DOI:
10.1039/C7RA01883E
(Paper)
RSC Adv., 2017,
7, 24453-24461
Facile synthesis of Fe3O4/MIL-101 nanocomposite as an efficient heterogeneous catalyst for degradation of pollutants in Fenton-like system
Received
15th February 2017
, Accepted 27th April 2017
First published on 5th May 2017
Abstract
An active Fe3O4/MIL-101 hybrid material was prepared via a simple in situ solvothermal method and characterized as a heterogeneous Fenton-like catalyst for Rhodamine B (RhB) degradation. The Fe3O4/MIL-101 exhibited enhanced catalytic performance for RhB degradation in a neutral solution. Under optimized conditions, almost 100% removal of 10 mg L−1 RhB was achieved in 30 min using 0.5 g L−1 Fe3O4/MIL-101 and 20 mM H2O2 at initial pH 7. The reusability of Fe3O4/MIL-101 was also investigated after four runs. On the basis of the characterization of the catalyst, the results of metal leaching, the effects of radical scavengers, and hydroxyl radical (˙OH) determination, it was concluded that RhB is decomposed mainly by the attack of the hydroxyl radical (˙OH), generated by the reaction of Fe2+ species with H2O2. MIL-101 showed a significant synergistic effect with Fe3O4, in which it played the role of a solid-acid catalyst with Lewis acid sites on its surface, ensuring that a Fenton-like reaction occurred in the neutral solution.
1. Introduction
The pollution of water resources by refractory organic compounds has become a serious environmental problem that has attracted much attention.1 However, conventional wastewater treatment technology is not very effective for the degradation of refractory organic pollutants due to the stable structures of these organic compounds.2,3 Among the available water remediation technologies, advanced oxidation processes (AOPs) are regarded as effective technologies for the degradation of hazardous organic pollutants in wastewater.4 The Fenton oxidation process, one of the AOPs, seems to be promising due to its low cost and environmental benignity. However, in contrast with homogeneous Fenton oxidation, heterogeneous Fenton-like oxidation has received extensive attention, as it can overcome the drawbacks of homogeneous Fenton oxidation by working in mild conditions and producing almost no byproduct sludge after reaction.5
In recent years, metal–organic frameworks (MOFs) have attracted great attention because of their salient features, including a porous structure, extremely high surface area, tunable pore size, and functionality.6–9 These unique properties endow MOFs with wide applications in the areas of catalysis,10 molecular separations,11 sensing,12,13 and gas adsorption,14 etc. Moreover, MOF materials also exhibit tremendous potential in the degradation of pollutants in the wastewater. For example, Jiang et al.15 presented an approach to construct a photocatalytically active MOF as a catalyst for RhB degradation under visible light. Zhao et al.16 reported that core–shell Fe3O4@MOFs could be used as a visible-light-driven catalyst for the degradation of dye pollutant with H2O2 as an electron acceptor. Jiang et al.17 observed that MIL-53(Fe) could completely decompose RhB in the presence of H2O2 under visible light irradiation. Nevertheless, among all of them, the introduction of external energy is a vital factor to ensure their high catalytic activity, which will hamper their practical application caused by the large cost. To the best of our knowledge, excellent MOF-based Fenton-like catalyst without the aid of external energy has been rarely reported. Therefore, the goal of the present work is to provide a simply synthetic MOF-based Fenton-like catalyst which exhibits powerful efficiency for degradation of RhB without additional energy input and works in mild operation condition with wide application.
MIL-101(Fe) is a typical MOF material with an extremely large surface area and numerous transition metal sites.18 Additionally, it has a zeotype crystal structure with high resistance to air, water and common solvents.19 Therefore, MIL-101(Fe) was selected as the object of study in our research. Fe3O4 nanoparticles were used in the synthetic process and functionalized MIL-101(Fe) as a superparamagnetic model for degradation of RhB and magnetic recovery.
The proposed synthetic procedure involved in situ fabrication of MIL-101(Fe) in the presence of Fe3O4 nanoparticles, using a solvothermal method. The electrostatic interaction between Fe3O4 and metal ions induced chemical stabilization of magnetic nanoparticles. Thus, MOF crystals were uniformly enclosed by Fe3O4 to form a homogeneous magnetic product, identified as a Fe3O4/MIL-101. The physical and chemical characterization of Fe3O4/MIL-101 was conducted, and the applicability of this hybrid material in heterogeneous Fenton-like reaction was evaluated in view of the effect of the various processes, material stability, degradation mechanism, and the roles of Fe3O4 and MIL-101(Fe).
2. Materials and methods
2.1. Reagents and materials
Rhodamine B, tert-butanol and coumarin were obtained from Sigma-Aldrich, Taiwan. FeCl3·6H2O, FeCl2·4H2O and terephthalic acid (H2BDC) were purchased from Aladdin, China. The organic solvents used (viz., ethanol, and dimethylformamide (DMF)) were obtained from Sinopharm, China. All reagents were of analytical grade without further purification.
