DOI:
10.1039/C7RA01620D
(Paper)
RSC Adv., 2017,
7, 14742-14751
A novel and simple solvent-dependent fluorescent probe based on a click generated 8-aminoquinoline–steroid conjugate for multi-detection of Cu(II), oxalate and pyrophosphate†
Received
8th February 2017
, Accepted 28th February 2017
First published on 6th March 2017
Abstract
In this work, a novel and simple deoxycholic acid-based fluorescent probe for solvent-dependent multi-detection of Cu2+, C2O42− and P2O74− has been facilely constructed by click chemistry. This probe displayed sensitive recognition toward Cu2+ in low-water-content CH3CN aqueous solution (CH3CN–H2O, 99/1, v/v) via strong fluorescence quenching. Whereas, in DMSO–H2O medium (1/1, v/v), it exhibited significant fluorescent enhancement for C2O42− and P2O74− over a wide range of tested anions. Moreover, with low detection limits and fast response time, the developed fluorescent molecule was used successfully for analysis of the target ions on test paper strips and in water samples.
Introduction
The development of effective fluorescent probes for the detection of small cations, anions, and neutral molecules is of great interest and significance because of the vital roles of these species in biological, medical and environmental processes.1–3 Conventionally, to obtain an excellent fluorescent probe, high selectivity for one analyte should be given priority in the traditional sensor design. However, the applications of these single-selective fluorescent molecules are frequently limited by their simple recognition properties resulting from the notable background interference in complicated real samples. Therefore, multi-analyte fluorescent probes, which can be regulated to bind more than one guest species with distinct spectral changes in different media based upon a single host, have gradually become an intriguing and promising research focus during the past few years.4–17 Undoubtedly, this innovative transformation from specific to differential detection with a multi-functional probe by varying the compositions of solvent would simplify the analysis work and reduce the cost of synthesis. At present, finding novel and simple solvent-dependent fluorescent probes for multi-analyte detection is still one of the most challenging tasks.
Copper ion (Cu2+) is an essential trace element in human body and serves as an important catalytic cofactor in a variety of fundamental physiological processes owing to its unique redox-active nature.18 The levels of Cu2+ are firmly controlled in biological systems, as its disorders can interfere in cellular metabolism and cause many neurodegenerative diseases.19–21 In addition, Cu2+ is also considered as a serious pollutant in the natural environment on account of its high toxicity. Oxalate (C2O42−) is abundantly present in nature as an important nutrient found in the human diet.22 And it is also a primary chelator of calcium ions and forms insoluble chelates with dietary calcium, which inhibits calcium absorption in the body and is finally accumulates in the renal tissue, thus inducing renal injury, kidney lesions and pancreatic insufficiency.23,24 As an essential anion for normal cellular function, pyrophosphate (P2O74−) involves in many crucial biochemical pathways, such as DNA and RNA polymerizations, cellular ATP hydrolysis and energy storage and transmission.25 The difference in P2O74− concentrations is also related to blood-clotting, telomerase activity and calcium pyrophosphate dihydrate crystal deposition disease, and therefore can be used as a potential indicator in clinical diagnosis.26,27 Moreover, P2O74− is one of the major phosphorus pollutants due to its wide application in our daily lives. Consequently, the development of fluorescent probes for the facile detection of Cu2+, C2O42− and P2O74− is very important to control their concentration levels in the biosphere and avoid severe damage to humans.
Although a number of dual functional fluorescent probes for Cu2+/C2O42− and Cu2+/P2O74− have been developed,28–38 these chemosensors for anions are always based upon the use of metal complex receptors, especially dinuclear Cu2+ complex, or the metal displacement approaches. Using these indirect sensing out-put methods, the detection of C2O42− or P2O74− is very much dependent on the design of an appropriate metal coordinative complex and the affinity of the metal ion for its complementary anion, which could be affected by various factors and is less easily realizable. Besides, compared to P2O74−, limited fluorescent probes for C2O42− have been reported.28–30,39–41 Overcoming these problems in the development of novel and simple solvent-dependent fluorescent probes that can exhibit differential optical responses in different solvent media for simultaneous and multiple detection of Cu2+, C2O42− and P2O74− is highly desirable.
In this work, we demonstrated that a new and uncomplicated tweezer-type molecule 1 containing deoxycholic acid, 8-aminoquinoline and 1,2,3-triazole moieties could be employed as a multifunctional fluorescent probe for monitoring Cu2+, C2O42− and P2O74− in two different solvents. As a natural product in the steroid family, deoxycholic acid can provide an ideal scaffold for receptors with its rigid framework and easily chemically-modified functional groups.42 And 8-aminoquinoline and its derivatives are well-known excellent building blocks for constructing highly selective fluorescent sensors of transition metal cations.36,43–46 Meanwhile, the bridging 1,2,3-triazole group was introduced via click chemistry in order to offer not only a straightforward strategy for the linkage of various functionalities, but also a potential binding site for both metal ions and anions,47,48 which is very beneficial for multi-target analysis. Bearing these considerations in mind, fluorescent probe 1 was facilely synthesized by the Cu(I)-catalyzed 1,3-dipolar cycloaddition between steroidal diazides (2) and quinoline terminal alkyne (3) (Scheme 1). All the intermediates and probe 1 were well characterized (Fig. S1–S5†). The spectroscopic properties illustrated that this easily available fluorescent probe could propose a simple and sensitive solvent-dependent assay method with low detection limits and fast response time for multi-detection. Probe 1 displayed strong fluorescence quenching upon binding to Cu2+ in acetonitrile (CH3CN) and water, and at the same time it exhibited remarkable fluorescence enhanced response toward C2O42− and P2O74− in the dimethyl sulfoxide (DMSO) aqueous solution. The practical analytical utility of the probe was also validated to determine Cu2+, C2O42− and P2O74− on test paper strips and in water samples.
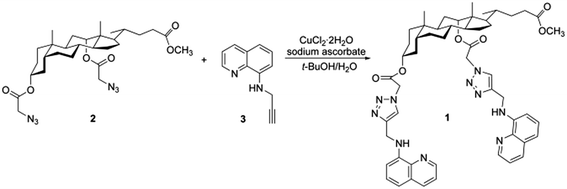 |
| Scheme 1 The synthetic route of probe 1. | |
Experimental
Materials and apparatus
All chemicals were of analytical grade or higher, obtained from commercial suppliers and used without further purification. Column chromatography and thin layer chromatography were performed on silica gel (200–300 mesh) and silica gel GF254 plates, respectively. Deionized water was used for all aqueous solutions. Hydroxyethyl piperazine ethanesulfonic acid (HEPES) and sodium hydroxide were used to prepare buffer solutions. The metal ion solutions were prepared from their chloride or nitrate salts. The anion solutions were prepared from their quaternary ammonium or sodium salts.
