DOI:
10.1039/C7RA00758B
(Paper)
RSC Adv., 2017,
7, 13347-13352
Highly dispersed Ag/TiO2 via adsorptive self-assembly for bactericidal application
Received
18th January 2017
, Accepted 19th February 2017
First published on 27th February 2017
Abstract
The strong electrostatic adsorption (SEA) technique was used for the preparation of Ag/TiO2 by driving the Ag precursor onto the TiO2 surface. The prepared material was characterized using nitrogen adsorption–desorption techniques, X-ray diffraction, scanning transmission electron microscopy, X-ray photoelectron spectroscopy and inductively-coupled plasma. The results demonstrated that the SEA method could effectively prevent Ag itself from aggregating, and Ag nanoparticles (<2 nm) were formed on the TiO2 surface with a uniform distribution. To prove the feasibility of the effective SEA method, the bactericidal performance of Ag/TiO2 particles for antibacterial packaging was evaluated and the results indicated that the highly dispersed Ag on TiO2 exhibited excellent antimicrobial properties since the particle size and dispersion of Ag nanoparticles have a strong relationship with the inhibition of bacterial growth.
1. Introduction
The antibacterial properties of silver have been widely studied in the literature. Silver ions can lead to denaturation of protein and cell death because of their reaction with nucleophilic amino acid residues in proteins, and attach to sulphydryl, amino, imidazole, phosphate and carboxyl groups of membrane or enzyme proteins.1–3 Another hypothesis of the antibacterial mechanism is that silver ions are in the catalytic activity center that activates water and oxygen in the air to produce hydroxyl free radicals and active oxygen ions (O2−). Active oxygen ions can destroy the reproductive ability of mould in a short time to induce cell death.4–6 Such a mechanism does not need any direct contact between antimicrobial agent and bacteria, because the produced active oxygen ions will automatically diffuse from the bulk metal to the surrounding environment. Nanoparticles exhibit completely new and improved properties based on specific characteristics such as size, distribution and morphology in comparison with their bulk counterparts.7–10 However, it is known that the high surface energy of Ag nanoparticles makes them easily aggregate into big particles, which could deteriorate their unique chemical properties and induce loss of overall performance. To stabilize Ag nanoparticles, an efficient method is to design highly dispersed Ag particles on substrates such as SiO2, Fe2O3, Al2O3, and TiO2.11–18 Especially, TiO2 has received considerable attention due to its good stability, environmentally benignity, safety and broad-spectrum antibiosis.17 Much attention has been focused on combing Ag and TiO2 to improve antimicrobial activity. Recently, nanocomposite food packages, produced by incorporating nanoparticles of Ag and TiO2, have been used in several agricultural products, such as mushrooms and strawberries.18–20 Very recently, it is reported that nano-packaging with Ag/TiO2 had a quite beneficial antimildew effect on rice storage.21 Therefore, Ag/TiO2 nanocomposites show great promise as efficient antibacterials in future.22–26
In practical application, both particle size and silver release are two important characteristics for silver-based functional materials. Ag particles of less than 10 nm are expected and they are more toxic to bacteria including Escherichia coli.27,28 However, it is difficult to produce uniform Ag nanoparticles that can strongly anchor on TiO2 surface by conventional sol–gel and photodeposition methods.29–31 A simple and rational approach to synthesizing highly dispersed, monometallic catalysts on a wide variety of oxide and carbon supports was developed using the strong electrostatic adsorption (SEA) method.32–35 The SEA method is based on an electrostatic mechanism36 in which the hydroxyl groups that populate oxide surfaces become protonated (positively charged) or deprotonated (negatively charged) depending on a characteristic pH value. The particular pH where the hydroxyl groups are neutral is termed the Point of Zero Charge (PZC). According to the theory, the oxides placed in solutions with equilibrium pH values above their PZC would adsorb cations such as silver ammonium ion [Ag(NH3)2]+. These electrostatic interactions can be used to control the complex deposition.
