Fig. 4b, NJFU-3a shows moderately high volumetric methane uptakes of 155 cm3 cm−3 under 35 bar and 298 K, reaching approximately 86% of the DOE standard (180 cm3 cm−3) at 35 bar and room temperature. The methane storage capacity of NJFU-3a is lower than some of the MOFs with extraordinary high methane storage, but higher than most MOF materials under similar condition (MOF-5,8 150 cm3 cm−3; PCN-80,48 118 cm3 cm−3; ZJU-36,49 142 cm3 cm−3; NU-111,7 138 cm3 cm−3; PCN-66,44 136 cm3 cm−3; UiO-66 (Zr),50 146 cm3 cm−3; MOF-205,1 119 cm3 cm−3). When the pressure reaches 65 bar, the methane storage capacity of NJFU-3a increases up to 189 cm3 cm−3. Taking 5 bar as a specific lower pressure limit and 65 bar as the upper limit, the methane delivery amounts of NJFU-3a is 135 cm3 cm−3, that is, a tank filled with NJFU-3a can deliver 51.3% as much fuel as the CNG tank operating at the same lower pressure limit and at 250 bar as the upper limit. Although this value is lower compared to the best performing MOFs, it is still quite comparable with MgMOF-74 (ref. 8) (142 cm3 cm−3), NiMOF-74 (ref. 8) (142 cm3 cm−3), CoMOF-74 (ref. 8) (136 cm3 cm−3), NOTT-100 (ref. 24) (139 cm3 cm−3), and higher than NOTT-119 (ref. 51) (134 cm3 cm−3), MOF-210 (ref. 1) (131 cm3 cm−3), DUT-4 (ref. 52) (124 cm3 cm−3), ZJU-32 (ref. 53) (120 cm3 cm−3), MOF-200 (ref. 1) (106 cm3 cm−3), indicating NJFU-3a as a potential material for CH4 storage.
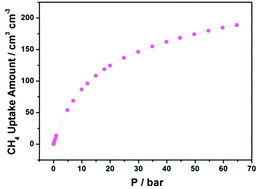 |
| Fig. 4 Volumetric methane uptakes of NJFU-3a at 298 K in high pressure range. | |
Conclusions
In summary, we have developed a new organic linker of the V-shaped tetracarboxylic ligand with methoxyl group, and investigated the gases adsorption of the three-dimensional mfj-type metal–organic framework. The activated NJFU-3a exhibits moderate high porosity with the BET surface area of 2531.1 m2 g−1. Due to the unsaturated metal sites, suitable pore aperture and moderate high permanent porosity, NJFU-3a exhibits a high H2 adsorption of 2.56 wt% at 77 K and 1 bar and a high CO2 uptake of 15.8 wt% at 298 K and 1 bar. Meanwhile, a moderately high CH4 storage of 155 cm3 cm−3 at 35 bar and 298 K and a comparably high delivery amount of 135 cm3 cm−3 was also observed in NJFU-3a when taking 5 bar as a specific lower pressure limit and 65 bar as the upper limit.
Acknowledgements
This work was supported by the Natural Science Fund of Jiangsu Province (Grant No. BK20140968 and BK20150798), the National Natural Science Foundation of China (Grant No. 21501094 and 21601047) and the priority academic program development of Jiangsu higher education institutions.
Notes and references
- H. Furukawa, N. Ko, Y. B. Go, N. Aratani, S. B. Choi, E. Choi, A. O. Yazaydin, R. Q. Snurr, M. O'Keeffe, J. Kim and O. M. Yaghi, Science, 2010, 329, 424–428 CrossRef CAS PubMed.
- S. Bureekaew, S. Horike, M. Higuchi, M. Mizuno, T. Kawamura, D. Tanaka, N. Yanai and S. Kitagawa, Nat. Mater., 2009, 8, 831–836 CrossRef CAS PubMed.
