DOI:
10.1039/C7RA00846E
(Paper)
RSC Adv., 2017,
7, 16916-16923
Benzimidazole derivative fluorescent probe for cascade recognition of phosphate and iron ions in aqueous medium and its logic gate behavior†
Received
20th January 2017
, Accepted 4th March 2017
First published on 17th March 2017
Abstract
A fluorescent probe based on benzimidazole was synthesized, and its cascade recognition of PO43− and Fe3+ ions was investigated using spectroscopic techniques. This probe showed a highly sensitive and selective response towards PO43− and Fe3+ relative to other anions and metal ions. Moreover, the resulting [probe–PO43−] complex exhibited a turn-off response toward Fe3+ ion, leading to the fabrication of an INHIBIT logic gate using PO43− and Fe3+ ions as inputs and the emission of the probe as output. This research may enrich the field of multi-functional chemosensors in natural products.
1. Introduction
Ionic recognition has drawn great attention in the past decades due to its crucial role in biological, environmental, and chemical fields.1–5 Although many selective chemosensors have been reported, multi-functional chemosensors that can accomplish cascade recognition by detecting a second analyte based on a coordination complex of a host molecule and the first analyte are relatively rare.6,7 Therefore, the development of recyclable, cost effective, and high performance sensors for cascade detection is of great interest.
Among the various anions, phosphates, including inorganic species (H2PO4−, HPO42−, PO43−, PPi) and their derivatives, such as nucleoside polyphosphates (ATP, ADP, and AMP), play a key role in life processes, such as energy storage,8 gene building9 and information transfer.10 However, the adverse effects of excess phosphate in blood also cause some diseases, such as hyperphosphatemia and cardiovascular complications resulting from the development of metastatic calcifications at the cardiac level, and increased morbidity and mortality.11,12 Considerable effort has focused on designing new chemosensors for the detection of phosphate ions and their derivatives in the last few decades.13–15 Though numerous materials have been developed to sense phosphate ions, most use metal ion complexes for sensing. For example, Tobey and Anslyn16 synthesized a copper ion complex for phosphate, and Bartoli and coworkers17 synthesized mono- and dinuclear fluorescent zinc(II) complexes for di- and triphosphate. Furthermore, many of the sensors reported require an organic solvent as the sensing medium or are easily affected by hydrogen phosphate, dihydrogen phosphate, and pyrophosphate. For example, Wu and coworkers18 designed two complexes as novel phosphate ion probes, which detected phosphate in DMSO/HEPES (8
:
2, v/v) buffer, Azath and coworkers19 developed a colorimetric sensor for phosphate and pyrophosphate anions, and Liao and coworkers20 developed a novel chemosensor for hydrogen phosphate and dihydrogen phosphate.
Iron is the most abundant transition metal ion in humans and other mammals, and plays important roles in various biological systems.21,22 However, an imbalanced iron concentration is known to cause severe deleterious effects. For example, an excess accumulation of iron can cause clinical deterioration and often death in patients with severe forms of thalassemia.23 Therefore, the detection of trace amounts of iron is of critical interest. In recent years, several fluorescent probes for iron ions with high selectivity and sensitivity have been reported.24 However, many of the reported fluorescent probes need either an organic solvent as the sensing medium; for example, in detecting Fe3+ in H2O/DMSO (1
:
1, v/v) solutions25 and in H2O/ACN (1
:
1, v/v) solutions.26
Though several studies on PO43− and Fe3+ ion recognition have been reported, there has been no report of a cascade recognition system that can detect PO43− ions first, followed by Fe3+ ions. Herein, we exploited a fluorescent probe, 1, containing an amide H-bonding donor and benzimidazole with better fluorescence properties. Binding with PO43− led to the fluorescence of probe 1 switching on. Due to the high binding ability of Fe3+ toward PO43−, Fe3+ can snatch PO43− from the [1–PO43−] complex to form a stable FePO4 complex and fully restore the emission, which can be applied to the further detection of Fe3+. Interestingly, using PO43− and Fe3+ as chemical inputs and the fluorescence intensity at 409 nm as output, probe 1 can be used to mimic an INHIBIT (INH) logic gate.
2. Experimental
2.1 Materials and general methods
Deionized water was used throughout the experiment. All reagents and solvents were obtained from commercial sources and were of analytical grade. Solvents were dried according to standard procedures. The salts and solvents were purchased from Sinopharm Chemical Reagent Co., Ltd (Shanghai, China) or Alfa-Aesar Chemical Co. (USA). NMR spectra of 1,4-benzenedi-[3-(1-pentylbenzimidazole)]-acetylaniline dichloride (1) were recorded in DMSO-d6 solution on a Bruker Avance 600 MHz spectrometer. 1H chemical shifts were reported in ppm downfield from tetramethylsilane (TMS), with the solvent resonances used as internal standards, and coupling constants (J) are given in Hz. Mass spectra were recorded on a Waters Xevo UPLC/G2-QTof MS instrument equipped with an electrospray (ESI) ion source. Infrared spectra were performed on a Nicolet AVATAR-750 FTIR spectrometer and samples were prepared as KBr pellets. Melting points were determined using an MPA100 Optimelt Automated Melting Point System and uncorrected. All pH measurements were prepared with a Sartorius basic pH-meter PB-10. UV absorption spectra were determined in 4-(2-hydroxyethyl)-1-piperazine-ethanesulfonic acid (HEPES) solution using a Pgeneral TU-1901 UV-vis spectrophotometer. Fluorescence spectra were recorded on a PerkinElmer LS 55 fluorescence spectrometer.