2.2. Preparation of catalysts
First, Fe3O4 nanoparticles were synthesized via a chemical co-precipitation method.20 Briefly, FeCl2·4H2O (2.0 g) and FeCl3·6H2O (5.2 g) were dissolved in 25 mL deionized water, followed by the addition of 0.85 mL concentrated hydrochloric acid. The resulting solution was added into a 500 mL three-necked round flask, and then 250 mL of NaOH solution (1.5 M) was dropwise into the above solution under vigorous non-magnetic agitation and N2 protection at 80 °C. Afterwards, the obtained Fe3O4 suspension was aged for 30 min and cooled down to the room temperature. Finally, the black precipitate was isolated from the supernatant by magnetic decantation and washed with deionized water several times until the pH of the washings approached a neutral value.
For the preparation of Fe3O4/MIL-101, the freshly wet magnetic Fe3O4 particles (equal to 40 mg of dried Fe3O4) were dispersed in 20 mL of FeCl3·6H2O (2700 mg) DMF solution with ultrasonication for 10 min, and then in 40 mL of H2BDC (824 mg) DMF solution with ultrasonication for another 30 min. The mixture was transferred into a Teflon-lined autoclave bomb and heated at 110 °C for 20 h. Thereafter, the brown solid was separated from the reaction medium with an external magnet, washed several times, and dried overnight in vacuum at 50 °C. The resulting product was identified as Fe3O4/MIL-101. MIL-101 was synthesized as in the above procedure without adding Fe3O4.
2.3. Catalyst characterization
The powder X-ray diffraction (XRD) patterns of samples were collected using a diffractometer (X'Pert Pro, Holland) with Cu Kα radiation (40 kV, 40 mA). The microscopic feature of the samples was observed using a transmission electron microscope (TEM) (JEM 2010, JEOL, Japan) operated at 200 kV. A Fourier Translation Infrared Spectroscopy (FT-IR) Spectrometer (Nicolet Nexus 670, America) was used to record the spectra of the samples in a range from 500 nm to 2500 nm. Nitrogen adsorption/desorption was analysed using a Micromeritics ASAP 2010 sorption analyser. The functional groups and the related oxidation state of the surface of the materials were analysed by X-ray photoelectron spectroscopy (XPS) on a PHI 5000 Versaprobe spectrometer equipped with a rotating Al anode generating Al Kα X-ray radiation at 1486.6 eV. The X-ray beam was monochromatized using seven crystals that were mounted on three Rowland circles. The zeta potentials of samples were measured by micro-electrophoresis apparatus (JS94J, Shanghai).
2.4. Experimental procedures
Batch trials were performed in glass beakers (150 mL) with mechanical stirring in the dark. The reaction suspension was prepared by adding the required amount of catalyst into 100 mL of a 10 mg L−1 RhB solution that had been adjusted to the desired pH value by H2SO4 or NaOH. The required amount of H2O2 was added to the solution to initiate the reaction. Then, the samples were extracted at set intervals using a 5 mL syringe and filtered immediately through a 0.22 μm filter film prior to analysis. To evaluate the contribution of homogeneous removal by the leaching ions, experiments were carried out as follows: after mixing Fe3O4/MIL-101 with deionized water (pH 7) for 30 min and removing the composite by filtering, the required amount of RhB was added into the filtrate. To test the stability of Fe3O4/MIL-101, the catalyst was gathered, washed, dried under vacuum, and reused in a fresh solution of RhB and H2O2 several times. All experiments were conducted in duplicate, and the relative error was less than 5%. Unless specifically noted, each experiment was carried out at an ambient temperature of 25 ± 1 °C and was exposed to air.
2.5. Sample analysis
The pH values were determined by a pH-meter (PHS-3C). The concentration of RhB was monitored at 554 nm with a dual-beam UV-visible spectrophotometer (TU-1901). The concentration of ferrous ions was measured colorimetrically with 1,10-phenanthroline at 510 nm on a UV/vis spectrophotometer (Lambda 25, PerkinElmer), as described previously.21,22 ˙OH produced in the Fenton-like system was estimated by employing coumarin (1.0 mM) as a selective trap for ˙OH. The fluorescence intensity of 7-hydroxycoumarin was determined by a fluorescence spectrophotometer (F97PRO, Lengguang Tech.) at λexcitation = 322 nm and λemission = 455 nm.23,24
3. Results and discussion
3.1. Characterization of catalysts
In this study, Fe3O4/MIL-101 with different Fe3O4 loading amounts (e.g., 40 mg, 80 mg, 120 mg, and 200 mg) were prepared for characterization and performance measurements. The smallest Fe3O4 loading amounts was determined to be 40 mg mainly because when any smaller loading amount of the composite was used, some free MIL-101 crystals remained in the solution even after a magnetic field was applied to the side wall of the vessel. Wide-angle XRD was used to identify the crystalline phase of the samples. Fig. 1 shows the XRD pattern of Fe3O4, MIL-101 and Fe3O4/MIL-101 with the least amount of Fe3O4 (40 mg). The powder XRD patterns of MIL-101 were in accordance with the literature.18 Five characteristic peaks for Fe3O4 and two characteristic peaks for MIL-101 were preserved in the pattern of Fe3O4/MIL-101, revealing that this hybrid material was composed of Fe3O4 and MIL-101.