- S. Horike, K. Kishida, Y. Watanabe, Y. Inubushi, D. Umeyama, M. Sugimoto, T. Fukushima, M. Inukai and S. Kitagawa, J. Am. Chem. Soc., 2012, 134, 9852–9855 CrossRef CAS PubMed.
- V. Guillerm, D. Kim, J. F. Eubank, R. Luebke, X. Liu, K. Adil, M. S. Lah and M. Eddaoudi, Chem. Soc. Rev., 2014, 43, 6141–6172 RSC.
- S. Yang, X. Lin, W. Lewis, M. Suyetin, E. Bichoutskaia, J. E. Parker, C. C. Tang, D. R. Allan, P. J. Rizkallah, P. Hubberstey, N. R. Champness, K. M. Thomas, A. J. Blake and M. Schroder, Nat. Mater., 2012, 11, 710–716 CrossRef CAS PubMed.
- C. E. Wilmer, O. K. Farha, T. Yildirim, I. Eryazici, V. Krungleviciute, A. A. Sarjeant, R. Q. Snurr and J. T. Hupp, Energy Environ. Sci., 2013, 6, 1158 CAS.
- Y. Peng, V. Krungleviciute, I. Eryazici, J. T. Hupp, O. K. Farha and T. Yildirim, J. Am. Chem. Soc., 2013, 135, 11887–11894 CrossRef CAS PubMed.
- J. A. Mason, M. Veenstra and J. R. Long, Chem. Sci., 2014, 5, 32–51 RSC.
- O. Shekhah, Y. Belmabkhout, Z. Chen, V. Guillerm, A. Cairns, K. Adil and M. Eddaoudi, Nat. Commun., 2014, 5, 4228 CAS.
- Y. He, W. Zhou, G. Qian and B. Chen, Chem. Soc. Rev., 2014, 43, 5657–5678 RSC.
- S. Ma and H. C. Zhou, Chem. Commun., 2010, 46, 44–53 RSC.
- J. R. Li, J. Yu, W. Lu, L. B. Sun, J. Sculley, P. B. Balbuena and H. C. Zhou, Nat. Commun., 2013, 4, 1538 CrossRef PubMed.
- X.-L. Qi, R.-B. Lin, Q. Chen, J.-B. Lin, J.-P. Zhang and X.-M. Chen, Chem. Sci., 2011, 2, 2214 RSC.
- Z. Zhang, Y. Zhao, Q. Gong, Z. Li and J. Li, Chem. Commun., 2013, 49, 653–661 RSC.
- Z. Lu, J. Bai, C. Hang, F. Meng, W. Liu, Y. Pan and X. You, Chem.–Eur. J., 2016, 22, 6277–6285 CrossRef CAS PubMed.
- L. Du, Z. Lu, K. Zheng, J. Wang, X. Zheng, Y. Pan, X. You and J. Bai, J. Am. Chem. Soc., 2013, 135, 562–565 CrossRef CAS PubMed.
- D. Sun, S. Ma, J. M. Simmons, J. R. Li, D. Yuan and H. C. Zhou, Chem. Commun., 2010, 46, 1329–1331 RSC.
- D. Zhao, D. Yuan, A. Yakovenko and H. C. Zhou, Chem. Commun., 2010, 46, 4196–4198 RSC.
- S. Yang, X. Lin, A. J. Blake, G. S. Walker, P. Hubberstey, N. R. Champness and M. Schroder, Nat. Chem., 2009, 1, 487–493 CrossRef CAS PubMed.
- B. Chen, N. W. Ockwig, A. R. Millward, D. S. Contreras and O. M. Yaghi, Angew. Chem., Int. Ed., 2005, 44, 4745–4749 CrossRef CAS PubMed.
- X. Lin, J. Jia, X. Zhao, K. M. Thomas, A. J. Blake, G. S. Walker, N. R. Champness, P. Hubberstey and M. Schroder, Angew. Chem., Int. Ed., 2006, 45, 7358–7364 CrossRef CAS PubMed.