2.2 General procedure for spectroscopic measurements
All metal ion and anion detections were operated at pH 7.4, maintained with HEPES buffer (10 mM). The 10 μM stock solution of probe 1 was prepared in HEPES. The working solutions of probe 1 were freshly prepared by diluting the concentrated stock solution to the desired concentration prior to spectroscopic measurements. Anion solutions (0.1 M) were obtained from the tetrabutylammonium salt of I−, Cl−, Br−, F−, AcO−, H2PO4−, NO3−, CO32−, HCO3−, SO42−, NO2−, HPO42−, BrO3−, SO32−, HSO3−, SCN−, and PO43−. Metal ion solutions (0.1 M) were obtained from NaCl, KBr, Mg(NO3)2, CaCl2, Cr(NO3)3, FeSO4, Co(NO3)2, NiCl2, Cu(NO3)2, Zn(NO3)2, AgNO3, Cd(NO3)2, HgCl2, Pb(NO3)2, Ba(NO3)2, Fe(NO3)3, and Al(NO3)3. Compounds were stored in a vacuum desiccator containing self-indicating silica, and dried fully before use. All fluorescence data were recorded with an excitation of 269 nm and the slit widths of excitation and emission were 10 nm. Fluorescence measurements used titration experiments and the volume added did not exceed 3% of the total. After the solution mixture was shaken for 1 min, new spectra were measured. All experiments were performed at room temperature and conducted in triplicate at least.
2.3 Synthesis of probe 1
The synthetic route to probe 1 is shown in Scheme 1. 1,4-Bis(chloroethylamido)benzene (A) and N-pentyl benzimidazole (B) were synthesized using reported methods.27,28 Characterization results for 1 are given in the ESI.†
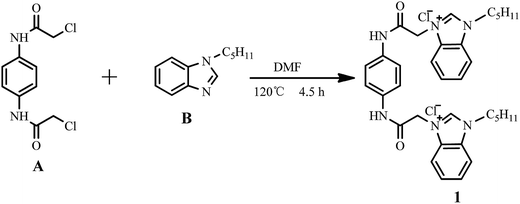 |
| Scheme 1 Synthetic route to 1. | |
A mixture of A (0.783 g, 3.0 mmol), B (1.412 g, 7.5 mmol), and N,N-dimethylformamide (30 mL) in a 100 mL round bottom flask was placed in an oil bath. The reaction mixture was heated until a homogeneous solution was formed. The solution was stirred for 4.5 h at 120 °C under a nitrogen atmosphere. The mixture was cooled to room temperature and a light yellow solid was separated and filtered. The resultant solid was recrystallized from methanol–ethyl acetate (1
:
1, v/v) to afford the pure product, 1.528 g (80.1%) of probe 1, as a light yellow powder. Mp: 244.4–245.9 °C. 1H NMR (DMSO-d6, 600 MHz, ppm): 11.08 (s, 2H), 9.88 (s, 2H), 8.13–8.14 (m, 2H), 8.06–8.08 (m, 2H), 7.68–7.72 (m, 4H), 7.60 (s, 4H), 5.57 (s, 4H), 4.57 (t, J = 7.2 Hz, 4H), 1.90–1.95 (m, 4H), 1.30–1.37 (m, 8H), 0.87 (t, J = 6.9 Hz, 6H). 13C NMR (DMSO, 150 MHz, ppm): 163.73, 143.89, 134.79, 132.31, 131.17, 127.28, 127.06, 120.23, 114.32, 114.20, 79.68, 79.46, 79.24, 49.53, 47.22, 28.72, 28.27, 22.03, 14.23. Mass (ESI-MS): [M − 2Cl]2+, calcd 283.3676, found 283.3718. FTIR (KBr) ν (cm−1): 3406, 3142, 3035, 2959, 2934, 2862, 1687, 1588, 1566, 1513, 1486, 1309 and 751.
3. Results and discussion
3.1 Anion sensing studies using probe 1
The availability of probe 1 as a highly selective probe for PO43− was investigated using UV-vis absorbance spectra and fluorescence spectra of probe 1 after the addition of other representative anions (I−, Cl−, Br−, CO32−, HCO3−, SO42−, NO2−, AcO−, H2PO4−, HPO42−, BrO3−, NO3−, SO32−, HSO3−, F−, and SCN−). The UV-vis absorbance spectra (Fig. S5†) showed a decrease in absorption intensity at 269 nm soon after PO43− addition, while other anions did not induce significant changes. Furthermore, as shown in Fig. 1, only PO43− led to an approx. three-fold increase in intensity at 409 nm in the fluorescence spectrum, with a noticeable red shift in emission wavelength observed. Fluorescence emission around 373 nm was not apparent, and only slight quenching was detected, for other anions, indicating the selective sensing behavior of probe 1 toward PO43− ions. These results indicated that probe 1 was highly selective toward PO43− and might be a potential fluorescent probe for PO43− in aqueous solution. Moreover, when L-tryptophan was dissolved in aqueous solution as a reference standard, the fluorescence quantum yield of the [1–PO43−] complex was 0.2%, which was higher than that of probe 1 (0.03%). This indicated that the binding of probe 1 and PO43− could improve the fluorescence quantum yield.