Nuclear magnetic resonance (NMR) spectra were recorded on a Bruker Advance III 400 spectrometer. High resolution electrospray ionization mass spectrometry (HR-ESI-MS) spectra were measured on a Bruker micrOTOF-Q II mass spectrometer. Fluorescence spectra were obtained on a Hitachi F-2700 fluorescence spectrophotometer under 350 nm excitation with both excitation and emission slit widths set at 5 nm. The photomultiplier voltage was 700 V. Ultraviolet-visible (UV-vis) absorption spectra were collected on a Shimadzu UV-2700 UV-vis spectrophotometer. All pH measurements were conducted with a Mettler Toledo SG2 pH meter.
Synthesis of probe 1
Compound 2 was synthesized according to the previous ref. 49. 1H NMR (400 MHz, CDCl3): δ (ppm) 0.74 (s, 3H, 18-CH3), 0.81 (d, 3H, 21-CH3), 0.92 (s, 3H, 19-CH3), 3.65 (s, 3H, OCH3), 3.83 (s, 2H, –COCH2), 3.88 (d, 2H, –COCH2), 4.81 (m, 1H, 3-CH), 5.25 (br, 1H, 12-CH).
Compound 3 was synthesized according to the previous ref 50. 1H NMR (400 MHz, CDCl3): δ (ppm) 2.26 (s, 1H,
CH), 4.17 (s, 2H, –CH2–), 6.43 (br, 1H, –NH–), 6.82 (d, 1H), 7.15 (d, 1H), 7.37 (m, 1H), 7.44 (m, 1H), 8.06 (d, 1H), 8.75 (d, 1H).
Synthesis of 1. To a solution of compound 2 (372 mg, 0.65 mmol) and compound 3 (260 mg, 1.43 mmol) in tert-butanol (30 mL) and water (2 mL), CuCl2·2H2O (16.6 mg, 0.098 mmol) and sodium ascorbate (77.3 mg, 0.39 mmol) were added. The resulting mixture was stirred at 65 °C for 7 h. Then the solvent was evaporated under vacuum. The residue was dissolved in dichloromethane (50 mL) and washed with saturated brine (3 × 50 mL). After being dried over anhydrous magnesium sulphate and filtered, the organic layer was condensed to dryness. The crude product was purified by column chromatography (dichloromethane–ethanol, 15/1, v/v) to afford 1 as a light yellow solid (316 mg, yield 52%). 1H NMR (400 MHz, CDCl3): δ (ppm) 0.66, 0.83 (2 × s, 2 × 3H, 18, 19-CH3), 0.74 (d, 3H, 21-CH3), 3.63 (s, 3H, –COOCH3), 4.60 (s, 2H, –COCH2–), 4.70 (m, 3H, 3-H, –COCH2–), 5.09 (m, 5H, –NHC
2–, 12-H), 6.67 (d, 1H), 6.75 (d, 1H), 7.04 (m, 2H), 7.33 (m, 4H), 7.61, 7.65 (2 × s, 2 × 1H, triazole-H), 8.01 (m, 2H), 8.64, 8.68 (2 × dd, 2 × 1H). 13C NMR (100 MHz, CDCl3): δ (ppm) 12.1, 17.6, 22.6, 23.1, 25.2, 25.7, 26.0, 26.5, 27.1, 30.6, 30.8, 31.5, 33.7, 33.9, 34.4, 34.5, 35.2, 39.39, 39.43, 41.4, 44.9, 47.3, 49.2, 51.1, 51.4, 76.5, 78.3, 105.15, 105.24, 114.5, 114.6, 121.37, 121.42, 123.0, 123.3, 127.6, 127.7, 128.50, 128.51, 136.0, 137.98, 138.04, 143.97, 143.99, 146.6, 146.86, 146.89, 165.3, 165.9, 174.5. HR-ESI-MS m/z [M + H]+ calcd for C53H65N10O6+ 937.5089, found 937.5073; [M + Na]+ calcd for C53H64N10NaO6+ 959.4908, found 959.4882.
General methods for spectroscopic analysis
A stock solution of probe 1 (1 mM) was prepared in CH3CN or DMSO, respectively. Metal ion solutions (50 mM) and anion solutions (20 mM) were prepared in deionized water. Buffer solution for tests was HEPES (10 mM). For determination of spectroscopic properties, probe 1 stock solution (1.0 mM), appropriate aliquots of organic solvent and each analyte standard solution were transferred into a microtube by a pipette and then diluted with HEPES (10 mM). All spectroscopic measurements were carried out at 25 °C with samples placed in a 1 cm path-length quartz cuvette. The relative fluorescence quantum yields for probe 1 and complexes emission in different solvents were measured using anthracene (Φ = 0.36, in cyclohexane) as the quantum yield standard.51
Determination of the detection limit and the association constant
The detection limit was calculated from the following equation based on fluorescence titration52:
Sd is the standard deviation of blank measurements without ion tested and k is the slope between the fluorescence intensity at the maximum emission wavelength versus ion concentration. The fluorescence intensity of the blank probe 1 (20 μM) was measured 6 times.
The association constant (Ka) of probe 1 with ion tested was calculated from the Benesi–Hildebrand equation53:
F is the fluorescence intensity at a given ion concentration,
F0 is the fluorescence intensity in the absence of ion, and
Feq is the equilibrium fluorescence intensity.
C is the concentration of ion added. The association constant value was determined graphically by plotting 1/(
F −
F0) against 1/
C.
Practical application experiments
Test paper strips were prepared by immersing normal filter papers into a solution of probe 1 (1 mM) in CH3CN or DMSO and dried in air. Then the test strips were immersed into the solutions containing different concentrations of ion tested and dried in air.
Tap water samples from different sources were freshly collected and passed through a microfiltration membrane before use. Prior to detection, the fluorescence intensity of the probe system at the maximum emission wavelength in the presence of various concentrations of ion tested was quantified under the optimized fluorescence titration conditions, and the corresponding plots were prepared as the standard curves. Then samples spiked with different concentrations of ion were quantified under the same detection conditions by fitting to the standard curves.