- X. Lin, I. Telepeni, A. J. Blake, A. Dailly, C. M. Brown, J. M. Simmons, M. Zoppi, G. S. Walker, K. M. Thomas, T. J. Mays, P. Hubberstey, N. R. Champness and M. Schroder, J. Am. Chem. Soc., 2009, 131, 2159–2171 CrossRef CAS PubMed.
- S. Ma, D. Sun, J. M. Simmons, C. D. Collier, D. Yuan and H. C. Zhou, J. Am. Chem. Soc., 2008, 130, 1012–1016 CrossRef CAS PubMed.
- Y. He, W. Zhou, T. Yildirim and B. Chen, Energy Environ. Sci., 2013, 6, 2735 CAS.
- X.-S. Wang, S. Ma, K. Rauch, J. M. Simmons, D. Yuan, X. Wang, T. Yildirim, W. C. Cole, J. J. López, A. d. Meijere and H.-C. Zhou, Chem. Mater., 2008, 20, 3145–3152 CrossRef CAS.
- S. Yang, X. Lin, A. Dailly, A. J. Blake, P. Hubberstey, N. R. Champness and M. Schroder, Chem.–Eur. J., 2009, 15, 4829–4835 CrossRef CAS PubMed.
- A. J. Cairns, J. A. Perman, L. Wojtas, V. Kravtsov, M. H. Alkordi, M. Eddaoudi and M. J. Zaworotko, J. Am. Chem. Soc., 2008, 130, 1560–1561 CrossRef CAS PubMed.
- X. S. Wang, S. Ma, P. M. Forster, D. Yuan, J. Eckert, J. J. Lopez, B. J. Murphy, J. B. Parise and H. C. Zhou, Angew. Chem., Int. Ed., 2008, 47, 7263–7266 CrossRef CAS PubMed.
- X. Liu, M. Park, S. Hong, M. Oh, J. W. Yoon, J. S. Chang and M. S. Lah, Inorg. Chem., 2009, 48, 11507–11509 CrossRef CAS PubMed.
- W. Qiu, J. A. Perman, L. Wojtas, M. Eddaoudi and M. J. Zaworotko, Chem. Commun., 2010, 46, 8734–8736 RSC.
- C. Li, W. Qiu, W. Shi, H. Song, G. Bai, H. He, J. Li and M. J. Zaworotko, CrystEngComm, 2012, 14, 1929 RSC.
- J. J. t. Perry, V. Kravtsov, G. J. McManus and M. J. Zaworotko, J. Am. Chem. Soc., 2007, 129, 10076–10077 CrossRef CAS PubMed.
- D. Wang, B. Liu, S. Yao, T. Wang, G. Li, Q. Huo and Y. Liu, Chem. Commun., 2015, 51, 15287–15289 RSC.
- Y. Liu, J. R. Li, W. M. Verdegaal, T. F. Liu and H. C. Zhou, Chem.–Eur. J., 2013, 19, 5637–5643 CrossRef CAS PubMed.
- Z. Lu, L. Du, K. Tang and J. Bai, Cryst. Growth Des., 2013, 13, 2252–2255 CAS.
- K. Sumida, D. L. Rogow, J. A. Mason, T. M. McDonald, E. D. Bloch, Z. R. Herm, T. H. Bae and J. R. Long, Chem. Rev., 2012, 112, 724–781 CrossRef CAS PubMed.
- X. Duan, Y. Cui, Y. Yang and G. Qian, CrystEngComm, 2017, 19, 1464–1469 RSC.
- H.-M. Wen, G. Chang, B. Li, R.-B. Lin, T.-L. Hu, W. Zhou and B. Chen, Cryst. Growth Des., 2017, 17, 2172–2177 CAS.