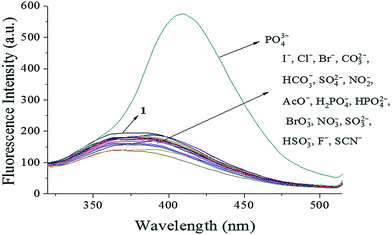 |
| Fig. 1 Fluorescence spectra of probe 1 (10 μM) upon addition of different anions (1.0 equiv.) in HEPES buffer (10 mM, pH 7.4, 25 °C) solution (λex = 269 nm, slit = 10 nm). | |
3.2 Anti-interference of 1 in PO43− detection
To further gauge the increased antidisturbance performance of probe 1 toward PO43− compared with other anions, competition experiments were carried out by adding 1.0 equiv. PO43− to solutions of probe 1 (10 μM) in the presence of 5.0 equiv. of other anions (I−, Cl−, Br−, CO32−, HCO3−, SO42−, NO2−, AcO−, H2PO4−, HPO42−, BrO3−, NO3−, SO32−, HSO3−, F−, and SCN−), and fluorescence spectra were recorded, as shown in Fig. 2. The added competing ions had a negligible influence on the fluorescence maxima of the [1–PO43−] solutions, which indicated the excellent antidisturbance performance of probe 1 in monitoring PO43−.
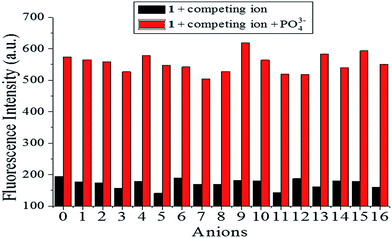 |
| Fig. 2 Fluorescence intensity of probe 1 (10 μM) upon addition of various anions (5.0 equiv.) and PO43− (1.0 equiv.) in HEPES buffer (10 mM, pH 7.4, 25 °C) solution (λex = 269 nm, slit = 10 nm). (0 is a blank, 1–16 respectively represent (1) I−, (2) Cl−, (3) Br−, (4) CO32−, (5) HCO3−, (6) SO42−, (7) NO2−, (8) AcO−, (9) H2PO4−, (10) HPO42−, (11) BrO3−, (12) NO3−, (13) SO32−, (14) HSO3−, (15) F−, (16) SCN−.) | |
3.3 Fluorescent titration of PO43−
To investigate the PO43− ion binding ability of probe 1, fluorescence titration of probe 1 with varying PO43− concentration (0–1.5 equiv.) was carried out. As shown in Fig. 3, as the PO43− concentration increased, at λex = 269 nm the fluorescence intensity increased gradually, accompanied by a red shift and narrowing. Using 1.0 equiv. of PO43− enhanced the emission of probe 1 significantly in the fluorescence titration data due to formation of the [1–PO43−] complex. Fluorescence intensity has a linear response toward PO43− in the PO43− concentration range of 0–1.0 μM, with the linear equation found to be Y = 161.8002 + 62.6713X (linearly dependent coefficient: R2 = 0.9989), as shown in Fig. 4. The detection limit derived from the fluorescent titration was 0.60 nM with 3σ/slope.29 The results suggested that probe 1 detected PO43− qualitatively with high sensitivity. Furthermore, the binding constant of probe 1 with PO43−, which was obtained from fluorescence titration data using the Benesi–Hildebrand equation,30,31 was 2.3 × 105 M−1, as shown in Fig. 5.
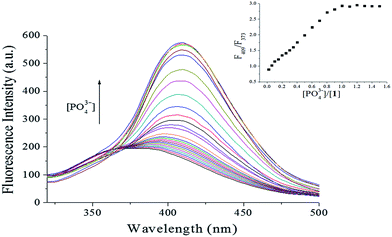 |
| Fig. 3 Fluorescence intensity changes of 1 (10 μM) at various PO43− concentrations (0–1.5 equiv.) in HEPES buffer (10 mM, pH 7.4, 25 °C) solution (λex = 269 nm, slit = 10 nm). Insets: plot of fluorescence intensity ratio (F409/F373). | |
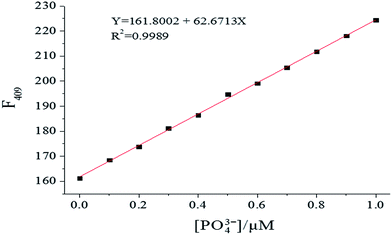 |
| Fig. 4 Fluorescence intensity changes of 1 (10 μM) upon gradual addition of PO43− in HEPES buffer (10 mM, pH 7.4, 25 °C) solution (λex = 269 nm, slit = 10 nm). | |
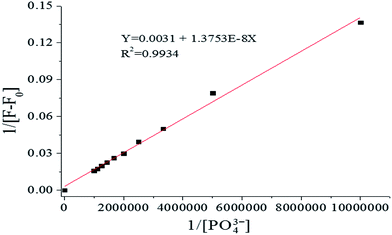 |
| Fig. 5 Benesi–Hildebrand plot from fluorescence titration data of 1 (10 μM) in HEPES buffer (10 mM, pH 7.4, 25 °C) solution (λex = 269 nm, slit = 10 nm). | |
3.4 Effect of pH
To eliminate disturbance from protonation or deprotonation of the fluorophore during detection, the effect of pH on the fluorescence signal of probe 1 in the absence and presence of PO43− was studied. As shown in Fig. 6, with increasing pH from 2.0 to 5.9, the fluorescence intensities almost overlapped, with the dominant forms of phosphate anions likely to be H2PO4− and HPO42−.32,33 Taking the changes in the fluorescence spectra into consideration, probe 1 showed high selectivity for PO43− over other anions. Therefore, the combination of probe 1 and PO43− was very weak in acidic environments. From pH 5.9 to 6.5, the results were very different, showing a rapid increase in the fluorescence emission of probe 1 in with the presence of 1.0 equiv. PO43−. However, strong fluorescence was observed from the solution of probe 1 with PO43− in the pH range 6.5–10.1, which implied the potential application of probe 1 at environmental and physiological pH values.