In this work, we addressed the use of SEA method for the preparation of Ag-promoted TiO2 by driving Ag onto TiO2 phase. This was achieved by first measuring the PZC of TiO2 to determine its unique oxide charging parameters. Then the adsorption survey of [Ag(NH3)2]+ on TiO2 at various pH values was conducted. The [Ag(NH3)2]+ should strongly adsorb at high pH over high-PZC material such as TiO2. This information was used to determine the optimal uptake pH for [Ag(NH3)2]+ to adsorb onto the supported TiO2 for Ag/TiO2 preparation. The antibacterial analysis of Ag/TiO2 particles was carried out by minimum inhibitory concentration technique.
2. Experimental section
2.1 PZC measurements and adsorption experiments
The two different pure commercial TiO2 and nano-sized TiO2 (denoted as TiO2-N) were obtained from Nanjing Dingbei biological technology Company, China. To determine their PZC, the materials were weighed out to obtain surface loadings of 1000, 10
000 and 50
000 m2 L−1 for 50 mL of solution. The pH values of each 50 mL deionized water were adjusted in the range of 2–13 by using HCl or NaOH. Then TiO2 was added to the above pH adjusted solutions and shaked for 1 h. Final pH measurements were obtained using a general combination pH electrode. At sufficiently high surface loadings, the plateau of the pH shift plot corresponds to the PZC of the support.37 The PZCs of TiO2 and nano-TiO2 are 6.9 and 7.1 respectively. Preliminary equilibrium adsorption experiments to study [Ag(NH3)2]+ uptake were performed over the pure TiO2 and TiO2-N materials. The pH-adjusted [Ag(NH3)2]+ (solutions 200 ppm Ag) were contacted with the pure oxides at a surface loading of 500 m2 L−1, in excess liquid to prevent large shifts in the solution pH due to the oxide,37 shaken for 1 h, and then ∼5 mL of solution was filtered off to analyze Ag concentration using a Perkin-Elmer Inductively Coupled Plasma-Optical Emission Spectrometer (ICP-OES). Ag uptake can be calculated with difference in concentration between the pre- and post-contacted solutions.
2.2 Materials preparation
Ag promoted TiO2 samples were prepared by SEA as follows: the equilibrium adsorption uptake of [Ag(NH3)2]+ onto TiO2 and TiO2-N was determined as a function of pH from solutions with a fixed metal concentration (200 ppm Ag). Larger samples (1 g) of catalysts were prepared at the pH of maximum uptake, which corresponds to optimal condition for SEA. Shaken 1 h and then the filtered solids were dried overnight and then irradiated with UV light (100 W mercury lamp from Philips) for 1 h. The samples properties are listed in Table 1.
Table 1 Preparation methods and Ag loadings of TiO2 supported with different precursor
Samplesa |
Preparation method |
Surface area (m2 g−1) |
Initial pH |
Final pH |
Uptake (μmol m−2) |
Ag loading (wt%) |
BET surface area 79.3 m2 g−1 for TiO2 and 93.7 m2 g−1 TiO2-N. |
Ag/TiO2 |
SEA |
75.1 |
11.49 |
10.92 |
1.08 |
0.88 |
Ag/TiO2-N |
SEA |
91.3 |
11.49 |
11.01 |
1.03 |
1.01 |
2.3 Physical characterization
X-ray diffraction (XRD) analysis was performed on a Siemens D5000 diffractometer with Cu Kα radiation operating at 30 kV and 40 mA and a scan range of 20–70° 2θ. N2 adsorption–desorption isotherms were measured with a Micromeritics ASAP 2020 automatic adsorption instrument using nitrogen as the analysis gas. The data were analyzed with the Brunauer–Emmett–Teller (BET) equation to calculate the surface area. Scanning Transmission Electron Microscopy (STEM) measurements were carried out on JEM-2010F manufactured by JEOL, USA. A small amount of the catalyst sample was dispersed in isopropanol and sonicated for 10 min. A drop of the sample was then placed onto a carbon-coated copper grid (200 mesh, SPI supplies, USA). The grid supported sample was finally dried in an ultra-infrared lamp until isopropanol evaporated thoroughly. High angle annular dark field (HAADF) imaging was performed at 200 kV and extracting voltage of 4500 V. X-ray photoelectron spectroscopy (XPS) analysis was carried out with a monochromatic Al X-ray source fitted with a charge neutralization coil. Binding energies were referenced to C 1s peak at 285 eV. The vacuum level during the experiments was 10−7 Pa.