- S. Biswas, D. E. P. Vanpoucke, T. Verstraelen, M. Vandichel, S. Couck, K. Leus, Y.-Y. Liu, M. Waroquier, V. Van Speybroeck, J. F. M. Denayer and P. Van Der Voort, J. Phys. Chem. C, 2013, 117, 22784–22796 CAS.
- A. Demessence, D. M. D'Alessandro, M. L. Foo and J. R. Long, J. Am. Chem. Soc., 2009, 131, 8784–8786 CrossRef CAS PubMed.
- A. R. Millward and O. M. Yaghi, J. Am. Chem. Soc., 2005, 127, 17998–17999 CrossRef CAS PubMed.
- J. An, S. J. Geib and N. L. Rosi, J. Am. Chem. Soc., 2009, 131, 8376–8377 CrossRef CAS PubMed.
- T. K. Kim and M. P. Suh, Chem. Commun., 2011, 47, 4258–4260 RSC.
- D. Yuan, D. Zhao, D. Sun and H. C. Zhou, Angew. Chem., Int. Ed., 2010, 49, 5357–5361 CrossRef CAS PubMed.
- S. Surble, F. Millange, C. Serre, T. Duren, M. Latroche, S. Bourrelly, P. L. Llewellyn and G. Ferey, J. Am. Chem. Soc., 2006, 128, 14889–14896 CrossRef CAS PubMed.
- J. B. Lin, W. Xue, J. P. Zhang and X. M. Chen, Chem. Commun., 2011, 47, 926–928 RSC.
- O. K. Farha, A. O. Yazaydin, I. Eryazici, C. D. Malliakas, B. G. Hauser, M. G. Kanatzidis, S. T. Nguyen, R. Q. Snurr and J. T. Hupp, Nat. Chem., 2010, 2, 944–948 CrossRef CAS PubMed.
- W. Lu, D. Yuan, T. A. Makal, J. R. Li and H. C. Zhou, Angew. Chem., Int. Ed., 2012, 51, 1580–1584 CrossRef CAS PubMed.
- G. Q. Kong, Z. D. Han, Y. He, S. Ou, W. Zhou, T. Yildirim, R. Krishna, C. Zou, B. Chen and C. D. Wu, Chem.–Eur. J., 2013, 19, 14886–14894 CrossRef CAS PubMed.
- A. D. Wiersum, J.-S. Chang, C. Serre and P. L. Llewellyn, Langmuir, 2013, 29, 3301–3309 CrossRef CAS PubMed.
- Y. Yan, S. Yang, A. J. Blake, W. Lewis, E. Poirier, S. A. Barnett, N. R. Champness and M. Schroder, Chem. Commun., 2011, 47, 9995–9997 RSC.
- I. Senkovska, F. Hoffmann, M. Fröba, J. Getzschmann, W. Böhlmann and S. Kaskel, Microporous Mesoporous Mater., 2009, 122, 93–98 CrossRef CAS.
- J. Cai, X. Rao, Y. He, J. Yu, C. Wu, W. Zhou, T. Yildirim, B. Chen and G. Qian, Chem. Commun., 2014, 50, 1552–1554 RSC.
Footnotes |
† Electronic supplementary information (ESI) available: Experimental details, PXRD patterns, heat of adsorption. CCDC 1532967. For ESI and crystallographic data in CIF or other electronic format see DOI: 10.1039/c7ra02122d |
‡ Crystal data for NJFU-3: C69H36Cu6O33, M = 1774.28, orthorhombic, Cmc21, a = 24.742(3) Å, b = 33.475(3) Å, c = 18.4621(19) Å, α = β = γ = 90°, V = 15 291 (3) Å3, Z = 4, Dc = 0.771 g cm−3, GOF = 0.984 based on F2, final R1 = 0.0729, wR2 = 0.1842 [for 18 616 data I > 2σ(I)]; data for structure was treated with squeeze. |
|
This journal is © The Royal Society of Chemistry 2017 |
Click here to see how this site uses Cookies. View our privacy policy here.