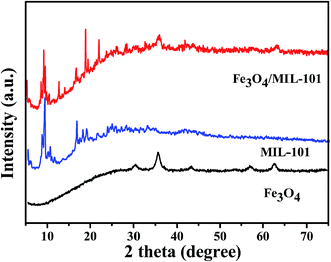 |
| Fig. 1 XRD patterns of Fe3O4, MIL-101, and Fe3O4/MIL-101 with different Fe3O4 loading amounts of 40 mg, 80 mg, 120 mg and 200 mg. | |
Fig. 2 shows the morphologies of Fe3O4/MIL-101 with Fe3O4 loading amount of 40 mg. It is apparent from the SEM images (Fig. 2a) that MIL-101 exhibited a uniform size and the Fe3O4 nanoparticles attached to the external surface of the MIL-101 crystals were uniformly distributed, which is a result of the electrostatic interaction between the positively charged MIL-101 and negatively charged Fe3O4 (provided by the –OH on its surface). This interaction was supported by the zeta potentials of Fe3O4 and MOF.25 Additionally, Fig. 2b illustrates that the nanoparticles possess a uniform polyhedral morphology. From a suitable perspective, MIL-101 presented a perfectly analogous hexagonal morphology in the TEM image, with many nanoparticles of Fe3O4 enclosing all loading amounts (Fig. 2c). The HRTEM image in Fig. 2d shows that the lattice fringe spacing of the nanoparticles was approximately 0.25 nm, which can be assigned to the (311) reflection of Fe3O4. More detailed information can be obtained from the TEM images (Fig. 3). The Fe3O4 particles were sparse when the loading amount was 40 mg, and the TEM images show clearly increasing Fe3O4 content on the surface of the hybrid material with increasing amounts of Fe3O4. This is consistent with the synthetic process with different loading amounts.
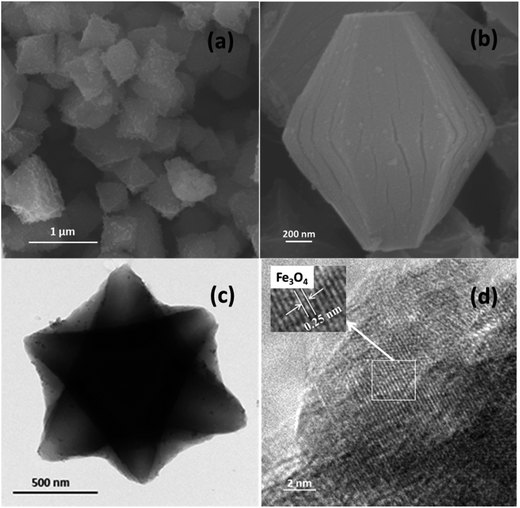 |
| Fig. 2 Fe3O4/MIL-101 composite with Fe3O4 loading amount of 40 mg SEM images (a) and (b), TEM image (c) and HRTEM image (d). | |
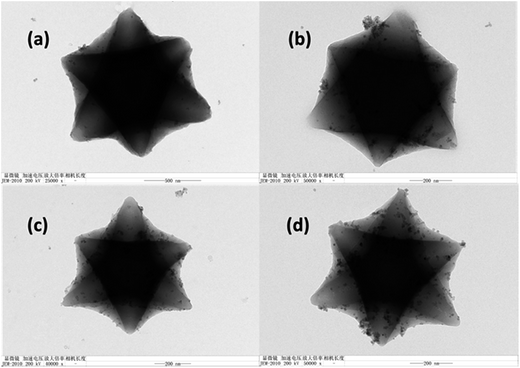 |
| Fig. 3 TEM images of Fe3O4/MIL-101 composites with Fe3O4 loading amounts of 40 mg (a), 80 mg (b), 120 mg (c) and 200 mg (d). | |
Fig. 4 shows the FT-IR spectra of Fe3O4, MIL-101 and Fe3O4/MIL-101 with the least amount of Fe3O4 (40 mg). The FT-IR spectrum for Fe3O4 shows a typical band at 3415 cm−1, which is attributed to O–H stretching vibrations. For MIL-101, the peak at 741 cm−1 is assigned to the out-of-plane bending vibration of C–H in the benzene ring of H2BDC. The bands at 1681 and 1506 cm−1 are assigned to the asymmetric stretching of carboxyl groups in H2BDC, whereas the band at 1385 cm−1 is assigned to the symmetric stretching of carboxyl groups in H2BDC.26 No special differences between MIL-101 and Fe3O4/MIL-101 were observed, and all the bands demonstrate the dominance of the properties of MIL-101 in the hybrid material.
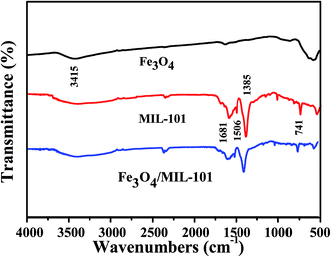 |
| Fig. 4 FT-IR spectra of Fe3O4, MIL-101, and Fe3O4/MIL-101 with Fe3O4 loading amount of 40 mg. | |
The textural properties of samples were characterized by nitrogen adsorption isotherms. MIL-101 powder has an extremely high BET surface area and porous volume (1987.05 m2 g−1 and 0.624 cm3 g−1, respectively). Compared with MIL-101 powder, the surface area of Fe3O4/MIL-101 powder with Fe3O4 loading amounts of 40 mg was observed to be smaller by approximately 15.2% (Table 1). This may be due to the loading of Fe3O4 in the MIL-101 material as well as the activating role of the Fe(III) salt. On the other hand, the insignificant change in porosity of the Fe3O4/MIL-101 and MIL-101 could result from the small loading amount of Fe3O4, which ensures that Fe3O4/MIL-101 remains higher surface area and porous volume. The nitrogen adsorption–desorption isotherms exhibited a typical type-I form (Fig. 5), which indicates that the Fe3O4/MIL-101 composite has a typical microporous structure similar to that of pure MIL-101.