Results and discussion
Spectral studies of probe 1 with metal ions
First, probe 1 having nitrogen atoms of triazoles and aminoquinolines with several lone pair electrons could coordinate with metal ions, so the binding properties of probe 1 toward various metal ions, including Cu2+, Fe2+, Zn2+, Ag+, Hg2+, K+, Mg2+, Al3+, Na+, Cr3+, Ca2+, Cd2+ and Pb2+, were studied by fluorescence measurement. As shown in Fig. 1a, the free probe displayed an evident emission peak with the maximum intensity at around 470 nm (Φ = 0.036) in CH3CN–H2O (99/1, v/v, 10 mM HEPES, pH 7.2), which are characteristics of the 8-aminoquinoline moiety. Upon the addition of 5.0 equivalent of Cu2+ into the solution of probe 1, remarkable emission quenching was observed, while only slight decrease or a minimal change was detected for other tested metal ions under the identical conditions. The selective binding behavior of probe 1 for Cu2+ was also confirmed by the visual emission picture taken under 365 nm light, in which the solution color of the probe was quenched evidently from bright blue to dark black only in the presence of Cu2+ (Fig. 1b). The high selectivity might be attributed to the nice binding pocket for Cu2+ provided by triazole and aminoquinoline moieties.
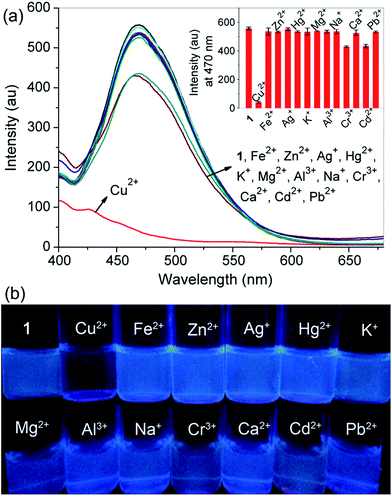 |
| Fig. 1 (a) Fluorescence spectra of probe 1 (20 μM) with different metal ions (100 μM) in CH3CN–H2O (99/1, v/v, 10 mM HEPES, pH 7.2). λex = 350 nm. Inset: fluorescence intensity of probe 1 at 470 nm with different metal ions. (b) Photographs of probe 1 (100 μM) with different metal ions (200 μM) in CH3CN–H2O (99/1, v/v, 10 mM HEPES, pH 7.2) taken under 365 nm illumination. | |
During Cu2+ titration, a gradual decrease in the emission intensity of probe 1 solution could be visible and reached a minimum after adding 5.0 equivalent of Cu2+. The observed emission maximum at 470 nm was nearly proportional to the Cu2+ concentration (0–5.0 equivalent), and the equilibrium fluorescence intensity was quenched about 93% of the original data (Fig. 2). The fluorescence quenching was attributed to the formation of the probe 1–Cu2+ complex, resulting in the metal-to-ligand charge transfer upon excitation.54 In this case the results are as expected because the d9 electron configuration of Cu2+ usually produces a chelation enhancement of quenching (CHEQ) effect during its metal complex formation.55 The UV-vis absorption spectrum of probe 1 was characterized by two typical absorbance bands of aminoquinoline centered at 255 (ε = 54150 M−1 cm−1) and 355 nm (ε = 12950 M−1 cm−1) before titration in CH3CN–H2O (99/1, v/v, 10 mM HEPES, pH 7.2). When Cu2+ was successively introduced, there was a blueshift from 255 to 230 nm, while the absorption at 355 nm gradually increased and remained constant up to the addition of 5.0 equivalent of Cu2+ (ε = 25500 M−1 cm−1), and a clear isosbestic point was observed at 266 nm. These results suggested that a metal complex was generated from the binding of probe 1 with Cu2+ (Fig. S6†). The absorption spectra of the probe were also investigated with the aforementioned competing metal ions under the same conditions, but only slight changes were noticed, which manifested again the selectivity of probe 1 toward Cu2+ (Fig. S7†).
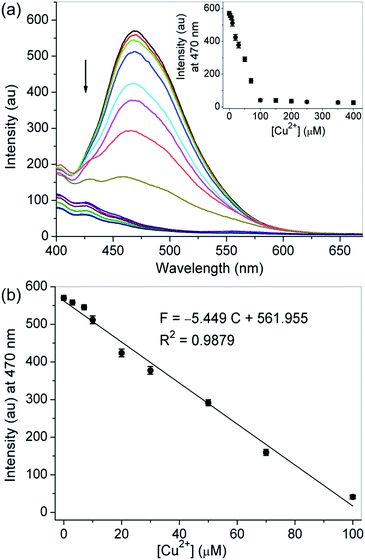 |
| Fig. 2 (a) Fluorescence spectra of probe 1 (20 μM) with different levels of Cu2+ (from top to bottom: 0, 3, 7, 10, 20, 30, 50, 70, 100, 150, 200, 250, 350 and 400 μM) in CH3CN–H2O (99/1, v/v, 10 mM HEPES, pH 7.2). λex = 350 nm. Inset: fluorescence intensity changes of probe 1 at 470 nm as a function of the whole range of Cu2+ concentration tested. (b) Fluorescence intensity changes of probe 1 at 470 nm as a function of the Cu2+ concentration from 0 to 100 μM. | |
Job's plot experiments were carried out to determine the binding stoichiometry of probe 1 and Cu2+ under a fixed total concentration. Represented in Fig. 3a, a maximum value was obtained when the molar fraction of probe 1 was 0.5, revealing a 1
:
1 ratio for the 1–Cu2+ complex. Based on the Benesi–Hildebrand method, the association constant (Ka) of probe 1 with Cu2+ was calculated to be 3.28 × 103 M−1 (Fig. 3b). The detection limit of molecule 1 as a fluorescent probe for testing Cu2+ was determined to be 0.12 μM by using 20 μM probe 1 in fluorescence titration, which is reasonable for the analysis of micromolar concentrations of Cu2+.
