DOI:
10.1039/C6RA28483C
(Paper)
RSC Adv., 2017,
7, 11297-11303
Homogeneous capture and heterogeneous separation of proteins by PEG-functionalized ionic liquid–water systems
Received
20th December 2016
, Accepted 9th February 2017
First published on 14th February 2017
Abstract
Extraction and separation of proteins is important for their interesting applications as continuous enzymatic reaction and/or product separation systems. In this work, a new highly efficient homogeneous capture and heterogeneous liquid–liquid separation strategy is presented for the extraction of proteins from aqueous solution by PEG (poly(ethylene glycol))-functionalized ionic liquids, which have a lower critical solution temperature (LCST) phase behaviour in water. The factors influencing the solvent extraction process such as homogeneous capture time, pH value of aqueous phase and water content in the ionic liquids were investigated systematically. It was found that homogeneous capture of the proteins was quite fast, typically 1 min was enough to achieve equilibrium, and the extraction efficiency was greatly affected by water phase pH value and water content in the ionic liquid phases. Under optimal conditions, the single-step extraction efficiency by [PEG800(mim)2][NTf2]2 was higher than 95% for most of the studied proteins (cytochrome c, myoglobin, hemoglobin, lysozyme and papain), but it was only 8% for bovine serum and 2% for peroxidase. Based on the significant differences in the extraction efficiencies observed, selective separation of the proteins from bovine serum or/and peroxidase was successfully performed using the IL/water mixture. In addition, circular dichroism and FT-IR spectroscopy were used to probe the structure and conformation change of the proteins, and the extraction mechanism was also discussed from the point of view of the electrostatic and hydrophobic/hydrophilic interactions of proteins with ionic liquids.
Introduction
Separation and purification of specific bio-macromolecules is one of the most critical procedures for life science studies. A range of techniques for protein extraction/separation are available in the literature.1,2 Yet it is frequently problematic when conventional organic solvents are used as an extraction medium due to their natural toxicity to biological molecules and life processes. Ionic liquids (ILs) are usually defined as organic salts with a melting point below 100 °C. A number of studies on bio-macromolecules/IL systems3–5 indicate that ILs display excellent performance for proteins such as enhancement of catalytic activity of enzymes6,7 and thermal stability of proteins.8,9 Thus, the applications of ILs in the extraction/separation of bio-macromolecules have recently attracted increasing interest.10,11
Some ILs having hydrophobic anions/cations can easily form liquid–liquid biphase systems after mixing with water, which makes them interesting alternatives for conventional organic solvents used in liquid–liquid extraction.12–15 However, very few reports can be found in the literature on the direct extraction of proteins from water using hydrophobic ILs16 due to the limited solubility and possible denaturation of proteins in most of neat hydrophobic ILs. Later, some ILs systems with the addition of an extractant have been developed to extract proteins or enzymes from water.17 In this procedure, sonication or much time shaken is usually applied in spite of fear of protein decomposition. Recently, extraordinary stability of proteins in ILs with a certain amount of water has been reported by a few groups,18–20 which cannot be realized in neat water. Thus, there are a growing number of publications on aqueous two phase systems (ATPSs) based on ILs for the primary recovery and purification of a variety of biological products.21–23 However, high concentrations of inorganic salts are always used in the IL-based ATPSs. This will produce a great amount of highly salty water in the application, thus causing environmental problems. Therefore, the development of highly efficient and environmentally friendly methods for the protein extraction is still a challenge.
In principle, if one strategy can be used to realize the homogeneous capture and heterogeneous liquid–liquid separation of proteins, the extraction efficient would be enhanced and the consumed energy could be decreased. Actually, some ILs have been found to possess upper critical solution temperature (UCST)-type phase transition or lower critical solution temperature (LCST)-type phase transition in H2O.24–26 It is expected that these aqueous ILs systems can be applied in homogeneous capture and heterogeneous separation of proteins. Indeed, Binnemans et al.27 found that betainium bis(trifluoromethylsulfonyl)imide–water mixture with an UCST phase behavior could be used for homogeneous capture and heterogeneous separation of metal ions. This provides an energy-saving extraction strategy due to the fast mass transfer speed and kinetic, and the shorter extraction equilibrium time. Other examples were also reported for the extraction of metal ions with such a strategy.28–30 However, to our knowledge, only one report can be found in the literature on the extraction of biopolymers such as proteins with this new strategy31 primarily due to the fact that proteins are often sensitivity to temperature, and high temperature can cause protein denaturation. On the other hand, in the thermo-responsive ILs/water mixture, the amount of water in the IL phase is strongly dependent on the temperature, which is also important for the stability and extraction efficiency of protein. Therefore, design of ILs with convenient phase separation temperature range in water is the key to achieve highly effective and safe extraction and separation of proteins from water.