Table 1 BET properties of Fe3O4/MIL-101
Sample |
Fe3O4 (mg) |
SBET (m2 g−1) |
Pore size (nm) |
Pore volume (cm3 g−1) |
MIL-101 |
0 |
1987.05 |
2.68 |
0.624 |
Fe3O4/MIL-101 |
40 |
1685.89 |
2.73 |
0.632 |
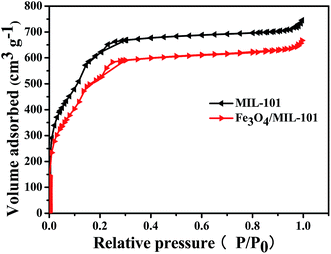 |
| Fig. 5 Nitrogen adsorption–desorption isotherms for pure MIL-101 and Fe3O4/MIL-101 with Fe3O4 loading amount of 40 mg. | |
3.2. Catalytic activity of Fe3O4/MIL-101
Control experiments were conducted to compare the removal efficiencies at initial pH 7 with RhB concentration of 10 mg L−1. Fig. 6a shows the catalytic activity of Fe3O4/MIL-101 with different Fe3O4 loading amounts (40 mg, 80 mg, 120 mg and 200 mg). It is clearly seen that with decreasing Fe3O4 loading, the hybrid material showed gradual improvement in catalytic activity, indicating that MIL-101 crystals played a key role in the degradation of RhB. The high porosity and the presence of a large amount of oxygen groups on the MOF surfaces resulted in their high surface area and moderate polarity, thus facilitating effective interaction with RhB and accelerating the catalytic process. Fe3O4/MIL-101 with the least Fe3O4 loading amount of 40 mg provided the highest catalytic capacity. Therefore, it was employed in the following studies.
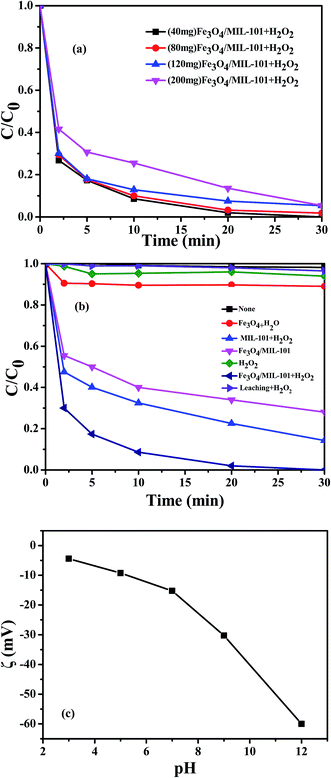 |
| Fig. 6 (a) Removal of RhB with different loading amounts of Fe3O4 as catalysts at initial pH 7; (b) removal of RhB under different reaction conditions at initial pH 7; (c) the zeta potential of Fe3O4/MIL-101 at different pH values. Conditions: [catalyst] = 0.5 g L−1, [RhB] = 10 mg L−1, [H2O2] = 20 mM. | |
To clarify the excellent performance of Fe3O4/MIL-101, MIL-101 and Fe3O4 were also prepared and examined under same experimental conditions. As seen from Fig. 6b, no noticeable removal of RhB was observed in the presence of RhB alone. H2O2 only led to a slight removal of RhB within 30 min. In the leached metal–H2O2 system, the oxidation efficiency was greatly limited, with approximately 2% of RhB degraded after 30 min. This indicates that nearly all of reactions occur on the surface of catalyst, thus proving the stability of Fe3O4/MIL-10. In the presence of H2O2, the removal of RhB using Fe3O4/MIL-101 as the heterogeneous Fenton-like catalyst was notably higher than that for pure MIL-101 and Fe3O4, implying that the catalytic activity was enhanced by the introduction of MIL-101 and Fe3O4. There is a synergistic effect in the hybrid material, which enhances the relative rates of mass transfer to reactive sites and subsequent chemical reaction at the reactive sites. With 0.5 g L−1 Fe3O4/MIL-101 only, approximately 65% removal was observed, mainly due to surface adsorption of Fe3O4/MIL-101. Furthermore, the effect of surface adsorption was investigated. The adsorption of RhB is sensitive with surface charge of Fe3O4/MIL-101. Therefore, the zeta potential of Fe3O4/MIL-101 was provided to better understand the adsorption behavior of RhB in solution. As seen from Fig. 6c, the absolute values of zeta potential increased obviously with the increase of initial pH, which indicates that Fe3O4/MIL-101 has negative charge in solution at initial pH 3, 5, 7, 9 and 12, and the negative charges of Fe3O4/MIL-101 particles increase with the increase of pH. As we know, RhB is a kind of cationic dyes. Thus, there are electrostatic interaction between Fe3O4/MIL-101 with negative charge and RhB molecule with positive charge, and the adsorption properties of Fe3O4/MIL-101 increased gradually with the increase of zeta potential. Several experiments were performed to explore the interaction between catalysis and adsorption. Desorption was almost completely achieved via ultrasonic treatment of the used hybrid material for 30 min with using ethanol as solvent. The result was confirmed by desorbing RhB from Fe3O4/MIL-101 after the surface adsorption reaction, resulting in nearly 100% desorption. However, after the recovery of used Fe3O4/MIL-101 from the Fenton-like system, RhB desorption percentage was only approximately 14% in the same experiment conditions. All surface adsorption–desorption experiments demonstrate that Fe3O4/MIL-101 has a strong adsorption property. However, in the presence of H2O2, catalytic action plays an absolutely leading role rather than adsorption. Also, it is speculated that the adsorption action of Fe3O4/MIL-101 may facilitate the process of catalysis.