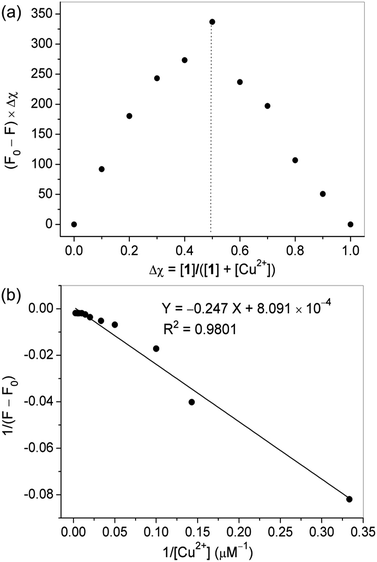 |
| Fig. 3 (a) Job's plot from the fluorescence spectra of probe 1 and Cu2+ with a total concentration of 100 μM. λex = 350 nm. (b) Benesi–Hildebrand plot from the fluorescence titration data of probe 1 (20 μM) with Cu2+. The fluorescence intensity was monitored at 470 nm. | |
Solvent greatly affected the fluorescence emission properties of the probe and its response to Cu2+. To obtain a suitable testing medium, the fluorescence emission spectra of probe 1 with Cu2+ were investigated in different solvents, including methanol, ethanol, N,N-dimethylformamide (DMF), DMSO and CH3CN. Interestingly, when CH3CN was used as the solvent, obvious and steady fluorescence quenching signals could be detected. In addition, the fluorescence response of probe 1 in the absence and presence of Cu2+ was found to be dependent on the ratio of CH3CN to water (Fig. S8†). In low-water-content CH3CN-buffer media, the probe itself gave strong fluorescent signals and efficient fluorescence quenching could be observed with the presence of Cu2+. To get a better detection sensitivity and solubility of metal ion, here a CH3CN–H2O solution (99/1, v/v, 10 mM HEPES, pH 7.2) was chosen as the testing system to investigate the spectral characteristics of probe 1 with Cu2+. The pH titration of probe 1 was performed to determine a suitable pH range for Cu2+ sensing (Fig. S9†). The results indicated that high-efficiency fluorescence quenching of probe 1 by Cu2+ was obtained in the tested pH range of 4.0–7.2, but the spectral response toward Cu2+ declined with the increasing pH values as a result of the hydrolysis of metal ion in basic solution. A time course study revealed that the recognizing process could complete immediately after the addition of Cu2+ (Fig. S10†). This feature of fast detection of Cu2+ is particularly favorable in practical application.
Spectral studies of probe 1 with anions
On the other hand, probe 1 having protons of acetyl methylenes, triazole rings and –NH in aminoquinolines made a very favorable platform for hydrogen bonding interactions with anions, which might change its electronic properties giving rise to an alteration of the emission properties. Hence, the recognition abilities of probe 1 toward different anions, containing C2O42−, P2O74−, F−, Cl−, Br−, I−, AcO−, H2PO4−, HSO4−, NO3−, CO32−, N3−, S2−, SCN− and ClO4−, were examined by fluorescence spectroscopy. As depicted in Fig. 4a, in DMSO–H2O (1/1, v/v, 10 mM HEPES, pH 7.2), the probe itself exhibited a characteristic emission band of 8-aminoquinoline centered at 464 nm with weaker fluorescence intensity (Φ = 0.024) than in CH3CN–H2O (99/1, v/v, 10 mM HEPES, pH 7.2). While upon the addition of 1.5 equivalent of C2O42− or P2O74− into the solution of probe 1, great fluorescence enhancement could be detectable. For other tested anions, no obvious fluorescence changes were observed under the identical conditions. The selectivity of probe 1 for C2O42− and P2O74− was further identified by the visual emission picture taken under 365 nm light, in which the probe showed notable turn-on responses only in the presence of C2O42− and P2O74− (Fig. 4b). We believe that these results are related to the combined effects of both basicity and size or shape of the anions. For probe 1, the selectivity might be due to its relatively flexible and well matched binding pocket, in which acetyl methylene protons, triazole ring protons and –NH protons of aminoquinoline could make favorable hydrogen bonding interactions with C2O42− and P2O74−.
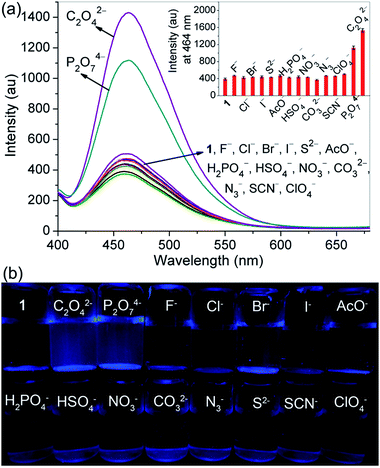 |
| Fig. 4 (a) Fluorescence spectra of probe 1 (20 μM) with different anions (30 μM) in DMSO–H2O (1/1, v/v, 10 mM HEPES, pH 7.2). λex = 350 nm. Inset: fluorescence intensity of probe 1 at 464 nm with different anions. (b) Photographs of probe 1 (10 μM) with different anions (30 μM) in DMSO–H2O (1/1, v/v, 10 mM HEPES, pH 7.2) taken under 365 nm illumination. | |
In the fluorescence titration profiles (Fig. 5), a gradual increase in the emission intensity of probe 1 solution could be observed with increasing C2O42− or P2O74− concentration until 3.5 equivalent of anion added. The emission maximum at 464 nm increased linearly with the addition of C2O42− (0–1.5 equivalent) or P2O74− (0–2.5 equivalent), and the equilibrium fluorescence intensity was increased 4.5 and 3.8 fold respectively. For probe 1, the pre-organized tweezer-type scaffold with triazole rings and 8-aminoquinoline moieties might lead to the formation of a perfect hydrogen bonding cavity for accommodating C2O42− or P2O74−. The strong complexation between the oxygen atoms of the tested two anions and the –NH groups of quinoline parts could enhance the N–H…O hydrogen bonding interaction, which resulted in the inhibition for the photo induced electron transfer (PET) process from the attached amino group to the excited singlet state of quinoline. Consequently, the fluorescence of the probe increased as the concentration of the tested anions was increased. With regard to the free probe, 11-fold and 4.2-fold quantum yields were found for the detection of C2O42− (Φ = 0.26) and P2O74− (Φ = 0.10) respectively. The big difference in quantum yield can be used to discriminate these two tested anions from each other. But, unlike the interaction between probe 1 and metal ions, no significant changes were observed in the bands of the absorption spectra of the probe after adding all the above-mentioned tested anions (Fig. S11 and S12†).