Poly(ethylene glycol) (PEG) is important in many industrial, environmental, and biological applications because of its unique properties, such as non-toxicity, non-volatility, and biocompatibility.32 Incorporating PEG moiety into cationic (or anionic) unit of ILs can yield PEG-functionalized ILs. These ILs are a new appealing group of liquid materials and have found various applications in chemical synthesis,33 biomass dissolution,34 stabilization of proteins35 and nanoparticles.36 In a recent work,37 we have designed, synthesized and characterized a new class of PEG-functionalized imidazolium ILs [PEGm(mim)2][NTf2]2 (with PEG average molecular weight m = 200, 400, 600, 800 and 1000), and their chemical structures were shown in Chart 1. It was found that these ILs exhibited unique LCST phase behavior in water.38 The phase separation temperature was lower than 50 °C and could be tuned by the molecular weight of PEG middle block at any composition of the mixtures due to the fact that hydrophilicity of the ILs increases with increasing molecular weight of the PEG middle block. For example, when [PEG800(mim)2][NTf2]2 was mixed with an equal amount of water (50 wt%), the LCST-type phase separation temperature could be observed at 25 °C. This should be an ideal system for homogeneous capture (say at 22 °C) and heterogeneous separation (say at 27 °C) of proteins. In this work, we show the possibility to use these ILs for the homogeneous capture and heterogeneous separation of some proteins from water.
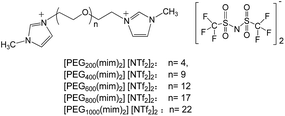 |
| Chart 1 Chemical structures of the ILs investigated in this work. | |
Experimental section
Materials
All the [PEGm(mim)2][NTf2]2 ILs were synthesized, purified, and characterized according to the procedures described previously.37 The purities of these ILs were found to be higher than 99.0 wt%, as determined by quantitative 1H NMR measurements.38 Myoglobin (Mb) from horse heart, hemoglobin (Hb) from bovine blood, albumin from bovine serum (BSA), trypsin from bovine pancreas, horseradish peroxidase (HRP) and lysozyme (Lyz) from chicken egg white, cytochrome c (Cyt.c) and papain were purchased from Sigma-Aldrich. All the proteins were used as received without further purification. Doubly distilled deionized water was used throughout the experiments. 1.0 M aqueous HCl or NaOH was used to adjust the pH of the systems.
Homogeneous capture and heterogeneous liquid–liquid separation of the proteins
In the extraction experiments, an IL such as [PEG800(mim)2][NTf2]2 was presaturated with water to minimize phase volume change when mixed with water. At the moment, the IL is classified as “hydrated IL”. Then, 1.0 ml of the presaturated IL was mixed with 1.0 ml of aqueous protein solution (1.0 mg ml−1) at room temperature. The resulting IL/water solution was changed into a single phase instantly under stirring and cooling in a water bath at 22.0 °C. After 1 min, this homogeneous solution was heated to 27.0 °C and left to stand until the separated water phase became clear. At this temperature, a 29.5 wt% of water content was determined gravimetrically39 for the IL phase. Once the volume of the top and bottom phases was recorded, concentrations of the protein in aqueous phases were measured by using a UV spectrophotometer (BeijingTU-1900), and the protein concentrations in IL phase was calculated by mass balance. The maximum absorption band at 408 nm was used to determine concentrations of the heme proteins Cyt.c, Mb, HRP and Hb, while the absorbance at 280 nm was used for the concentration determination of the other proteins. The relative error in the proteins concentration measurements was estimated to be within 5%. To avoid the interference from the phase components, the samples were diluted and analyzed against the blanks containing the same phase components but without protein. Partition coefficients (K) and extraction efficiencies (E%) of the proteins were calculated by using the equations: |
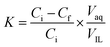 | (1) |
|
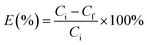 | (2) |
where Ci and Cf are the protein concentrations in the aqueous phase before and after extraction, Vaq and VIL are the volumes of the aqueous phase and IL phase, respectively.