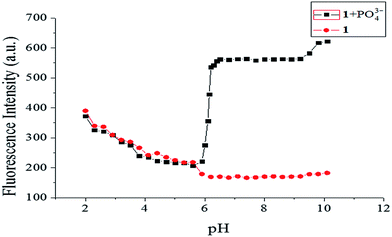 |
| Fig. 6 Fluorescence intensity of probe 1 (10 μM) at different pH values in the absence/presence of PO43− (1.0 equiv.). | |
3.5 Time-dependence of detection
Time is an important parameter for reaction-based probes. The time-dependent fluorescence of probe 1 (10 μM) using 1.0 equiv. of PO43− was studied by monitoring the fluorescence intensity vs. time, as shown in Fig. 7. Upon addition of PO43− to the solution of probe 1, the fluorescence intensity increased rapidly, reaching a maximum within 10 s, and then approximately leveling off, while that of the probe 1 solution alone was stable at a very low level. These results indicated that probe 1 was sensitive and reliable, and that PO43− detection can be completed within minutes.
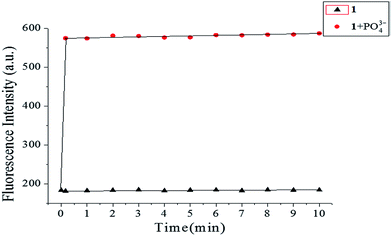 |
| Fig. 7 Time-dependent fluorescence intensity of free probe 1 (10 μM) and probe 1 (10 μM) with 1.0 equiv. of PO43− in HEPES buffer (10 mM, pH 7.4, 25 °C) solution. | |
3.6 Mechanism for PO43− sensing using 1
To confirm the stoichiometry of binding between probe 1 and PO43− ions, Job's plots analysis was carried out. As shown in Fig. 8, the maximal increment of fluorescence intensity between 1 and [1–PO43−] appeared at a PO43− molar fraction of 0.5. According to the equation n = x/(1 − x),34 in which x is the molar fraction of PO43−, corresponding to the maximal fluorescence change and n is the binding stoichiometry, 0.5 indicated a binding stoichiometry for 1 and PO43− of 1
:
1.
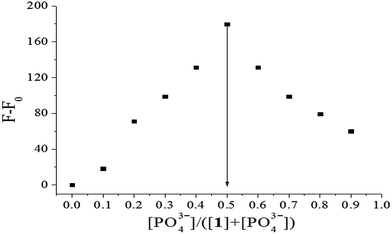 |
| Fig. 8 Job's plot of 1 (forms 1 : 1 complex) in HEPES buffer (10 mM, pH 7.4, 25 °C) solution. F0 and F: fluorescence maxima before and after addition of PO43−, respectively (total concentration of 1 and PO43− is 0.1 μM, λex = 269 nm). | |
This 1
:
1 stoichiometry was further confirmed by ESI-MS results. A series of peaks at m/z 679.5204 corresponding to [12+ + PO43− + 4H+ + H2O]3+, 701.4925 corresponding to [12+ + PO43− + 3H+ + H2O + Na+]3+, 715.3033 corresponding to [12+ + PO43− + 4H+ + H2O + Cl−]2+, 737.2858 corresponding to [12+ + PO43− + 4H+ + H2O + Cl− + Na+]3+, 759.2678 corresponding to [12+ + PO43− + 3H+ + H2O + Cl− + 2Na+]3+, and 781.2493 corresponding to [12+ + PO43− + 2H+ + H2O + Cl− + 3Na+]3+ in ESI-MS corresponded with a strong 1
:
1 complex formed between probe 1 and PO43− anion (Fig. S6†). Therefore, the significant fluorescence intensity enhancement could be attributed to hydrogen bonding interactions between PO43− and probe 1 in which oxygen atoms of PO43− coordinate to the NH proton of amide and CH proton of imidazole. Moreover, hydrogen bonding interactions between amide NH protons and imidazole CH proton in the probe with oxygen atoms in PO43− was precedented14,20,35 and PO43− formed three-coordinate complexes.19
The binding ability of probe 1 toward PO43− was investigated using 1H NMR titration experiments. Fig. 9 shows the partial 1H NMR spectra of the interaction of probe 1 and PO43−. Free probe 1 exhibited two singlet peaks at 11.08 and 9.88 ppm in DMSO-d6 in the far downfield region, attributed to amide Ha and imidazole Hc protons in probe 1, respectively. With increasing of PO43− concentrations, the singlet of Ha disappeared gradually and the signal of Hc broadened significantly through strong intramolecular hydrogen-bonding interactions in the probe and anion-ligand coordination between probe 1 and PO43−. The disappearance of Ha was also caused by the acidity of NH, which resulted in H–D exchange in D2O. Meanwhile, a small and definite upfield shift (0.12 ppm) for the imidazole Hc signal was observed. A small yet definite upfield shift for the Hd, He, and Hf signals from the aromatic units in the benzimidazolium cation occurred, from 7.72–8.14 to 7.70–8.11 ppm. This evidence showed that the high affinity of probe 1 for phosphate ions among other anions was attributed to high negative charges and the oxyacid tetrahedron that binds the coordinate complex, intermolecular hydrogen bonding interactions36 with amide Ha and imidazole Hc, and the bis-cation was going to interact with the tris-anion through ion pairing with the anion between the two benzimidazolium units to form new host–guest complexes.