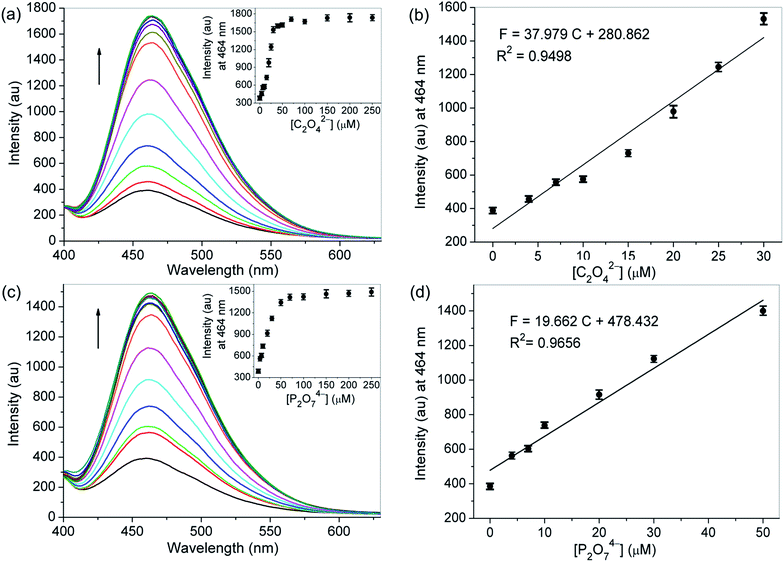 |
| Fig. 5 Fluorescence spectra of probe 1 (20 μM) with different levels of (a) C2O42− (from bottom to top: 0, 4, 7, 10, 15, 20, 25, 30, 40, 50, 70, 100, 150, 200 and 250 μM) and (c) P2O74− (from bottom to top: 0, 4, 7, 10, 20, 30, 50, 70, 100, 150, 200 and 250 μM) in DMSO–H2O (1/1, v/v, 10 mM HEPES, pH 7.2). λex = 350 nm. Inset: fluorescence intensity changes of probe 1 at 464 nm as a function of the whole range of C2O42−/P2O74− concentration tested. Fluorescence intensity changes of probe 1 at 464 nm as a function of (b) the C2O42− concentration from 0 to 30 μM and (d) the P2O74− concentration from 0 to 50 μM. | |
Job's plot experiments were carried out to determine the binding stoichiometry of probe 1 with C2O42− or P2O74− under a constant total concentration. As shown in Fig. 6a and c, a maximum value was obtained when the molar fraction of probe 1 was 0.5, indicating that 1
:
1 stoichiometric complexation of the probe with C2O42− or P2O74− was formed, respectively. Based on the fluorescence titration data, the association constant (Ka) of probe 1 for C2O42− or P2O74− was calculated by the Benesi–Hildebrand expression, and determined to be 2.23 × 104, and 4.96 × 104 M−1, respectively (Fig. 6b and d), indicating that the probe had high binding affinities for these two anions. The detection limits of molecule 1 as a fluorescent probe for the analysis of C2O42− and P2O74− were calculated and found to be 0.28 and 0.55 μM, respectively, by using 20 μM probe 1 in fluorescence titration, which are appropriate for the detection of micromolar concentrations of these two ions.
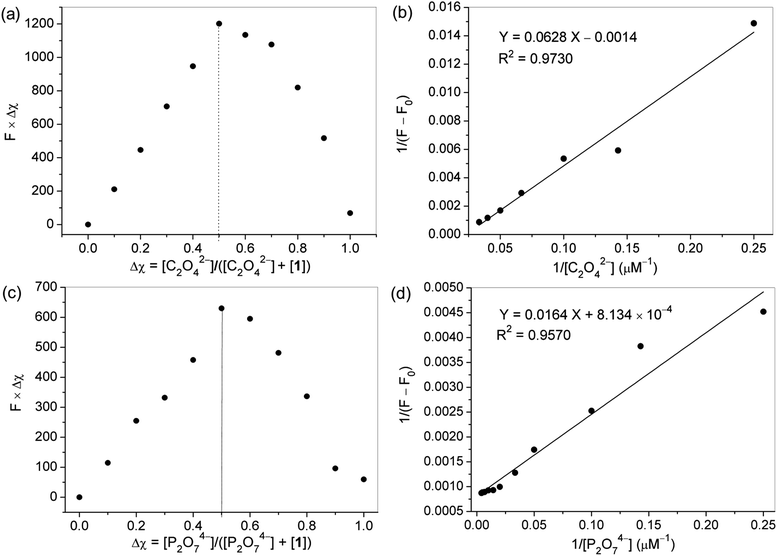 |
| Fig. 6 Job's plot from the fluorescence spectra of (a) probe 1 and C2O42− with a total concentration of 100 μM, and (c) probe 1 and P2O74− with a total concentration of 50 μM. λex = 350 nm. Benesi–Hildebrand plot from the fluorescence titration data of probe 1 (20 μM) with (b) C2O42− and (d) P2O74−. The fluorescence intensity was monitored at 464 nm. | |
To obtain suitable testing media, the fluorescence emission spectra of probe 1 with C2O42− or P2O74− were investigated in different solvents, including methanol, ethanol, DMF, DMSO, CH3CN and aqueous solutions of these organic solvents. Although most of the anion recognition process was observed in non-aqueous and aprotic solvents, the results indicated that high signal turn-on ratios and better sensitivity could be obtained when the spectral properties of probe 1 with the tested two anions were investigated in an optimized mixture of DMSO–H2O (1/1, v/v, 10 mM HEPES, pH 7.2) (Fig. S13†). The appropriate-water-content system might be able to improve the sodium salts solubility of the tested anions. The pH titration of probe 1 was performed to determine suitable pH ranges for C2O42− and P2O74− sensing. As depicted in Fig. S14,† high-efficiency fluorescence enhancement of probe 1 by C2O42− or P2O74− was observed in the tested pH range of 7.0–8.0. Time course studies revealed that the recognizing processes could complete immediately after the addition of C2O42− or P2O74− (Fig. S15†). The features of fast detection of C2O42− and P2O74− are particularly important and favorable in practical application.
Detection mechanism
To get a better understanding of the binding modes of probe 1 with Cu2+, C2O42− and P2O74−, 1H NMR titration studies were performed as shown in Fig. 7 and 8.