Circular dichroism and FT-IR spectroscopic measurements
Circular dichroism (CD) spectra (300–500 nm) of Cyt.c in water and in the IL phase after extraction were determined at room temperature by using a Jasco J-820 CD spectropolarimeter, where [Cyt.c] = 1.0 mg ml−1 and the optical path length is 1 mm. IR spectra for Cyt.c in D2O and D2O-saturated IL phase were recorded on a PerkinElmer FT-IR spectrometer at room temperature with 16 scans at 4 cm−1 resolution for an acceptable signal-to-noise ratio.
Results and discussion
Extraction of Cyt.c from water to the IL phases
Here, Cyt.c was used as a representative protein to investigate the liquid–liquid extraction process since it is one of the most thoroughly characterized metalloproteins.40,41 The course to use [PEG800(mim)2][NTf2]2 for the homogeneous capture and heterogeneous liquid–liquid separation of Cyt.c was shown in Fig. 1. First, 1.0 ml of aqueous Cyt.c solution (1.0 mg ml−1) was added to 1.0 ml of the presaturated IL at room temperature. At this temperature, the aqueous phase was formed on the top of the IL phase (Fig. 1a), and Cyt.c was mainly present in the aqueous phase. Once the system was cooled to 22.0 °C, the two separated phases immediately became a homogeneous solution under stirring (Fig. 1b). After staying for 1 min, phase separation was then induced by gentle heating to 27 °C and then left it to stand until the colorless top phase was formed (Fig. 1c). In this way, the protein was exclusively exacted in the IL phase (bottom).
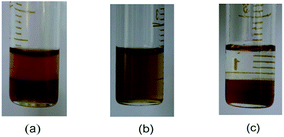 |
| Fig. 1 Homogeneous capture and heterogeneous liquid–liquid separation of Cyt.c (1.0 mg ml−1) by changing the temperature: (a), an aqueous solution of Cyt.c was added to [PEG800(mim)2][NTf2]2 at room temperature; (b), homogeneous capture of the protein for 1 min at 22 °C; (c), heterogeneous liquid–liquid separation of Cyt.c at 27 °C. The upper layer is aqueous phase and the lower layer is the IL phase. | |
To investigate the influence of homogeneous capture time on the extraction efficiency, each solution containing 1.0 ml of proteins and 1.0 ml of water-saturated [PEG800(mim)2][NTf2]2 was performed with the same procedure except for the different homogeneous capture time. Fig. 2 shows the extraction efficiency of Cyt.c as a function of homogeneous capture time. It can be seen that the extraction efficiency of the protein could reach to 98.1% in 5 seconds. After 1 minute, no significant changes in the extraction efficiency (99.8%) could be observed. Compared with the conventional liquid–liquid extraction systems, this very short equilibrium time is understandable since mass transfer and kinetics was significantly accelerated due to the molecular level mixing at the temperature below LCST. Therefore, 1 min was chosen to insure the attainment of extraction equilibrium.
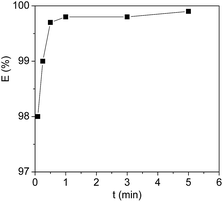 |
| Fig. 2 Extraction efficiency of Cyt.c by [PEG800(mim)2][NTf2]2 at 27 °C as a function of homogeneous capture time. The protein concentration = 1 mg ml−1, pH = 7.0. | |
Effect of water content in the ILs phases on proteins extraction
In the thermo-responsive ILs/water two phase mixtures investigated in this work, the amount of water in the ILs phases is strongly dependent on both the temperature and the molecular weight of PEG chains of the ILs. In order to realize the protein extraction at room temperature, water content in the IL phase can be controlled by tuning the molecular weight of PEG chains of the ILs. Because the phase separation temperature (45 °C) of [PEG1000(mim)2][NTf2]2 in equal weight of water was not convenient for the extraction, [PEG200(mim)2][NTf2]2, [PEG400(mim)2][NTf2], [PEG600(mim)2][NTf2]2 and [PEG800(mim)2][NTf2]2 were performed with the same procedure to study the effect of water content in the ILs phases on protein extraction. Fig. 3 shows the extraction efficiency of Cyt.c at 27.0 °C as a function of water content of the ILs phases. It can be seen from Fig. 3 that the extraction efficiency increased with increasing water content in the ILs phase. These results indicate that the extraction of proteins was closely related to water content in the ILs phases. Fujita et al.18 reported that mixtures of small amount of water and hydrophilic ILs with oxo acid residues were effective in dissolving proteins. They suggested that the small amount of water present in the ILs was probably involved in direct interaction with the proteins. In fact, the transfer of protein to the IL phase requires the breaking of the interaction between the cation and anion to create a cavity where the protein will be included, and the energy for this process would be obtained from the interactions between proteins and the IL. Because of the presence of water, the association between cation and anion of the IL is partially disrupted, leading to an increase both in the number of free ions and in the ionic mobility of the IL, which may enhance the interactions of the IL with protein. In addition, it has been reported that PEG is an effective chemical modifier for improving the solubility of Cyt.c in solvents including ILs without denaturation.42 These results clearly demonstrate that the partitioning of a protein into the PEG functionalized ILs is controllable on the basis of tuning molecular weight of PEG chains and water content in the ILs phases. A suitable amount of water is necessary to efficiently extract Cyt.c, and the hydrated state of ions may play an important role in controlling the affinity with proteins.