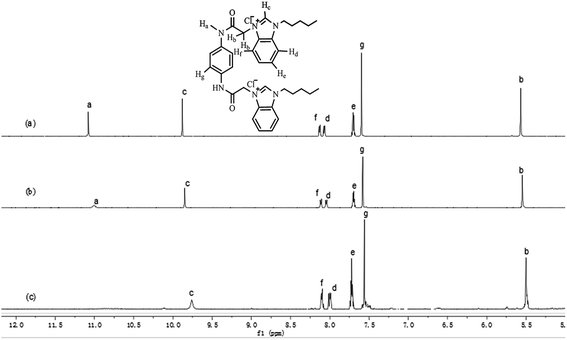 |
| Fig. 9 Partial 1H NMR spectra of probe 1 (10 μM) in DMSO-d6 after addition of PO43−. (a) 1; (b) 1 + 0.5 equiv.; (c) 1 + 1.0 equiv. | |
Based on the Job's plot, ESI-MS spectra, and 1H NMR titration experiment results, a plausible mechanism for anion sensing is shown in Scheme 2. The energy minimized structure also shows that the amide NH proton and imidazole CH proton in [1–PO43−] bind to phosphate through strong hydrogen bonds. From the optimized geometry of the complex of probe 1 in Fig. 10, it was evident that the guest was strongly bound in the cleft through several hydrogen bonding interactions with both oxygen atoms of PO43−, and amide and imidazole protons. Both the amide and imidazole protons form hydrogen bonds through C–H+⋯O− and N–H+⋯O− bonds.
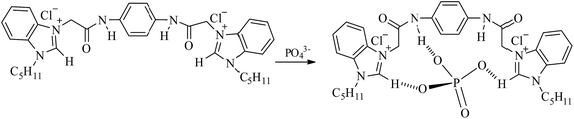 |
| Scheme 2 Proposed binding mechanism between probe 1 and PO43−. | |
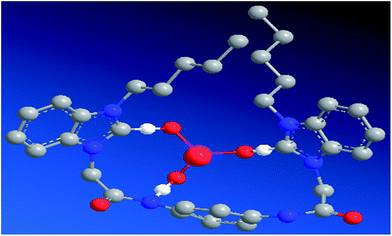 |
| Fig. 10 Energy minimized structure of [1–PO43−]; carbon atoms are gray, oxygen atoms are red, nitrogen atoms are blue, phosphorus atoms are pink, and hydrogen atoms are omitted for clarity. | |
3.7 Cascade recognition of [1–PO43−] toward Fe3+
Iron is one of the most abundant and versatile transition metals, and plays various important roles in many biochemical processes. There is increasing evidence that neither iron deficiencies nor excess iron induce biological disorders in the human body. Therefore, the development Fe3+-selective probes with short response time, low detection limit, and low cytotoxicity is highly desirable.22 After selective recognition with a turn-on response, 17 metal ions (Al3+, Zn2+, Ag+, Ca2+, Mg2+, Fe3+, Fe2+, Hg2+, Pb2+, Na+, Ba2+, Ni2+, K+, Cu2+, Cr3+, Cd2+, and Co2+) were utilized to study the cascade recognition abilities of [1–PO43−] using UV-vis absorbance spectra and fluorescence spectroscopy in HEPES buffer (pH 7.4) solution. Complex [1–PO43−] (10 μM) was treated with 1.0 equiv. of each of the 17 metal ions, as shown in Fig. 11. Of the metal ions, only Fe3+ led to a noticeable increase in absorbance, a color change from colorless to light yellow, and precipitate formation after standing for 3 min, as observed by the naked eye (inset of Fig. 11). The other metal ions did not result in significant absorption changes. The selectivity of [1–PO43−] toward Fe3+ was further confirmed by fluorescence spectra. As shown in Fig. 12, only 1.0 equiv. Fe3+ restored emission completely. In comparison, the addition of 1.0 equiv. of the other metal ions resulted in small changes. Fe3+ was believed to snatch PO43− to form a stable FePO4 complex due to strong electrostatic forces, which resulted in the release of free probe 1. Apparently, the [1–PO43−] complex can serve as a fluorescent probe in the cascade recognition, enabling its potential use in bioanalysis.