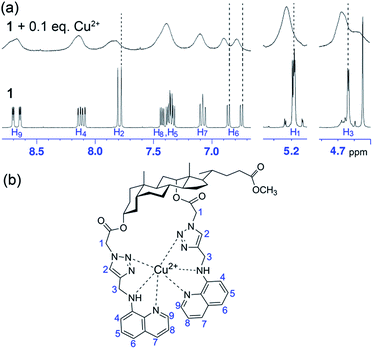 |
| Fig. 7 (a) Partial 1H NMR spectra of probe 1 (10 mM) measured before and after addition of Cu2+ (0.1 equivalent) in CD3CN–D2O (99/1, v/v). (b) The proposed binding mode between probe 1 and Cu2+. | |
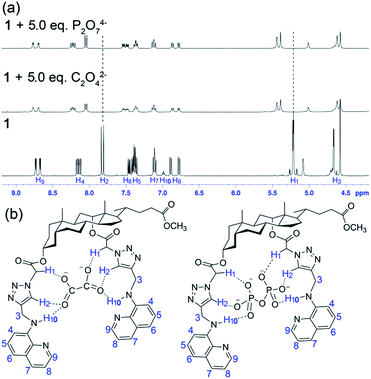 |
| Fig. 8 (a) Partial 1H NMR spectra of probe 1 (10 mM) measured before and after addition of C2O42− or P2O74− (5.0 equivalent) in deuterated DMSO–D2O (19/1, v/v). (b) The proposed binding interactions between probe 1 and anions. | |
The 1H NMR spectra of probe 1 in the presence of increasing concentrations of Cu2+ resulted in broadening and deshielding of the proton signals of triazole units, aminoquinoline moieties as well as the two kinds of aliphatic methylenes in the tweezer-type side chains as compared to those of free probe (Fig. 7a). After the addition of 0.1 equivalent of Cu2+ to the solution of probe 1 (10 mM) in CD3CN–D2O (99/1, v/v), both triazole protons (H2) showed slight downfield shifts by 0.025 and 0.055 ppm, respectively. Besides, slight downfield shifts in H6 of aminoquinoline moieties by 0.020 and 0.030 ppm, respectively, along with deshielding of methylene protons (H1 and H3) by 0.015 ppm suggested the crucial roles of 1,2,3-triazole rings and aminoquinoline groups as binding sites in the recognition of Cu2+. Due to the paramagnetic property of Cu2+, the protons of steroidal skeleton moiety and methylenes in the tweezer-type side chains (H1 and H3) became more broadened, while the protons of triazole and aminoquinoline groups (H2, H4–H9) disappeared completely even in the presence of 0.5 equivalent of Cu2+ (Fig. S16†). The reversible binding ability of probe 1 toward Cu2+ was verified by introduction of ethylenediaminetetraacetic acid disodium salt (EDTA-2Na) into the system containing probe 1 (20 μM) and Cu2+ (100 μM). The experiment results showed that the completed quenched fluorescence signal of probe 1 by Cu2+ was recovered gradually with the addition of increasing concentrations of EDTA (Fig. S17†). Based on the spectral and 1H NMR data, a tentative binding mode between probe 1 and Cu2+ is presented in Fig. 7b.
The 1H NMR titration of probe 1 with C2O42− and P2O74− illustrated the involvement of protons in anion binding. As shown in Fig. 8a, upon the addition of 5.0 equivalent of C2O42− or P2O74− to the solution of probe 1 (10 mM) in deuterated DMSO–D2O (19/1, v/v), downfield shifts of triazole protons (H2, 7.80–8.02 and 7.84–8.04 for C2O42−, and 7.80–8.01 and 7.84–8.03 for P2O74−) and acetyl methylene protons (H1, 5.21–5.37 and 5.21–5.44 for both C2O42− and P2O74−) were observed, respectively. And the proton signals of –NH at 7.0 ppm (H10) in aminoquinoline moieties became invisible after addition of the tested two anions, presumably as a result of broadening by hydrogen bond interactions. The quinoline ring protons H4 and H9 showed slight downfield shifting on complexation. These clearly indicated the participation of triazole protons, H1 methylene protons as well as –NH protons in the hydrogen bonding with C2O42− and P2O74−. The reversible binding abilities of probe 1 toward C2O42− and P2O74− were verified by introduction of Pb2+ into the probe–anion complex system. The experiment results demonstrated that the enhanced fluorescence of probe 1 by C2O42− or P2O74− was quenched gradually with the addition of increasing concentrations of Pb2+, while the addition of Pb2+ to the solution of probe 1 alone did not cause obvious change under the identical conditions, suggesting that the presence of Pb2+ quenched the fluorescence of the probe–anion complex through the formation of insoluble salts between Pb2+ and the tested anion, and therefore the probe–anion complexation was reversible (Fig. S18†). The proposed binding interactions between probe 1 and anions are given in Fig. 8b according to the spectral and 1H NMR data.
Analytical application
To investigate the practical applications of the probe, experiments for visual detection of Cu2+, C2O42− and P2O74− on solid paper strips with naked eyes under 365 nm light were first performed. As shown in Fig. 9, remarkable color changes of the paper strips containing probe 1 (1 mM) appeared clearly from bright blue to dark black upon interaction with different levels of Cu2+ (0, 100, 300, 500, 1000 μM) under UV light. Interestingly, the color of the paper strips containing probe 1 (1 mM) showed immediate and sensitive changes from dark blue to light blue after interaction with C2O42− or P2O74− (0, 300, 5000 μM) under UV light. The utilization of these dipsticks is particularly useful as an instant qualitative method without resorting to instrumental analysis.
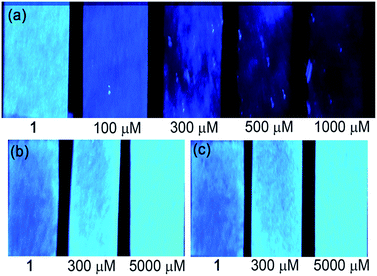 |
| Fig. 9 Photographs of test paper strips containing probe 1 (1 mM) for detection of (a) Cu2+, (b) C2O42− and (c) P2O74− with different concentrations under 365 nm illumination. | |
The probe was also applied to the detection of our target ions in tap water samples. Before the experiments, the corresponding calibration plots were prepared as the standard curves under the optimized fluorescence titration conditions. No significant fluorescence changes were produced with both tap water samples, indicating that these samples are free from detectable amount of target ions. The recovery studies were further conducted by spiking two different concentrations of Cu2+, C2O42− or P2O74− in triplicate to both the samples. The good percentage recoveries proved the fine accuracy of this fluorescent system and its potential applicability in environmental water (Table 1).