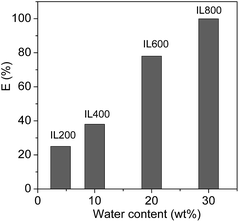 |
| Fig. 3 The extraction efficiency of Cyt.c at 27 °C as a function of water content in the ILs phases of [PEG200(mim)2][NTf2]2, [PEG400(mim)2][NTf2]2, [PEG600(mim)2][NTf2]2 and [PEG800(mim)2][NTf2]2. | |
Effect of pH on the extraction efficiency of the proteins
The extraction efficiency is expected to depend on the surface net charge of the proteins which can be adjusted by the pH values of the systems. To confirm the effect of the pH values, the extraction efficiency of Cyt.c was studied, as an example, as a function of pH, and the results were shown in Fig. 4. In this study, pH range of 2.3–12.5 was chosen to examine the pH dependence of proteins extraction because protein can easily be denatured at extreme pH values. It can be seen from Fig. 4 that the extraction efficiency of Cyt.c was kept constant at about 99.8% within the range of pH < 10, but sharply decreased when the pH value was over the isoelectric point (10.2) of the protein.
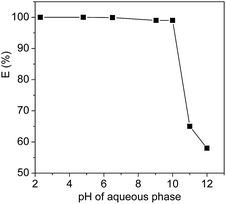 |
| Fig. 4 Extraction efficiencies of Cyt.c by [PEG800(mim)2][NTf2]2 as a function of the pH of the systems at 27 °C. | |
It is known that isoelectric point (pI) value of horse heart Cyt.c is 10.2, which indicates that Cyt.c exists as a cationic (–NH3+) species under neutral or acid conditions, while Cyt.c exists as a anion (–COO−) species when the pH values was over the isoelectric point value. This clearly indicates that surface charged groups in the protein is an important factor for the extraction efficiency of Cyt.c by the IL. The (CH2CH2O)n segments of PEG chains of the ILs are probably prefer to interact with the ammonium cation (–NH3+) instead of anion (–COO−) of Cyt.c by favourable hydrogen bonding and ion–dipole interactions, which allows the transfer of protein into the IL phase. In addition, considering the fact that the PEG-functionalized ILs used in this study include dications and exhibit a distinctive electrical double layer at the electrode surface,43,44 the stronger columbic interaction between the imidazolium cation and protein is another possible driving force for the extraction of Cyt.c.
Extraction efficiency of the proteins in the ILs–water systems
Based on the above discussion on the extraction of Cyt.c from aqueous solution, the partition behavior of the other proteins in [PEG800(mim)2][NTf2]2–water systems was also investigated at pH 7, and the results were given in Fig. 5, where the partition coefficients of these proteins varied from 0.05 to 53.0 (not shown). It is clearly indicated that by a single-step extraction procedure, 99.8% of Cyt.c, Mb and Hb, 99.0% of lysozyme, 95.0% of papain, and 88.9% of trypsin could be extracted into the IL phase. However, HRP and BSA were remained in the aqueous phase and the extraction efficiency was only 8% for BSA and 2% for HRP.