2.4 Antibacterial activity
The antibacterial analysis of normal Ag/TiO2 and nano-Ag/TiO2 particles was carried out by minimum inhibitory concentration (MIC) technique and the antibacterial effect of packaging with normal Ag/TiO2 and nano-Ag/TiO2 particles were tested against mould that isolated from aged rice. The packaging was produced using the method of Li Li.21 In a typical process, 25 g of aged rice was placed in a stomacher bag containing 225 mL of 0.85% aseptic physiological saline and pummeled for 5 min. The homogenate was standardized to a concentration of at least 1 × 105 cell per mL using plate count. The Ag/TiO2 particle was dispersed in sterile ultrapure water using ultrasonic vibration, and the suspension was serially diluted into 10, 1, 0.1, 0.01, 0.001 mg L−1. The MIC was determined by spread plate method where 500 μL of antibacterial suspension and 200 μL of bacteria solution were poured and plated onto sterile Rose Bengal Agar (RBA) plate. The plates were then incubated at 28 °C for 48 ± 2 h and the distinct colonies were counted after that.
To test the antibacterial capacity of the two antibacterial packing, a modification of the International Standard ISO 22196:2007 was used. 1 mL mould suspension that prepared according the above method was daubed onto films. After two days, the films were washed by aseptic physiological saline and the antibacterial results were shown by the plate count.
3. Result and discussion
3.1 Preliminary equilibrium adsorption
Fig. 1a displays the pH shifts for [Ag(NH3)2]+ adsorption experiments over TiO2 and TiO2-N. The pH shift curves after adsorption have similar trends as other oxides studied previous.32 It is important to measure the optimum final pH required for maximum [Ag(NH3)2]+ uptake on TiO2 and TiO2-N, which are shown in Fig. 1b. For both of different TiO2 precursors, little [Ag(NH3)2]+ is adsorbed below pH 9, but the adsorption increases rapidly with increasing pH, particularly between pH from 10 to 11.0. The adsorption decrease a little when pH reach 11.5, which is caused by the strong and competition adsorption of another cation at highest pH. The optimum pH final for TiO2 and TiO2-N are around 11, where the maximum uptakes are both around 1.1 μmol m−2. The Ag loading of the samples prepared at the pH of maximum uptake, which corresponds to the optimal condition for SEA is 0.88 wt% and 1.01 wt% respectively (Table 1).
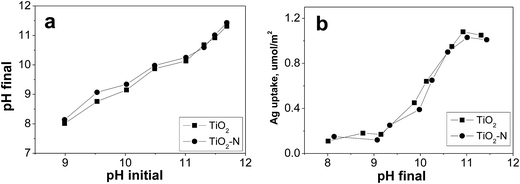 |
| Fig. 1 (a) pH shift with pure oxides-control experiment; [Ag(NH3)2]+ solution pH shifts for TiO2 (∼200 ppm [Ag(NH3)2]+, 500 m2 L−1) (b) Ag uptake on TiO2 versus pH. | |
3.2 Characterization of nanoparticles
Fig. 2 shows STEM images of Ag/TiO2-N and Ag/TiO2 prepared with the SEA method. Fig. 2a displays the STEM micrograph where it can be observed TiO2-N nanocrystals with particle sizes of 20–50 nm. Ag nanoparticles are observed as white spots on light grey TiO2-N, and the Ag nanoparticles are generally spherical in shape.38 The average particle size of Ag calculated from STEM images is quite small (1.8 nm), indicating that the SEA method could homogeneously deposit Ag particles over TiO2-N surface (Fig. 2b). Fig. 2c and d show STEM image and the particle size distribution of Ag/TiO2, which is limited to a narrow range as well, as shown by the values of standard deviation. It is worth noting that the optimal Ag uptake is found to be around 1 μmol m−2 onto both TiO2 and TiO2-N surface (Table 1). According to the BET method, the surface area of TiO2 precursors lost little after deposition using SEA method. The oxide surface is strongly charged and the precursors deposited on individual adsorption sites at the pH of the strongest electrostatic attraction result in uniform dispersion of Ag nanoparticles with no Ag agglomeration. These results suggest that the synthesized nanocomposite is stable in nature.