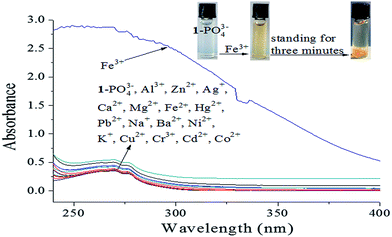 |
| Fig. 11 UV-vis spectra of [1–PO43−] (10 μM) upon the addition of different metal ions (1.0 equiv.) in HEPES buffer (10 mM, pH 7.4, 25 °C) solution. | |
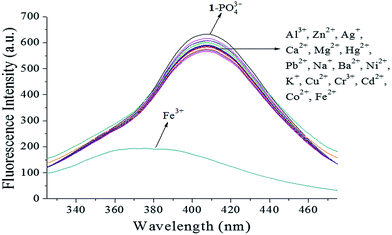 |
| Fig. 12 Fluorescence spectra of [1–PO43−] (10 μM) upon the addition of different metal ions (1.0 equiv.) in HEPES buffer (10 mM, pH 7.4, 25 °C) solution (λex = 269 nm, slit = 10 nm). | |
3.8 Anti-interference of [1–PO43−] in Fe3+ detection
The sensing properties of fluorescence probe [1–PO43−] toward Fe3+ detection were verified using competitive experiments, which were conducted by adding 1.0 equiv. Fe3+ to [1–PO43−] solution in the presence of 5.0 equiv. of other metal ions (Al3+, Zn2+, Ag+, Ca2+, Mg2+, Fe2+, Hg2+, Pb2+, Na+, Ba2+, Ni2+, K+, Cu2+, Cr3+, Cd2+, and Co2+). As shown in Fig. 13, the added competing ions had a negligible influence on the fluorescence maxima of [1–PO43−] solutions in Fe3+ solution, which indicated the excellent anti-disturbance performance of [1–PO43−] for monitoring Fe3+.
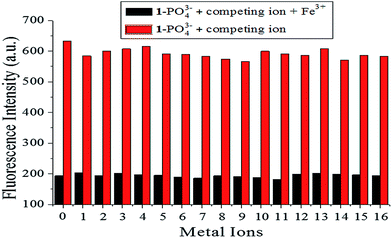 |
| Fig. 13 Fluorescence intensity of probe [1–PO43−] (10 μM) upon addition of various metal ions (5.0 equiv.) and Fe3+ (1.0 equiv.) in HEPES buffer (10 mM, pH 7.4, 25 °C) solution (λex = 269 nm, slit = 10 nm). (0 is a blank, 1–16 represent (1) Al3+, (2) Zn2+, (3) Ag+, (4) Ca2+, (5) Mg2+, (6) Fe2+, (7) Hg2+, (8) Pb2+, (9) Na+, (10) Ba2+, (11) Ni2+, (12) K+, (13) Cu2+, (14) Cr3+, (15) Cd2+, and (16) Co2+.) | |
3.9 Fluorescent titration of Fe3+
The sensing ability of [1–PO43−] toward Fe3+ was further determined by fluorescence titration. As shown in Fig. 14, the emission intensity of [1–PO43−] at 409 nm decreased gradually until 1.0 equiv. of Fe3+ was added. The linear response of fluorescence intensity toward Fe3+ occurred in the Fe3+ concentration range 0–1.0 μM, and the linear equation was found to be Y = 569.3283 − 103.8911X (linearly dependent coefficient: R2 = 0.9905), as shown in Fig. 15. The detection limit, evaluated using fluorescent titration, was 0.36 nM, which was lower than the maximum level of Fe3+ (5.4 μM) ions permitted in drinking water by the US Environmental Protection Agency,37 implying that probe [1–PO43−] has great potential for use in the development of probe materials for Fe3+.
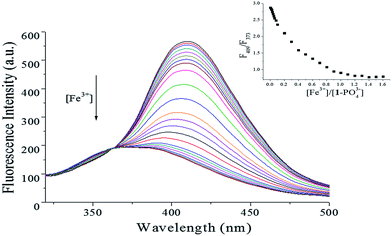 |
| Fig. 14 Fluorescence changes in [1–PO43−] complex (1 : 1, 10 μM) upon addition of Fe3+ (0–1.6 equiv.) in HEPES buffer (10 mM, pH 7.4, 25 °C) solution (λex = 269 nm, slit = 10 nm). Insets: plot of fluorescence intensity ratio (F409/F373). | |
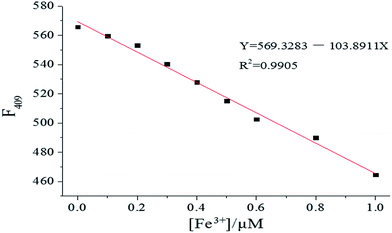 |
| Fig. 15 Fluorescence intensity changes in [1–PO43−] (10 μM) upon gradual addition of Fe3+ in HEPES buffer (10 mM, pH 7.4, 25 °C) solution (λex = 269 nm, slit = 10 nm). | |
3.10 Molecular logic circuits
Molecular logic gates, which perform logical operations by converting inputs into detectable outputs at the molecular level, have become an active research field in supramolecular chemistry and material science because of their promising applications in molecular-scale information technology.38,39 These systems contain binary switches to determine the output state by controlling input conditions. Due to the cascade recognition abilities of probe 1, the logic gate properties of probe 1 were studied using PO43− ions (IN 1) and Fe3+ ions (IN 2) as input signals, and the fluorescence intensity at 409 nm as the output signal. The presence of PO43− and Fe3+ was defined as “1” for input, and the absence of these species was defined as “0”. Similarly, the “turn-on” and “turn-off” of fluorescence were defined as “1” and “0” for the output, respectively. From the perspective of logic functions, the turn-on of 1 (output “1”) can be observed only in the presence of PO43− ions (IN 1 = 1) and in the absence of Fe3+ ions (IN 2 = 0), as shown in Fig. 16. Under other circumstances, output “0” would be afforded due to the weak emission of 1. Therefore, probe 1 can be used as an INHIBIT (INH) logic gate40 toward PO43− ions in the absence of Fe3+ ions by observing emission at 409 nm. The pictorial representation and truth table for the corresponding INH logic gate are shown in Fig. 16b and c, respectively.