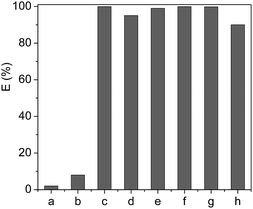 |
| Fig. 5 Extraction efficiency of the proteins (1 mg ml−1) by [PEG800(mim)2][NTf2]2 at pH 7.0 (a), HRP; (b), BSA; (c), Cyt.c; (d), papain; (e), Lyz; (f), Mb; (g), Hb and (h), typsin. | |
It is known that the proteins used in this study have different isoelectric points in water, which are 10.2 for Cyt.c, 7.0 for Hb and Mb, 7.2 for HRP, 8.7 for papain, 11.4 for Lyz, 9.4 for trypsin and 4.6 for BSA, respectively. Because the pH of IL–H2O system was fixed at 7.0, the isoelectric points of Hb, Mb and HRP are close to the pH value of IL–H2O system and the net charges of these proteins are null. On the other hand, the net charges of proteins including Cyt.c, Lyz, papain and trypsin are positive, but that of BSA is negative under the experimental conditions. At this stage, it is not difficult to understand why Cyt.c, Lyz, papain and trypsin could be easily extracted by the IL, and why BSA could not be extracted into the IL. However, although Hb, Mb and HRP have similar isoelectric point in value, the extraction efficiency of heme proteins of Hb and Mb was much higher than that of HRP. This result suggests that the extraction efficiency of the proteins cannot be explained only by their surface charge, there are other factors such as hydrophobicity/hydrophilicity of proteins, which are also important for affecting the extraction efficiency. For example, hydrophilic glycoside chains present in HRP tend to remain this protein in the aqueous phase in spite of the belief that PEG chain can more easily wrap-up or coordinate with heme-group of the protein,45 leading to its low extraction efficiency.
Selective separation of the target protein
As mentioned in Fig. 5, extraction efficiency of the proteins in the [PEG800(mim)2][NTf2]2–water system varies significantly. This suggests that HRP or BSA could be selectively separated from its mixture with Cyt.c, papain, Lyz, Mb, Hb and typsin. To confirm this deduction, 0.5 ml of aqueous Cyt.c (1.0 mg ml−1) and 0.5 ml of aqueous HRP (1.0 mg ml−1) were mixed to obtain a 1.0 ml of aqueous solution of the proteins. Then, 1.0 ml of the presaturated IL was added, and the mixture was cooled to 22.0 °C to generate a homogeneous solution. After capture of the protein, the solution was heated to 27.0 °C to induce phase separation. The content of proteins isolated in each phase was analyzed by 15% of standard SDS-PAGE (sodium dodecyl sulfate-polyacrylamide gel electrophoresis), and the gel was stained with Coomassie Brilliant Blue R-250. It is clearly observed from Fig. 6 that both phases exhibited a single band, but in different positions. The lane for the IL phase (Fig. 6a) showed a band at 12.5 kDa, which was assigned to Cyt.c, whereas the lane for the water phase (Fig. 6b) showed a band at 44 kDa, which corresponded to HRP. This shows that both Cyt.c and HRP were fully separated in the IL/water mixture.
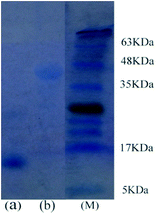 |
| Fig. 6 The standard SDS-PAGE analysis. (a), The IL phase; (b), the aqueous phase after separation of Cyt.c and HRP; (M), shows molecular mass markers (5–245 kDa).The band at 12.5 kDa was assigned to Cyt.c and the band at 44 kDa to HRP. | |
Possible structure and conformation change of the proteins after extraction
Fig. 7 shows the soret region CD spectra (350–450 nm) of native Cyt.c in water and in [PEG800(mim)2][NTf2]2 phase. It can be seen that a negative peak at 417 nm and a positive peak at 404 nm appeared in water due to a split cotton effect for native Cyt.c in H2O. The negative band was resulted from the interaction of Phe80 (phenylalanyl-80) with heme on the Met80 (methionyl-80) side of the heme plane, and axial coordination of the low spin heme iron with His18 (histidyl-18) and Met80 strongly stabilized the native tertiary structure.46 In the [PEG800(mim)2][NTf2]2 phase, the CD spectrum was characterized by the disappearance of the negative cotton effect and a concomitant increase in the intensity of the positive cotton effect at 400 nm. These changes indicates that the cleavage of the sixth coordinating bond occurred between iron and the Met80, which probably resulted mainly from favorable interactions between Cyt.c and (CH2CH2O)n segments of the PEG chain in the IL. The fact that ILs with shorter PEG chain such as [PEG200(mim)2][NTf2]2, [PEG400(mim)2][NTf2]2 and [PEG600(mim)2][NTf2]2 had lower extraction efficiency for Cyt.c supports this speculation.