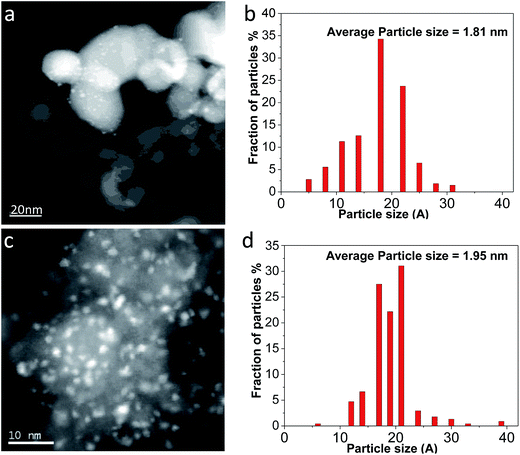 |
| Fig. 2 STEM image of (a) Ag/TiO2-N, (c) Ag/TiO2 and (b) and (d) its particle size distribution. | |
The good Ag dispersion observed locally by STEM was confirmed at global scale using X-ray diffraction (XRD). Fig. 3 shows XRD patterns of TiO2, TiO2-N, Ag/TiO2, Ag/TiO2-N and metal Ag. A typical anatase phase TiO2 crystal is observed from both commercial TiO2 and nano-sized TiO2-N. The mean crystalline size of TiO2-N is evaluated to be around 23 nm based on Scherrer's equation from (101) peak.39 It is agreed with the model TiO2-N used as precursor. The power XRD pattern of Ag (Fig. 3e) exhibits cubic phase with peaks at 38.2°, 44.4°, 64.4° and 77.4° (JCPDS 03-0921). The power XRD pattern of Ag/TiO2 shows the characteristic peaks corresponding to anatase TiO2 phase. However, no characteristic peaks corresponding to Ag phase are observed, which confirms that the Ag particles are very small and highly dispersed on the TiO2 support. It can be explicated that once the particle size is less than 3 nm, the characteristic peaks cannot be detected,33 which is in agreement with the result of STEM. The XRD pattern of the Ag/TiO2-N in Fig. 3d also shows that the Ag phase is well dispersed on support surface, since no peaks can be observed. This is also in agreement with the STEM result reported above. From XRD, it seems that using SEA method to prepare the Ag based antimicrobial agent can lead to very different formulations for the antibacterial active sites affecting antibacterial procedure.
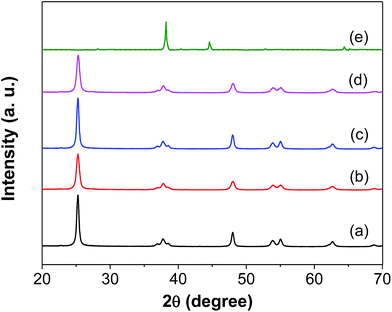 |
| Fig. 3 XRD patterns of (a) TiO2, (b) TiO2-N, (c) Ag/TiO2, (d) Ag/TiO2-N, (e) Ag. | |
The comparison of XPS spectra for Ag 3d3/2 and Ag 3d5/2 regions for samples supported with TiO2 and TiO2-N are provided in Fig. 4. As shown in the XPS, a small amount of Ag element is present. The Ag (3d3/2) and Ag (3d5/2) peaks are found at binding energy of 368.4 and 374.4 eV, respectively, in both of the two samples. In particular, the splitting of the 3d doublet of Ag is 6.0 eV, indicating formation of metallic silver nanoparticles40 on surface of the Ag/TiO2-N. To further study the chemical state of the silver atoms accumulated on the surface, a detailed deconvolution of Ag (3d) peak was also performed. There are three components after deconvolution according to the binding energy of Ag core level for Ag (368.4 eV), Ag2O (367.6 eV) and AgO (367.05 eV). The peak is deconvoluted into two Gaussian components with identical full width at half maximum (FWHM) after a Shirley back-ground subtraction. Based on the deconvolution analysis, it is found that most of the silver atoms accumulated on the surface are in the Ag (metallic) state, while only little silver atoms are in Ag+ chemical states and no silver atoms are in Ag2+. The ratio of Ag+ on the TiO2-N is a little more than that on TiO2, however, no obvious difference was found between the samples using different support. It is explicated that deposition [Ag(NH3)2]+ on TiO2 using SEA after UV irradiation results in different Ag0 and Ag+ composition and while metallic Ag plays a crucial role in electron transfer from TiO2.