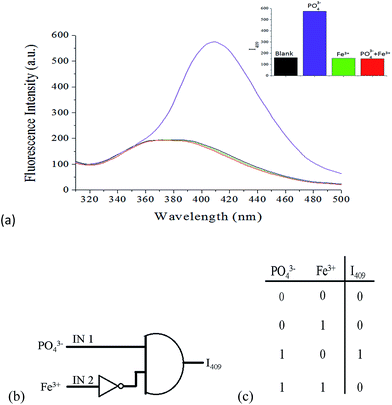 |
| Fig. 16 (a) Fluorescence spectra of probe 1 (10 μM) with the addition of 1.0 equiv. PO43− ions or 1.0 equiv. Fe3+ ions, and the addition of 1.0 equiv. Fe3+ ion after 1.0 equiv. PO43− ion; inset: fluorescence intensity at 409 nm. (b) Conventional gate notation and (c) truth table for the INH logic gate; PO43− and Fe3+ ions are inputs to the system, and I409 is the output signal. | |
4. Conclusions
In summary, we designed and synthesized a fluorescent probe (1) for PO43− with excellent overall performance, such as 100% aqueous solution working medium, high selectivity and sensitivity, low detection limits (0.60 nM), strong interference immunity, favorable working pH span, and a rapid response time (10 s). A Job's plot and MS analysis showed only the formation of a 1/PO43− complex with 1
:
1 stoichiometry. The binding constant of 1/PO43− complex was calculated to be 2.3 × 105 M−1. Interestingly, the resulting [1–PO43−] complex exhibited turn-off response toward Fe3+ ions in 100% aqueous solution, with a detection limit of 0.36 nM. The cascade recognition in the present study was used to construct an INH logic gate, which could inspire a wide range of multi-functional chemosensors based on natural products.
Acknowledgements
This work was financially supported by the Graduate Student Innovation Research Projects of Qiqihar University of China (No. YJSCX2016-ZD08 & 2015-ZD13), the Overseas Scholars Foundation of Scientific and Technical Agency of Heilongjiang province of China (No. LC2011C17), and the National Natural Science Foundation of China (No. 21506106 & 81403067).
References
- K. Dhanunjayarao, V. Mukundam and K. Venkatasubbaiah, Sens. Actuators, B, 2016, 232, 175–180 CrossRef CAS.
- J. B. Chao, Z. Q. Li, Y. B. Zhang, F. J. Huo, C. X. Yin, H. B. Tong and Y. H. Liu, Sens. Actuators, B, 2016, 228, 192–199 CrossRef CAS.
- L. M. Zhao, G. Liu and B. F. Zhang, Spectrochim. Acta, Part A, 2016, 169, 45–49 CrossRef CAS PubMed.
- K. M. Xiong, F. J. Huo, C. X. Yin, Y. Y. Chu, Y. T. Yang, J. B. Chao and A. M. Zheng, Sens. Actuators, B, 2016, 224, 307–314 CrossRef CAS.
- J. Tang, S. Ma, D. Zhang, Y. Q. Liu, Y. F. Zhao and Y. Ye, Sens. Actuators, B, 2016, 236, 109–115 CrossRef CAS.
- G. M. Wang, H. Q. Chen, Y. Q. Chen and N. Y. Fu, Sens. Actuators, B, 2016, 233, 550–558 CrossRef CAS.
- P. Ding, X. Xin, L. L. Zhao, Z. C. Xie, Q. H. Zhang, J. M. Jiao and G. Y. Xu, RSC Adv., 2017, 7, 3051–3058 RSC.
- J. R. Jadhav, M. W. Ahmad and H. S. Kim, Tetrahedron Lett., 2012, 53, 2627–2631 CrossRef CAS.
- Y. W. Wang, S. B. Liu, Y. L. Yang, P. Z. Wang, A. J. Zhang and Y. Peng, ACS Appl. Mater. Interfaces, 2015, 7, 4415–4422 CAS.
- J. Shao, H. Lin and H. K. Lin, Dyes Pigm., 2009, 80, 259–263 CrossRef CAS.
- S. Sen, M. Mukherjee, K. Chakrabarty, I. Hauli, S. K. Mukhopadhyay and P. Chattopadhyay, Org. Biomol. Chem., 2013, 11, 1537–1544 CAS.