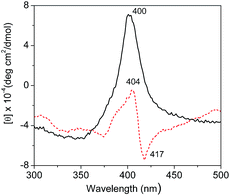 |
| Fig. 7 CD spectra (300–500 nm) of Cyt.c (1.0 mg ml−1) in water (red, broken line) and in [PEG800(mim)2][NTf2]2 phase after extraction (black, solid line). | |
Similar phenomenon was also observed for Cyt.c from an aqueous phase into [C2OHmim][NTf2] with crow ether complexation.47 These results suggest a change in heme–polypeptide interactions in the vicinity of the heme active site and an increase in the planarity of the ferric heme moiety, which can trigger the functional conversion of Cyt.c from an electron-transfer protein to peroxidase.46,48 Unfortunately, we could not take an accurate measurement of the CD spectrum in the α-helix region (190–230 nm) of Cyt.c to examine the secondary structure of this protein, because of the interference of the IL in the range of short-wavelength light. Therefore, the findings from CD investigations only confirm that the change in tertiary structure of Cyt.c was mainly resulted from its interaction with PEG chain of the IL.
FT-IR spectroscopy is one of the classical experimental methods to provide information on secondary structure features of proteins.49,50 It is known that amide is a basic unit of the peptide bond: amide I is characterized by both C
O stretching and ring stretching vibrations, while amide II is characterized only by C–N stretching vibration. The absorption bands most widely used as structure probes in protein FT-IR spectroscopy is the amide I vibrations, which fall in the range of 1690–1600 cm−1.49 FT-IR spectra of Cyt.c in [PEG800(mim)2][NTf2]2 phase after extraction were determined and compared with those of the protein in water to examine the influence of the IL on the secondary structure of Cyt.c. Fig. 8 shows FT-IR spectra of neat IL in D2O, Cyt.c in D2O, and Cyt.c in IL phase after extraction (IL–D2O). Here, D2O was used instead of H2O as the solvent to eliminate the overlap of the O–H stretching of H2O with amide I stretching of Cyt.c in the range of 1690–1600 cm−1. As can be seen from Fig. 8, amide I bond of the protein in the IL phase was identified to be the same with that in D2O, suggesting no observable change of the secondary structure of Cyt.c in the IL phase. This is very important for keeping protein activity during the extraction. However, amide II bond of the protein could not be identified clearly because of the overlapped C–N stretching of cation of the IL. Therefore, we can conclude that [PEG800(mim)2][NTf2]2 provides a gentle environment for biomacromolecules.
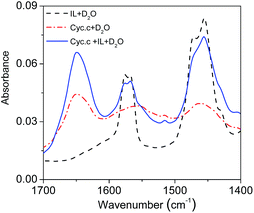 |
| Fig. 8 FT-IR spectra of the [PEG800(mim)2][NTf2]2 phase (black, solid line) as well as those of Cyt.c in D2O (red, dash dot line) and in the IL phase after extraction (blue, dash line). | |
Conclusions
In this work, a homogeneous capture and heterogeneous liquid–liquid separation strategy is reported for the extraction of proteins by PEG-functionalized ILs [PEGm(mim)2][NTf2]2–water systems. These ILs exhibited LCST phase behaviour in water, and the phase separation temperature and water content of the systems can be tuned for the efficient extraction of proteins by changing molecular weight of PEG chains of the ILs. It was found that the extraction efficiency of proteins was strongly dependent on the surface charges of the proteins and water content of the ILs phases. Under optimal conditions, by a single-step extraction procedure, 99.8% of Cyt.c, Mb and Hb, 99.0% of lysozyme, 95.0% of papain, and 88.9% of trypsin could be extracted into the IL phase. However, HRP and BSA were remained in the aqueous phase and the extraction efficiency was only 8% for BSA and 2% for HRP. From the considerable difference in the extraction efficiency, selective extraction of proteins from HRP and/or BSA was realized by using the IL/water mixture. CD and FT-IR spectra investigation suggest that only tertiary structure of Cyt.c in the IL phase changes after extraction, resulted from the interaction of the protein with PEG chain of the IL. But no native secondary structure change was observed for the protein. Therefore, the ILs–water systems provide a gentle environment for proteins.
Compared with the ILs based liquid–liquid extraction systems for proteins, the ILs–water systems developed in this work have many advantages such as very fast mass transfer and kinetics because of the molecular level mixing during the homogeneous capture of protein, tuneable phase separation temperature and water content of the ILs phases due to the functionalization of the ILs with different molecular weights of PEG chains. In addition, the preparation of the ILs is more simple and inexpensive. Therefore, these PEG-functionalized ILs mixed with water provide highly efficient, fast and environmentally friendly systems for the recovery and selective separation of proteins.
Acknowledgements
This work was supported by the National Natural Science Foundation of China (No. 21573060 and 21673068), the Program for Innovative Research Team in Science and Technology in University of Henan Province (No. 16IRTSTHN002), and the Plan for Scientific Innovation Talent of Henan Province (No. 144200510004).