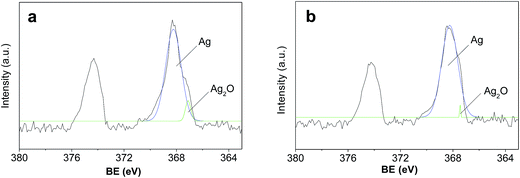 |
| Fig. 4 XPS spectra of (a) Ag/TiO2-N, and (b) Ag/TiO2. | |
3.3 Antibacterial activity
The antibacterial activity of the as-prepared samples and minimum inhibitory concentrations were investigated by measuring the growth inhibition of the mould that isolated from aged rice on Bengal medium solid agar plates. Fig. 5 shows that Ag/TiO2 particles could inhibit the mould growth and have an increase antibacterial effect with increasing the Ag/TiO2 concentration. When the concentration is 1 mg L−1, the inhibition rate of normal-Ag/TiO2 and Ag/TiO2-N is more than 96% and has no significant difference between each other. With higher concentration of particles (10 mg L−1), there is little change of inhibitory effect under the test conditions. So, the minimum inhibitory concentration of 1 mg L−1 is the optimal inhibitory concentration in mold growth. Silver has a broad spectrum of antibacterial activities, especially for the nanosized silver. Nanostructured antibacterial have high surface area-to-volume ratio compared with higher scale counterparts. The silver adsorbed onto TiO2 is nanosized with a narrow size. The normal-Ag/TiO2 and Ag/TiO2-N could release silver ions a long term and generate chemical species reactive to oxygen, form complexes with sulfur, nitrogen and oxygen, which leading to the cell death. Fig. 6a–c show the antibacterial activity of the packaging with Ag/TiO2 particle, packaging with Ag/TiO2-N particle and the normal packaging. The antibacterial activity of two antibacterial packaging can inhibit the mould growth and the total plate count is scare compared with the blank control that normal packaging had not added the antimicrobial agent. It is indicated that silver deposition onto different supports shows almost the same antibacterial performance. The packaging with antibacterial property can be used as a functional material in fruits and vegetables logistics. As we known, most fresh food should been packed for facilitate sales and resistance of the environmental pollution. The antibacterial packaging is efficient for retarding the changes of qualities of food and extend the shelf life. The higher inhibitory of Ag/TiO2 against mould in low concentration may be attributed to the uniformly distributed silver on TiO2 and the size of Ag. The inhibitory effect of Ag/TiO2 particles is related to their shape, state of aggregation and size.41 Silver nanoparticles with a size of about 2 nm are well known for their antibacterial properties because silver ions could migrate and attach to the cell membrane, leading to changes of cell structure, membrane permeability and cellular enzymes, ultimately resulting in the bacteria death.42–44 Ag/TiO2 nanoparticles prepared by SEA method using a strong electrostatic can keep stable releasing of silver with a long term and improve the utilization efficiency of silver and make the silver particles distribute on TiO2 uniformly, thus improving the inhibitory efficiency in attacking and destructing bacterial cell membranes.
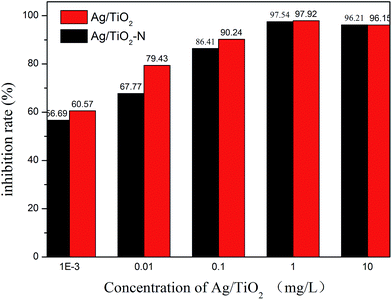 |
| Fig. 5 The antibacterial rate of different concentrations of Ag/TiO2. | |
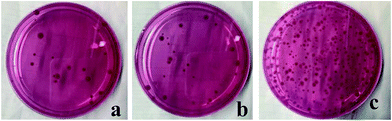 |
| Fig. 6 The antibacterial activity of packaging with Ag/TiO2 (a), Ag/TiO2-N (b) and normal packaging (c) to mould. | |
4. Conclusion
Ag/TiO2 and Ag/TiO2 nanopowder were synthesized by SEA method and well-dispersed Ag particles on TiO2 were obtained during the preparation process. Owing to the strong electrostatic adsorption that exists between the Ag precursor and TiO2, the intrinsic principles for deposition and the strong electrostatic interaction using SEA method prevented sintering and agglomeration of particles during UV reduction, which led to the formation of very uniform and small Ag particles on TiO2. Both of the Ag based antibacterial samples and packaging were proven to be great inhibitory efficiency of mould growth in attacking and destructing bacterial cell membranes. Finally, it is suggested that the use of SEA in the preparation of metal supported materials is a viable way where intimate contact between different metals is desired.