3.3. Effect of pH
It is well-known that the performance of the Fenton reaction is strongly correlated to the solution pH.27,28 Therefore, the effect of the initial pH value on the degradation of RhB with Fe3O4/MIL-101 catalyst was determined as presented in Fig. 7. Approximately 65% and 63% of RhB were removed after 30 min of reaction at initial pH 3 and 12, respectively. The amount removed increased dramatically, reaching almost 100% at initial pH 5, 7, and 9. Thus, the effect of initial pH on the degradation of phenol is clear. The removal rate achieved highest efficiency when the initial RhB solution had a nearly neutral pH. In contrast to the general Fenton reaction activated in an acidic environment, Fe3O4/MIL-101 shows extraordinary performance as an efficient Fenton-like heterogeneous catalyst in a steerable neutral solution of the pollutant, which is a great advantage for improve efficiency and cost saving. This may be related to the function of solid-acid catalyst of the MIL-101, which will be introduced in detail below. On the other hand, the amount removed decreased dramatically at initial pH 3 and 12, which is reasonable because compared to neutral environments, MOF materials lack stability in acidic and alkaline conditions, thus hindering the process of catalysis.
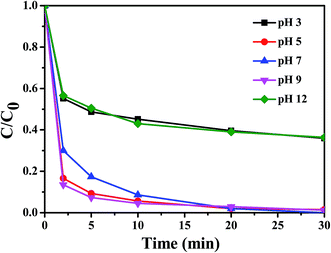 |
| Fig. 7 Influence of initial pH on the degradation of RhB in the Fe3O4/MIL-101-H2O2 system. Conditions: [Fe3O4/MIL-101] = 0.5 g L−1, [RhB] = 10 mg L−1, [H2O2] = 20 mM. | |
3.4. Stability of Fe3O4/MIL-101
The stability of the catalyst was evaluated by reusing it in four successive experiments under same reaction conditions. When the initial solution pH was 7, approximately 60% of RhB could still be removed in the fourth run after 30 min (data not shown). Additionally, as seen from Fig. 8a, nearly 100% RhB was further removed in each cycle if the reaction time was extended to 60 min, indicating the good stability and long lifetime of the composite. The concentration of dissolved Fe in the solution was investigated during the Fenton-like oxidation of RhB (Fig. 8b). The concentration of ferrous ion increased and reached a maximum of approximately 9 μg L−1 at 30 min, when almost complete removal of RhB occurred. Following this, the Fe2+ concentration decreased to approximately 2 μg L−1 at 60 min, similar to the results reported in earlier studies.29,30 In the ascending interval, oxidation of the catalyst by H2O2 may have released ferrous ions into solution, generating ˙OH. After RhB was almost completely removed, the decrease in concentration probably resulted from the oxidation of the dissolved ferrous ions to ferric ions by the remaining oxidants.31 Thus, the increase of total dissolved iron may be a result of the leaching of ferrous and ferric ions from Fe3O4/MIL-101, as well as the oxidation of ferrous ions in solution. The loss of iron amounted to 22.2 μg L−1, negligible compared with the 0.5 g L−1 of catalyst used. To further check the structural stability of the catalyst, the phase structure and molecular structure of the Fe3O4/MIL-101 after four times of repeated use were recorded by XRD (Fig. 8c) and FT-IR spectra (Fig. 8d). The almost identical XRD patterns and FT-IR spectra before and after the Fenton-like reactions illustrates that the chemical and crystal structure of the catalyst are almost unchanged after four repeated reactions. These results demonstrate the high long-term stability of our Fe3O4/MIL-101 Fenton-like catalyst.