Notes and references
- T. Ono, M. Goto, F. Nakashio and T. A. Hatton, Biotechnol. Prog., 1996, 12, 793–800 CrossRef CAS PubMed.
- M. G. Roper, M. L. Frisk, J. P. Oberlander, J. P. Ferrance, B. J. McGrory and J. P. Landers, Anal. Chim. Acta, 2006, 569, 195–202 CrossRef CAS.
- R. A. Sheldon, R. M. Lau, M. J. Sorgedrager, F. van Rantwijk and K. R. Seddon, Green Chem., 2002, 4, 147–151 RSC.
- J. L. Kaar, A. M. Jesionowski, J. A. Berberich, R. Moulton and A. J. Russell, J. Am. Chem. Soc., 2003, 125, 4125–4131 CrossRef CAS PubMed.
- F. van Rantwijk and R. A. Sheldon, Chem. Rev., 2007, 107, 2757–2785 CrossRef CAS PubMed.
- R. Mareria Lau, F. van Rantwijik, K. R. Seddon and R. A. Sheldon, Org. Lett., 2000, 2, 4189–4191 CrossRef.
- U. Kragl, M. Eckstein and N. Kaftzik, Curr. Opin. Biotechnol., 2002, 13, 565–571 CrossRef CAS PubMed.
- S. N. Baker, T. M. McCleskey, S. Pandey and G. A. Baker, Chem. Commun., 2004, 40, 940–941 RSC.
- P. Attri, P. Venkatesu and A. Kumar, Phys. Chem. Chem. Phys., 2011, 13, 2788–2796 RSC.
- R. K. Desai, M. Streefland, R. H. Wijffels and M. H. M. Eppink, Green Chem., 2014, 16, 2670–2679 RSC.
- S. Shahriari, C. M. Neves, M. G. Freire and J. A. Coutinho, J. Phys. Chem. B, 2012, 116, 7252–7258 CrossRef CAS PubMed.
- Z. Li, X. Liu, Y. Pei, J. Wang and M. He, Green Chem., 2012, 14, 2941–2950 RSC.
- M. L. Dietz, Sep. Sci. Technol., 2006, 41, 2047–2063 CrossRef CAS.
- Y. Liu, J. Chen and D. Li, Sep. Sci. Technol., 2012, 47, 223–232 CrossRef CAS.
- H. Zhao, S. Xia and P. Ma, J. Chem. Technol. Biotechnol., 2005, 80, 1089–1096 CrossRef CAS.
- D. Cheng, X. Chen, Y. Shu and J. Wang, Talanta, 2008, 75, 1270–1278 CrossRef CAS PubMed.
- K. Shimojo, K. Nakashima, N. Kamiya and M. Goto, Biomacromolecules, 2006, 7, 2–5 CrossRef CAS PubMed.
- K. Fujita, M. Forsyth, D. R. MacFarlane, R. W. Reid and G. D. EIliott, Biotechnol. Bioeng., 2006, 94, 1209–1213 CrossRef CAS PubMed.
- N. Byrne, L. M. Wang, J. P. Belieres and C. A. Angell, Chem. Commun., 2007, 43, 2714–2716 RSC.
- K. Fujita, N. Nakamura, K. Igarashi, M. Samejima and H. Ohno, Green Chem., 2009, 11, 351–354 RSC.
- X. Lin, Y. Wang, Q. Zeng, X. Ding and J. Chen, Analyst, 2013, 138, 6445–6453 RSC.
- M. G. Freire, A. F. M. Cláudio, J. M. M. Araújo, J. A. P. Coutinho, I. M. Marrucho, J. N. C. Lopes and L. P. N. Rebelo, Chem. Soc. Rev., 2012, 41, 4966–4995 RSC.
- Y. Pei, J. Wang, K. Wu, X. Xuan and X. Lu, Sep. Purif. Technol., 2009, 64, 288–295 CrossRef CAS.
- H. Yoshimitsu, A. Kanazawa, S. Kanaoka and S. Aoshima, Macromolecules, 2012, 45, 9427–9434 CrossRef CAS.
- Y. Men, H. Schlaad and J. Yuan, ACS Macro Lett., 2013, 2, 456–459 CrossRef CAS.
- K. Fukumoto and H. Ohno, Angew. Chem., Int. Ed., 2007, 46, 1852–1855 CrossRef CAS PubMed.