Table 1 Recovery of Cu2+, C2O42− and P2O74− in spiked water samplesa
Ions |
Spiked (μM) |
Tap water 1 |
Tap water 2 |
Found (μM) |
Recovery (%) |
Found (μM) |
Recovery (%) |
Mean (n = 3) ± standard deviation. |
Cu2+ |
10 |
10.26 ± 0.11 |
102.6 |
11.13 ± 0.21 |
111.3 |
30 |
29.44 ± 0.13 |
98.1 |
29.52 ± 0.19 |
98.4 |
C2O42− |
10 |
10.06 ± 0.39 |
100.6 |
9.63 ± 0.10 |
96.3 |
25 |
24.60 ± 0.32 |
98.4 |
24.50 ± 0.37 |
98.0 |
P2O74− |
10 |
9.86 ± 0.25 |
98.6 |
10.20 ± 0.33 |
102.0 |
25 |
24.46 ± 0.69 |
97.8 |
25.15 ± 0.14 |
100.6 |
Conclusions
In summary, a novel and simple deoxycholic acid-based fluorescent probe for multi-detection of Cu2+, C2O42− and P2O74− in different media has been facilely constructed using Cu(I)-catalyzed click reaction of steroidal azides and quinoline terminal alkyne. In this molecule, binding sites and the fluorophore subunits are covalently bonded to form a relatively flexible binding cleft, in which nitrogen atoms of triazoles and aminoquinolines provided a matched binding pocket for Cu2+ with strong fluorescence quenching in CH3CN aqueous solution, while protons of acetyl methylenes, triazole rings and –NH in aminoquinolines made favorable hydrogen bonding interactions with C2O42− and P2O74− accompanied by obvious fluorescence enhancement in DMSO aqueous solution. With high sensitivity, low detection limits and fast response time, this fluorescent probe also showed good performance of determination on test paper strips and in water samples. Compared with the recently reported multi-detection probes for Cu2+, C2O42− and P2O74− (Table S1†), our report is the first single fluorescent molecule to our knowledge that can separately detect them with distinct emission in different solvents. The design strategy of the molecule will be helpful in developing fluorescent probes for multi-analyte response, thus probably providing further insight into how to construct efficient sensors with special properties.
Acknowledgements
This work is supported by the National Natural Science Foundation of China (21102112), the Scientific and Technological Development Project of Shaanxi Province (2015GY148), and the Fundamental Research Funds for the Central Universities.
Notes and references
- X. Chen, F. Wang, J. Y. Hyun, T. Wei, J. Qiang, X. Ren, I. Shin and J. Yoon, Chem. Soc. Rev., 2016, 45, 2976–3016 RSC.
- J. Yin, Y. Hua and J. Yoon, Chem. Soc. Rev., 2015, 44, 4619–4644 RSC.
- T. D. Ashton, K. A. Jolliffe and F. M. Pfeffer, Chem. Soc. Rev., 2015, 44, 4547–4595 RSC.
- L. Li, Y. Shen, Y. H. Zhao, L. Mu, X. Zeng, R. Carl and G. Wei, Sens. Actuators, B, 2016, 226, 279–288 CrossRef CAS.
- X. Li, Y. Yin, J. Deng, H. Zhong, J. Tang, Z. Chen, L. Yang and L. J. Ma, Talanta, 2016, 154, 329–334 CrossRef CAS PubMed.
- L. He, C. Liu and J. H. Xin, Sens. Actuators, B, 2015, 213, 181–187 CrossRef CAS.
- Z. Yang, M. She, B. Yin, L. Hao, M. Obst, P. Liu and J. Li, Anal. Chim. Acta, 2015, 868, 53–59 CrossRef CAS PubMed.
- Y. Chen, Q. Lv, Z. Liu and Q. Fang, Inorg. Chem. Commun., 2015, 52, 38–40 CrossRef CAS.
- X. Fang, S. Zhang, G. Zhao, W. Zhang, J. Xu, A. Ren, C. Wu and W. Yang, Dyes Pigm., 2014, 101, 58–66 CrossRef CAS.
- P. S. Hariharan, N. Hari and S. P. Anthony, Inorg. Chem. Commun., 2014, 48, 1–4 CrossRef CAS.
- J. Y. Noh, S. Kim, I. H. Hwang, G. Y. Lee, J. Kang, S. H. Kim, J. Min, S. Park, C. Kim and J. Kim, Dyes Pigm., 2013, 99, 1016–1021 CrossRef CAS.
- M. Yu, R. Yuan, C. Shi, W. Zhou, L. Wei and Z. Li, Dyes Pigm., 2013, 99, 887–894 CrossRef CAS.
- S. Goswami, S. Maity, A. K. Das and A. C. Maity, Tetrahedron Lett., 2013, 54, 6631–6634 CrossRef CAS.
- Y. Chen, Y. Mi, Q. Xie, J. Xiang, H. Fan, X. Luo and S. Xia, Anal. Methods, 2013, 5, 4818–4823 RSC.
- T. Cheng, T. Wang, W. Zhu, Y. Yang, B. Zeng, Y. Xu and X. Qian, Chem. Commun., 2011, 47, 3915–3917 RSC.
- Y. Liu, Y. Sun, J. Du, X. Lv, Y. Zhao, M. Chen, P. Wang and W. Guo, Org. Biomol. Chem., 2011, 9, 432–437 CAS.
- L. Xu, Y. Xu, W. Zhu, B. Zeng, C. Yang, B. Wu and X. Qian, Org. Biomol. Chem., 2011, 9, 8284–8287 CAS.
- R. Uauy, M. Olivares and M. Gonzalez, Am. J. Clin. Nutr., 1998, 67, 952S–959S CAS.
- L. M. Gaetke and C. K. Chow, Toxicology, 2003, 189, 147–163 CrossRef CAS PubMed.
- Y. H. Hung, A. I. Bush and R. A. Cherny, J. Biol. Inorg. Chem., 2010, 15, 61–76 CrossRef CAS PubMed.
- J. C. Lee, H. B. Gray and J. R. Winkler, J. Am. Chem. Soc., 2008, 130, 6898–6899 CrossRef PubMed.