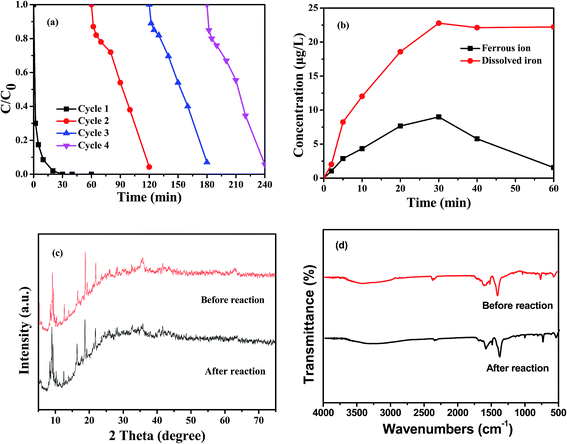 |
| Fig. 8 (a) Catalytic property of Fe3O4/MIL-101 during repeated use at neutral conditions; (b) variation of the concentration of dissolved iron and ferrous ion in solution during RhB degradation; (c) XRD patterns of the Fe3O4/MIL-101 before and after Fenton-like reaction; (d) FT-IR spectra of the Fe3O4/MIL-101 before and after Fenton-like reaction. Conditions: [Fe3O4/MIL-101] = 0.5 g L−1, [RhB] = 10 mg L−1, [H2O2] = 20 mM, initial pH 7. | |
3.5. Mechanistic study
It is well-known that tert-butanol (TBA) is mainly used as a quenching agent for ˙OH because of its high reactivity (k˙OH: 3.8–7.6 × 108 M−1 s−1).32–34 The actual reactive specie mediating the process was identified by determining the influence of TBA as a radical scavenger on the degradation of RhB, as shown in Fig. 9a. When no quenching agent was added, approximately 100% RhB was degraded in 30 min. The addition of 100 mM TBA resulted in the decrease of RhB removal (in 30 min) to 95%, with almost no inhibition, because of a small amount of added radical scavenger. However, the amount removed decreased dramatically, reaching only 15%, with 1000 mM TBA. Thereafter, there was a second apparent jump when the added TBA was approximately 2000 mM, with nearly 100% inhibition. Reasonably, the inhibition was enhanced with the increase in the concentration of TBA, indicating that ˙OH radicals were involved in the oxidation process. Moreover, excess TBA in solution not only scavenged all the produced ˙OH but also resulted in competitive adsorption against RhB due to the interaction of functional groups between TBA and Fe3O4/MIL-10, so that the inhibition effect reached almost 100%.
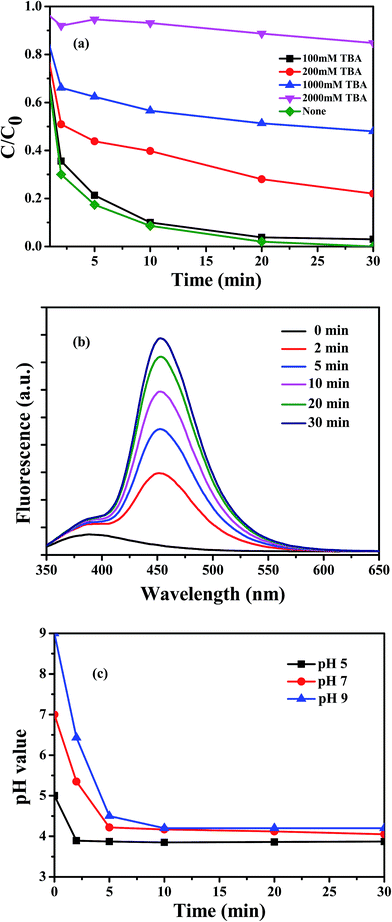 |
| Fig. 9 (a) Effects of TBA on the degradation of RhB at initial pH 7; (b) fluorescence intensity of 7-hydroxycoumarin produced in the Fenton-like reaction at initial pH 7; (c) pH variation during the reaction in the Fe3O4/MIL-101–H2O2 system at different initial pH. Conditions: [Fe3O4/MIL-101] = 0.5 g L−1, [RhB] = 10 mg L−1, [H2O2] = 20 mM. | |
The results of the radical scavenger experiments are validated by the fluorescence spectra in Fig. 9b. We observed that in the Fe3O4/MIL-101–H2O2–coumarin system, the oxidation of coumarin by the generated ˙OH radicals resulted in a rapid increase of the fluorescence intensity of the product 7-hydroxycoumarin with increasing reaction time. Coumarin did not easily react with H2O2 in the absence of the catalyst. The fluorescence intensity in the system gradually increased, indicating that H2O2 activated by Fe3O4/MIL-101 produced strong oxidizing ˙OH radical in the Fenton-like system.
The Lewis acid catalytic properties of MOFs have already been demonstrated for many reactions, including cyanosilylation of carbonyl compounds,35 epoxide methanolysis,36 isomerizations of α-pinene oxide and citronella,37 alkene cyclopropanation,38 etc. The Fe3O4/MIL-101 fabricated in this study also has a solid-acid catalytic property, which stems from the creation of a coordination vacancy upon unsaturated coordination of Fe ions in the MIL-101 structure. To better understand the interaction between the Lewis acid character and the neutral reaction environment, the change of solution pH value was monitored as a function of time at different initial pH (Fig. 9c). Obviously, the pH values remained unchanged after 5 min at approximately 3.8, 4.1, and 4.2 when the initial pH values were 5, 7, and 9, respectively. The change of pH values was probably due to the effect of the Lewis acid sites in the structure of MIL-101, in which the incompletely coordinated Fe ions could coordinate with H2O and supply H+ in the initially neutral Fenton-like system, as shown in eqn (1).