- F. Albaaj and A. J. Hutchison, Drugs, 2003, 63, 577–596 CrossRef PubMed.
- C. Lim, M. An, H. Seo, J. H. Huh, A. Pandith, A. Helal and H. S. Kim, Sens. Actuators, B, 2017, 241, 789–799 CrossRef CAS.
- Q. Y. Cao, Y. M. Han, P. S. Yao, W. F. Fu, Y. Xie and J. H. Liu, Tetrahedron Lett., 2014, 55, 248–251 CrossRef CAS.
- A. Kumar and P. S. Pandey, Org. Lett., 2008, 10, 165–168 CrossRef CAS PubMed.
- S. L. Tobey and E. V. Anslyn, Org. Lett., 2003, 5, 2029–2031 CrossRef CAS PubMed.
- F. Bartoli, A. Bencini, A. Garau, C. Giorgi, V. Lippolis, A. Lunghi, F. Totti and B. Valtancoli, Chem.–Eur. J., 2016, 22, 14890–14901 CrossRef CAS PubMed.
- G. F. Wu, Q. L. Xu, L. E. Guo, T. N. Zang, R. Tan, S. T. Tao, J. F. Ji, R. T. Hao, J. F. Zhang and Y. Zhou, Tetrahedron Lett., 2015, 56, 5034–5038 CrossRef CAS.
- I. A. Azath, P. Suresh and K. Pitchumani, Sens. Actuators, B, 2011, 155, 909–914 CrossRef CAS.
- J. H. Liao, C. T. Chen and J. M. Fang, Org. Lett., 2002, 4, 561–564 CrossRef CAS PubMed.
- J. H. Xu, Y. M. Hou, Q. J. Ma, X. F. Wu and X. J. Wei, Spectrochim.
Acta, Part A, 2013, 112, 116–124 CrossRef CAS PubMed.
- Y. M. Liu, J. Y. Zhang, J. X. Ru, X. Yao, Y. Yang, X. H. Li, X. L. Tang, G. L. Zhang and W. S. Liu, Sens. Actuators, B, 2016, 237, 501–508 CrossRef CAS.
- H. M. Chawla and T. Gupta, Tetrahedron Lett., 2015, 56, 793–796 CrossRef CAS.
- Z. G. Wang, P. Long, Y. Y. Feng, C. Q. Qina and W. Feng, RSC Adv., 2017, 7, 2810–2816 RSC.
- J. Liu, Q. Lin, Y. M. Zhang and T. B. Wei, Sci. China: Chem., 2014, 57, 1257–1263 CrossRef CAS.
- T. Nandhini, P. Kaleeswaran and K. Pitchumani, Sens. Actuators, B, 2016, 230, 199–205 CrossRef CAS.
- L. Y. Wang, Y. Zhang, L. M. Ding, J. Liu, B. Zhao, Q. G. Deng and T. Yan, RSC Adv., 2015, 5, 74764–74773 RSC.
- B. Wannalerse, T. Tuntulani and B. Tomapatanaget, Tetrahedron, 2008, 64, 10619–10624 CrossRef CAS.
- B. P. Joshi, J. Park, W. I. Lee and K. Lee, Talanta, 2009, 78, 903–909 CrossRef CAS PubMed.
- H. A. Benesi and J. H. Hildebrand, J. Am. Chem. Soc., 1949, 71, 2703–2707 CrossRef CAS.
- M. J. Yuan, W. D. Zhou, X. F. Liu, M. Zhu, J. B. Li, X. D. Yin, H. Y. Zheng, Z. C. Zuo, C. B. Ouyang, H. B. Liu, Y. L. Li and D. B. Zhu, J. Org. Chem., 2008, 73, 5008–5014 CrossRef CAS PubMed.
- S. S. Zumdahl and D. C. Chemistry, Chemical Principles, Heath and Company, Lexington, MA, 2nd edn, 1995 Search PubMed.
- J. L. Sessler, J. M. Davis, V. Kral, T. Kimbrough and V. Lynch, Org. Biomol. Chem., 2003, 1, 4113–4123 CAS.
- M. M. Hong, X. Y. Lu, Y. H. Chen and D. M. Xu, Sens. Actuators, B, 2016, 232, 28–36 CrossRef CAS.
- Z. C. Xu, S. K. Kim and J. Yoon, Chem. Soc. Rev., 2010, 39, 1457–1466 RSC.
- X. P. Bao, Y. H. Zhou and B. A. Song, Sens. Actuators, B, 2012, 171–172, 550–555 CrossRef CAS.
- C. Yi, W. W. Tian, B. Song, Y. P. Zheng, Z. J. Qi, Q. Qi and Y. M. Sun, J. Lumin., 2013, 141, 15–22 CrossRef CAS.
- J. Choi, J. H. Lee and J. H. Jung, Analyst, 2014, 139, 3866–3870 RSC.
- S. Mardanya, S. Karmakar, S. Das and S. Baitalik, Sens. Actuators, B, 2015, 206, 701–713 CrossRef CAS.
- J. D. Wu, X. Zhao, Y. X. Gao, J. Hu and Y. Ju, Sens. Actuators, B, 2015, 221, 334–340 CrossRef CAS.
Footnote |
† Electronic supplementary information (ESI) available. See DOI: 10.1039/c7ra00846e |
|
This journal is © The Royal Society of Chemistry 2017 |