Acknowledgements
This research was supported by the National key research and development program of China (2016YFD0400901), the National Natural Science Foundation of China (Grant No. 31571901), the Natural Science Foundation of Jiangsu Province (BK20141486), Agricultural independent innovation fund of Jiangsu Province (CX(16)1062), Development Program of Jiangsu Province (BK2016386) and the Priority Academic Program Development of Jiangsu Higher Education Institutions.
References
- W. Hou and S. B. Cronin, Adv. Funct. Mater., 2013, 23, 1612–1619 CrossRef CAS.
- T. Møretrø, G. S. Høiby-Pettersen, C. K. Halvorsen and S. Langsrud, Food Control, 2012, 28, 118–121 CrossRef.
- K. B. Holt and A. J. Bard, Biochemistry, 2005, 44, 13214–13223 CrossRef CAS PubMed.
- K. S. Abhijith, R. Sharma, R. Ranjan and M. S. Thakur, Photochem. Photobiol. Sci., 2014, 13, 986–991 CAS.
- Y. Matsumura, K. Yoshikata, S. Kunisaki and T. Tsuchido, Appl. Environ. Microbiol., 2003, 69(7), 4278–4281 CrossRef CAS PubMed.
- V. Kumar, M. S. Basu and T. P. Rajendran, Crop Prot., 2008, 27, 891–905 CrossRef CAS.
- Y. Lu and W. Chen, 1D Pd-Based Nanomaterials as Efficient Electrocatalysts for Fuel Cells, 2014, pp. 321–357 Search PubMed.
- J. E. Millstone, S. J. Hurst, G. S. Métraux, J. I. Cutler and C. A. Mirkin, Small, 2009, 5, 646–664 CrossRef CAS PubMed.
- Y. Yin and A. P. Alivisatos, Nature, 2005, 437, 664–670 CrossRef CAS PubMed.
- T. K. Sau and A. L. Rogach, Adv. Mater., 2010, 22, 1781–1804 CrossRef CAS PubMed.
- N. Z. And and G. D. Stucky, J. Am. Chem. Soc., 2006, 128, 14278–14280 CrossRef PubMed.
- Z. Deng, M. Chen and L. Wu, J. Phys. Chem. C, 2007, 111, 11692–11698 CAS.
- Z. Li, L. Fan, T. Zhang and K. Li, J. Hazard. Mater., 2011, 187, 466–472 CrossRef CAS PubMed.
- L. Liu, J. Liu, Y. Wang, X. Yan and D. D. Sun, New J. Chem., 2011, 7, 1418–1423 RSC.
- A. L. Motlagh, S. Bastani and M. M. Hashemi, Prog. Org. Coat., 2014, 77, 502–511 CrossRef.
- D. Tang, R. Yuan and Y. Chai, J. Phys. Chem. B, 2006, 110, 11640–11646 CrossRef CAS PubMed.
- M. R. Hoffmann, W. Choi and D. W. Bahnemann, Chem. Rev., 1995, 95, 69–96 CrossRef CAS.
- D. A. P. D. Abreu, J. M. Cruz, I. Angulo and P. P. Losada, Packag. Technol. Sci., 2010, 23, 59–68 Search PubMed.
- D. L. Fang, W. J. Yang, B. M. Kimatu, A. M. Mariga, L. Y. Zhao, X. X. An and Q. H. Hu, Innovative Food Sci. Emerging Technol., 2015, 33, 489–497 Search PubMed.