On the basis of the above information, a possible reaction mechanism for H2O2 activated by Fe3O4/MIL-101 under neutral conditions is proposed in Fig. 10. First of all, the Lewis acid sites in the structure of the MIL-101 provide H+ in initially neutral aqueous solution (eqn (1)). As reported by other researchers,39–41 the initially generated Fe2+ species can react with H2O2 to generate ˙OH (eqn (2)), where Fe2+ stands for Fe(II) sites on the catalyst surface. Additional Fe2+ species are produced through the reactions of the formed Fe3+ species with H2O2 (eqn (3)) and HO2˙ (eqn (4)). This is further accompanied by competitive reactions that can negatively affect the oxidation process, as shown in eqn (5)–(10). Finally, RhB is broken down, mainly by hydroxyl radical on the surface of catalyst (eqn (11)). It is worth mentioning that all the reactions occur on the surface of the catalyst, in accordance with the result of the leaching experiment, as shown in Fig. 6b.
|
 | (1) |
|
Fe2+ + H2O2 + H+ → Fe3+ + ˙OH + H2O
| (2) |
|
Fe3+ + H2O2 → Fe2+ + HO2˙ + H+
| (3) |
|
Fe3+ + HO2˙ → Fe2+ + O2 + H+
| (4) |
|
˙OH + Fe2+ → OH− + Fe3+
| (5) |
|
˙OH + H2O2 → H2O + HO2˙
| (6) |
|
Fe2+ + HO2˙ → Fe3+ + HOO−
| (7) |
|
HO2˙ + HO2˙ → H2O2 + O2
| (8) |
|
˙OH + HO2˙(O2˙−) → O2 + H2O(+OH−)
| (9) |
|
˙OH + RhB → degraded products
| (11) |
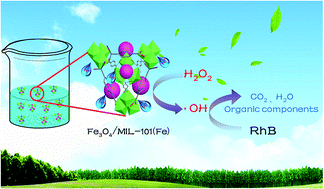 |
| Fig. 10 Mechanisms for RhB degradation by Fe3O4MIL-101–H2O2 system. | |
4. Conclusions
In this study, Fe3O4/MIL-101 was successfully synthesized via a simple solvothermal process and found to be highly efficient and environmentally benign for the heterogeneous activation of H2O2 in Fenton-like system without additional energy input. The prepared Fe3O4/MIL-101 exhibited enhanced catalytic performance for degradation of RhB in a neutral Fenton-like solution, which was superior to those of pure Fe3O4 and MIL-101. This enhancement of the catalytic performance has been demonstrated due to the significant synergistic effect between Fe3O4 and MIL-101, in which MIL-101 played the role of a solid-acid catalyst with Lewis acid sites on its surface. Compared with the traditional Fenton systems which work under strongly acidic environment, the neutral operation condition of Fe3O4/MIL-101 is more suitable for practical water treatment. The as-prepared Fe3O4/MIL-101 sample exhibited magnetic property for easy separation and high long-term stability on the degradation of the organic pollutants. The results from this study shed light on the application of MOF-based solid-acid catalyst in environmental remediation field with mild conditions and low-energy treatment.
Acknowledgements
This work was supported by the National Science and Technology Major Project (No. 2016ZX05040003); and the Postgraduate Innovation Project of China University of Petroleum (No. YCXJ2016035).
References
- Z. Ma, X. Wei, S. Xing and J. Li, Catal. Commun., 2015, 67, 68–71 CrossRef CAS.
- O. A. Yildirim, H. Arslan and S. Sönmezoğlu, Appl. Surf. Sci., 2016, 390, 111–121 CrossRef.
- B. Li, Y. Zhang, X. Zhou, Z. Liu, Q. Liu and X. Li, J. Alloys Compd., 2016, 673, 265–271 CrossRef CAS.
- W. Liu, C. Fang, Y. Huang, L. Ai, F. Yang, Z. Wang and J. Liu, RSC Adv., 2016, 6, 93558–93563 RSC.
- J. Fang, X. Huang, Q. Zhang, J. Chen and X. Wang, Appl. Surf. Sci., 2016, 360, 994–998 CrossRef CAS.
- Q.-L. Zhu and Q. Xu, Chem. Soc. Rev., 2014, 43, 5468–5512 RSC.
- M. Drobek, J.-H. Kim, M. Bechelany, C. Vallicari, A. Julbe and S. S. Kim, ACS Appl. Mater. Interfaces, 2016, 8, 8323–8328 CAS.
- S. Zhang, Z. Jiao and W. Yao, J. Chromatogr. A, 2014, 1371, 74–81 CrossRef CAS PubMed.
- X. Zhang, B. Li, H. Ma, L. Zhang and H. Zhao, ACS Appl. Mater. Interfaces, 2016, 8, 17389–17394 CAS.
- M. Zhao, K. Deng, L. He, Y. Liu, G. Li, H. Zhao and Z. Tang, J. Am. Chem. Soc., 2014, 136, 1738–1741 CrossRef CAS PubMed.
- A. Kertik, A. L. Khan and I. F. J. Vankelecom, RSC Adv., 2016, 6, 114505–114512 RSC.