- P. Nockemann, B. Thijs, S. Pittois, J. Thoen, C. Glorieux, K. Van Hecke, L. Van Meervelt, B. Kirchner and K. Binnemans, J. Phys. Chem. B, 2006, 110, 20978–20992 CrossRef CAS PubMed.
- D. Depuydt, L. Liu, C. Glorieux, W. Dehaena and K. Binnemans, Chem. Commun., 2015, 51, 14183–14186 RSC.
- T. V. Hoogerstraete, B. Onghena and K. Binnemans, J. Phys. Chem. Lett., 2013, 4, 1659–1663 CrossRef CAS PubMed.
- M. Blesic, H. Q. N. Gunaratne, J. Jacquemin, P. Nockemann, S. Olejarz, K. R. Seddon and C. R. Strauss, Green Chem., 2014, 16, 4115–4121 RSC.
- Y. Kohno, S. Saita, K. Murata, N. Nakamura and H. Ohno, Polym. Chem., 2011, 2, 862–867 RSC.
- J. Chen, S. K. Spear, J. G. Huddleston and R. D. Rogers, Green Chem., 2005, 7, 64–82 RSC.
- M. M. Cecchini, C. Charnay, F. D. Angelis, F. Lamaty, J. Martinez and E. Colacino, ChemSusChem, 2014, 7, 45–65 CrossRef CAS PubMed.
- S. Tang, G. A. Baker, S. Ravula, J. E. Jonesc and H. Zhao, Green Chem., 2012, 14, 2922–2932 RSC.
- S. Wallert, K. Drauz, I. Grayson, H. Grçger, P. Dominguez de Maria and C. Bolm, Green Chem., 2005, 7, 602–605 RSC.
- H. S. Schrekker, M. A. Gelesky, M. P. Stracke, C. M. L. Schrekker, G. Machado, S. R. Teixeira, J. C. Rubim and J. Dupont, J. Colloid Interface Sci., 2007, 316, 189–195 CrossRef CAS PubMed.
- W. Yao, H. Wang, G. Cui, Z. Li, A. Zhu, S. Zhang and J. Wang, Angew. Chem., Int. Ed., 2016, 55, 7934–7938 CrossRef CAS PubMed.
- W. Yao, H. Wang, G. Cui, Z. Li and J. Wang, Phys. Chem. Chem. Phys., 2016, 18, 29192–29198 RSC.
- M. G. Freire, P. J. Carvalho, R. L. Gardas, I. M. Marrucho, L. M. Santos and J. A. Coutinho, J. Phys. Chem. B, 2008, 112, 1604–1610 CrossRef CAS PubMed.
- R. A. Scott and A. G. Mauk, Cytochrome c: a multidisciplinary approach. University Science Books, 1996 Search PubMed.
- J. R. Telford, P. W. Stafshede, H. B. Gray and J. R. Winkler, Acc. Chem. Res., 1998, 31, 755–763 CrossRef CAS.
- N. V. Kawahara and H. Ohno, Solid State Ionics, 1998, 113, 161–166 CrossRef.
- S. Li, J. L. Bañuelos, P. Zhang, G. Feng, S. Dai, G. Rotherc and P. T. Cummings, Soft Matter, 2014, 10, 9193–9200 RSC.
- T. Ishida and H. Shirota, J. Phys. Chem. B, 2013, 117, 1136–1150 CrossRef CAS PubMed.
- J. D. Holbrey, A. E. Visser, S. K. Spear, W. M. Reichert, R. P. Swatloski, G. A. Broker and R. D. Rogers, Green Chem., 2003, 5, 129–135 RSC.
- M. Bihari, T. P. Russell and D. A. Hoagland, Biomacromolecules, 2010, 11, 2944–2948 CrossRef CAS PubMed.
- K. Shimojo, N. Kamiya, F. Tani, H. Naganawa, Y. Naruta and M. Goto, Anal. Chem., 2006, 78, 7735–7742 CrossRef CAS PubMed.
- J. W. Brauner, C. R. Flach and R. Mendelsohn, J. Am. Chem. Soc., 2005, 127, 100–109 CrossRef CAS PubMed.
- S. Strassburg, H. Bermudez and D. Hoagland, Biomacromolecules, 2016, 17, 2233–2239 CrossRef CAS PubMed.
- K. Fujita, D. R. MacFarlane and M. Forsyth, Chem. Commun., 2005, 41, 4804–4806 RSC.
|
This journal is © The Royal Society of Chemistry 2017 |
Click here to see how this site uses Cookies. View our privacy policy here.