- F. M. Yang, H. M. Li, F. Li, Z. H. Xin, L. Y. Zhao, Y. H. Zheng and Q. H. Hu, Food Sci., 2010, 75(3), C236–C240 CrossRef CAS PubMed.
- L. Li, C. J. Zhao, Y. D. Zhang, J. F. Yao, W. J. Yang, Q. H. Hu, C. L. Wang and C. J. Cao, Food Chem., 2017, 215, 477–482 CrossRef CAS PubMed.
- Z. Zheng, B. B. Huang, X. Y. Qin, X. Y. Zhang and Y. Dai, J. Mater. Chem., 2011, 21, 9079–9087 RSC.
- H. Zhang, X. Li and G. Chen, J. Mater. Chem., 2009, 19, 8223–8231 RSC.
- S. F. Chen, J. P. Li, K. Qian, W. P. Xu, Y. Lu, W. X. Huang and S. H. Yu, Nano Res., 2010, 3, 244–255 CrossRef CAS.
- H. Zhang and G. Chen, Environ. Sci. Technol., 2009, 43, 2905–2910 CrossRef CAS PubMed.
- R. Liu, P. Wang, X. Wang, H. Yu and J. Yu, J. Phys. Chem. C, 2012, 116, 17721–17728 CAS.
- S. K. Gogoi, P. Gopinath, A. Paul, A. Ramesh, S. S. Ghosh and A. Chattopadhyay, Langmuir, 2006, 22, 9322–9328 CrossRef CAS PubMed.
- X. H. Xu, W. J. Brownlow, S. V. Kyriacoou, Q. Wan and J. J. Viola, Biochemistry, 2004, 43, 10400–10413 CrossRef CAS PubMed.
- F. X. Zhang, N. J. Guan, Y. Z. Li, X. Zhang and J. X. Chen, Langmuir, 2003, 19, 8230–8234 CrossRef CAS.
- I. Bano, R. V. Kumar and A. Hameed, Ionics, 2012, 18, 307–313 CrossRef CAS.
- S. C. Chan and M. A. Barteau, Langmuir, 2005, 21, 5588–5595 CrossRef CAS PubMed.
- J. R. Regalbuto, A. Navada, S. Shadid, M. L. Bricker and Q. Chen, J. Catal., 1999, 184, 335–348 CrossRef CAS.
- C. Cao, G. Yang, L. Dubau, F. Maillard, S. D. Lambert, J. P. Pirard and N. Job, Appl. Catal., B, 2014, 150–151, 101–106 CrossRef CAS.
- L. D. Souza, L. Jiao, J. R. Regalbuto, J. T. Miller and A. J. Kropf, J. Catal., 2007, 248, 165–174 CrossRef.
- C. J. Cao, G. Yang, W. Song, X. R. Ju, Q. H. Hu and J. F. Yao, J. Power Sources, 2014, 272, 1030–1036 CrossRef CAS.
- J. P. Brunelle, Pure Appl. Chem., 1978, 50, 1211–1229 CrossRef CAS.
- J. Park and J. R. Regalbuto, J. Colloid Interface Sci., 1995, 175, 239–252 CrossRef CAS.
- A. Zielińska, E. Kowalska, J. W. Sobczak, L. Lacka, M. Gazda and B. Ohtani, Sep. Purif. Technol., 2010, 72, 309–318 CrossRef.
- K. Kaji, Fiber, 1975, 31, 204–214 CrossRef.
- R. Sanjines, J. Appl. Phys., 1994, 75, 2945–2951 CrossRef CAS.
- A. Klančnik, S. Piskernik, B. Jeršek and S. S. Možina, J. Microbiol. Methods, 2010, 81, 121–126 CrossRef PubMed.
- O. Yamamoto, M. Komatsu, J. Sawai and Z. Nakagawa, J. Mater. Sci.: Mater. Med., 2004, 15, 847–851 CrossRef CAS PubMed.
- G. Colon and B. C. W. J. Webster, J. Biomed. Mater. Res., Part A, 2006, 78, 595–604 CrossRef PubMed.
- M. Rai, A. Yadav and A. Gade, Biotechnol. Adv., 2010, 27, 76–83 CrossRef PubMed.
|
This journal is © The Royal Society of Chemistry 2017 |