- X.-L. Huang, L. Liu, M.-L. Gao and Z.-B. Han, RSC Adv., 2016, 6, 87945–87949 RSC.
- J. A. Gustafson and C. E. Wilmer, J. Phys. Chem. C, 2017, 121, 6033–6038 CAS.
- F. Rezaei, S. Lawson, H. Hosseini, H. Thakkar, A. Hajari, S. Monjezi and A. A. Rownaghi, Chem. Eng. J., 2017, 313, 1346–1353 CrossRef CAS.
- C. Zhang, L. Ai and J. Jiang, J. Mater. Chem. A, 2015, 3, 3074–3081 CAS.
- H. Zhao, L. Qian, H. Lv, Y. Wang and G. Zhao, ChemCatChem, 2015, 7, 4148–4155 CrossRef CAS.
- L. Ai, C. Zhang, L. Li and J. Jiang, Appl. Catal., B, 2014, 148–149, 191–200 CrossRef CAS.
- I. Y. Skobelev, A. B. Sorokin, K. A. Kovalenko, V. P. Fedin and O. A. Kholdeeva, J. Catal., 2013, 298, 61–69 CrossRef CAS.
- G. Férey, C. Mellot-Draznieks, C. Serre, F. Millange, J. Dutour, S. Surblé and I. Margiolaki, Science, 2005, 309, 2040–2042 CrossRef PubMed.
- M. H. Mashhadizadeh, M. Amoli-Diva and K. Pourghazi, J. Chromatogr. A, 2013, 1320, 17–26 CrossRef CAS PubMed.
- C. Lee, C. R. Keenan and D. L. Sedlak, Environ. Sci. Technol., 2008, 42, 4921–4926 CrossRef CAS PubMed.
- A. L.-T. Pham, C. Lee, F. M. Doyle and D. L. Sedlak, Environ. Sci. Technol., 2009, 43, 8930–8935 CrossRef CAS PubMed.
- P. Hu, Y. Liu, B. Jiang, X. Zheng, J. Zheng and M. Wu, Ind. Eng. Chem. Res., 2015, 54, 8277–8286 CrossRef CAS.
- K.-i. Ishibashi, A. Fujishima, T. Watanabe and K. Hashimoto, Electrochem. Commun., 2000, 2, 207–210 CrossRef CAS.
- S.-H. Huo and X.-P. Yan, Analyst, 2012, 137, 3445–3451 RSC.
- S. Zhang, Z. Du and G. Li, Talanta, 2013, 115, 32–39 CrossRef CAS PubMed.
- Z. Wan and J. Wang, RSC Adv., 2016, 6, 103523–103531 RSC.
- W. Wang, M. Zhou, Q. Mao, J. Yue and X. Wang, Catal. Commun., 2010, 11, 937–941 CrossRef CAS.
- L. Xu and J. Wang, Environ. Sci. Technol., 2012, 46, 10145–10153 CrossRef CAS PubMed.
- J. Feng, X. Hu and P. L. Yue, Environ. Sci. Technol., 2004, 38, 5773–5778 CrossRef CAS PubMed.
- M. Luo, D. Bowden and P. Brimblecombe, Appl. Catal., B, 2009, 85, 201–206 CrossRef CAS.
- Y. Yao, Y. Cai, G. Wu, F. Wei, X. Li, H. Chen and S. Wang, J. Hazard. Mater., 2015, 296, 128–137 CrossRef CAS PubMed.
- M. Li, D. Wen, Z. Qiang and J. Kiwi, RSC Adv., 2017, 7, 7640–7647 RSC.
- T. Zhang, H. Zhu and J.-P. Croue, Environ. Sci. Technol., 2013, 47, 2784–2791 CrossRef CAS PubMed.
- M. Fujita, Y. J. Kwon, S. Washizu and K. Ogura, J. Am. Chem. Soc., 1994, 116, 1151–1152 CrossRef CAS.
- L. H. Wee, M. R. Lohe, N. Janssens, S. Kaskel and J. A. Martens, J. Mater. Chem., 2012, 22, 13742–13746 RSC.
- L. Alaerts, E. Seguin, H. Poelman, F. Thibault-Starzyk, P. A. Jacobs and D. E. De Vos, Chem.–Eur. J., 2006, 12, 7353–7363 CrossRef CAS PubMed.
- A. Corma, H. García and F. X. Llabrés i Xamena, Chem. Rev., 2010, 110, 4606–4655 CrossRef CAS PubMed.
- R. C. C. Costa, F. C. C. Moura, J. D. Ardisson, J. D. Fabris and R. M. Lago, Appl. Catal., B, 2008, 83, 131–139 CrossRef CAS.
- W. Luo, L. Zhu, N. Wang, H. Tang, M. Cao and Y. She, Environ. Sci. Technol., 2010, 44, 1786–1791 CrossRef CAS PubMed.
- X. Hu, B. Liu, Y. Deng, H. Chen, S. Luo, C. Sun, P. Yang and S. Yang, Appl. Catal., B, 2011, 107, 274–283 CrossRef CAS.
|
This journal is © The Royal Society of Chemistry 2017 |
Click here to see how this site uses Cookies. View our privacy policy here.