- A. Jajoo, A. Sahay, P. Singh, S. Mathur, S. K. Zharmukhamedov, V. V. Klimov, S. I. Allakhverdiev and S. Bharti, Photosynth. Res., 2008, 97, 177–184 CrossRef CAS PubMed.
- E. L. Greene, G. Farell, S. H. Yu, T. Matthews, V. Kumar and J. C. Lieske, Urol. Res., 2005, 33, 340–348 CrossRef CAS PubMed.
- F. T. Borges, Y. M. Michelacci, J. A. K. Aguiar, M. A. Dalboni, A. S. Garofalo and N. Schor, Kidney Int., 2005, 68, 1630–1642 CrossRef CAS PubMed.
- M. Ronaghi, S. Karamohamed, B. Pettersson, M. Uhlen and P. Nyren, Anal. Biochem., 1996, 242, 84–89 CrossRef CAS PubMed.
- A. E. Timms, Y. Zhang, R. G. G. Russell and M. A. Brown, Rheumatology, 2002, 41, 725–729 CrossRef CAS PubMed.
- M. Doherty, C. Becher, M. Regan, A. Jones and J. Ledingham, Ann. Rheum. Dis., 1996, 55, 432–436 CrossRef CAS PubMed.
- S. Zhang, Q. Wang, G. Tian and H. Ge, Mater. Lett., 2014, 115, 233–236 CrossRef CAS.
- M. Hu and G. Feng, Chem. Commun., 2012, 48, 6951–6953 RSC.
- L. Tang, J. Park, H. J. Kim, Y. Kim, S. J. Kim, J. Chin and K. M. Kim, J. Am. Chem. Soc., 2008, 130, 12606–12607 CrossRef CAS PubMed.
- J. Qiang, C. Chang, Z. Zhu, T. Wei, W. Yu, F. Wang, J. Yin, Y. Wang, W. Zhang, J. Xie and X. Chen, Sens. Actuators, B, 2016, 233, 591–598 CrossRef CAS.
- G. Wang, H. Chen, Y. Chen and N. Fu, Sens. Actuators, B, 2016, 233, 550–558 CrossRef CAS.
- C. Zhao, B. Liu, X. Bi, D. Liu, C. Pan, L. Wang and Y. Pang, Sens. Actuators, B, 2016, 229, 131–137 CrossRef CAS.
- Z. S. Qian, L. J. Chai, Y. Y. Huang, C. Tang, J. J. Shen, J. R. Chen and H. Feng, Biosens. Bioelectron., 2015, 68, 675–680 CrossRef CAS PubMed.
- W. Yu, J. Qiang, J. Yin, S. Kambam, F. Wang, Y. Wang and X. Chen, Org. Lett., 2014, 16, 2220–2223 CrossRef CAS PubMed.
- L. Tang, P. Zhou, Z. Huang, J. Zhao and M. Cai, Tetrahedron Lett., 2013, 54, 5948–5952 CrossRef CAS.
- X. J. Zhao and C. Z. Huang, Biosens. Bioelectron., 2011, 30, 282–286 CrossRef CAS PubMed.
- L. Tang, M. Liu, F. Li and R. Nandhakumar, J. Fluoresc., 2011, 21, 701–705 CrossRef CAS PubMed.
- G. Wang, H. Zhu, Y. Lin, Y. Chen and N. Fu, Sens. Actuators, B, 2015, 206, 624–629 CrossRef CAS.
- L. Tang, D. Wu, X. Wen, X. Dai and K. Zhong, Tetrahedron, 2014, 70, 9118–9124 CrossRef CAS.
- C. He, X. Qian, Y. Xu, C. Yang, L. Yin and W. Zhu, Dalton Trans., 2011, 40, 1034–1037 RSC.
- S. Banerjee, R. K. Das and U. Maitra, J. Mater. Chem., 2009, 19, 6649–6687 RSC.
- R. Shen, D. Liu, C. Hou, J. Cheng and D. Bai, Anal. Methods, 2016, 8, 83–88 RSC.
- J. Huang, Y. Xua and X. Qian, Dalton Trans., 2014, 43, 5983–5989 RSC.
- R. Alam, T. Mistri, A. Katarkar, K. Chaudhuri, S. K. Mandal, A. R. K. Bukhsh, K. K. Das and M. Ali, Analyst, 2014, 139, 4022–4030 RSC.
- Z. Dong, X. Le, P. Zhou, C. Dong and J. Ma, New J. Chem., 2014, 38, 1802–1808 RSC.
- Y. H. Lau, P. J. Rutledge, M. Watkinson and M. H. Todd, Chem. Soc. Rev., 2011, 40, 2848–2866 RSC.
- J. Hu, J. R. Lu and Y. Ju, Chem.–Asian J., 2011, 6, 2636–2647 CrossRef CAS PubMed.
- J. Wu, Y. Gao, J. Lu, J. Hu and Y. Ju, Sens. Actuators, B, 2015, 206, 516–523 CrossRef CAS.
- Y. M. Zhang, Y. Chen, Z. Q. Li, N. Li and Y. Liu, Bioorg. Med. Chem., 2010, 18, 1415–1420 CrossRef CAS PubMed.
- H. Du, R. A. Fuh, J. Li, A. Corkan and J. S. Lindsey, Photochem. Photobiol., 1998, 68, 141–142 CAS.
- Y. Qian, L. Cao, C. Jia, P. O. Boamah, Q. Yang, C. Liu, Y. Huang and Q. Zhang, RSC. Adv., 2015, 5, 77965–77972 RSC.
- J. R. Lin, C. J. Chu, P. Venkatesan and S. P. Wu, Sens. Actuators, B, 2015, 207, 563–570 CrossRef CAS.
- E. Hao, T. Meng, M. Zhang, W. Pang, Y. Zhou and L. Jiao, J. Phys. Chem. A, 2011, 115, 8234–8241 CrossRef CAS PubMed.
- B. Pedras, V. Rosa, R. Welter, C. Lodeiro and T. Avilés, Inorg. Chim. Acta, 2012, 381, 143–149 CrossRef CAS.
Footnote |
† Electronic supplementary information (ESI) available: Experimental and spectroscopic data. See DOI: 10.1039/c7ra01620d |
|
This journal is © The Royal Society of Chemistry 2017 |
Click here to see how this site uses Cookies. View our privacy policy here.