DOI:
10.1039/C6RA28346B
(Paper)
RSC Adv., 2017,
7, 7658-7670
Novel Bi2O2CO3/polypyrrole/g-C3N4 nanocomposites with efficient photocatalytic and nonlinear optical properties†
Received
18th December 2016
, Accepted 11th January 2017
First published on 23rd January 2017
Abstract
Semiconductor nanostructures perform wide applications in light-driven physical and chemical processes; in particular, nanomaterials based on Bi2O2CO3 with tunable photophysical properties have attracted intense interest. However, the nonlinear optical properties and photocatalytic performances of the ternary system Bi2O2CO3/polypyrrole/g-C3N4 have not been reported. In this paper, a series of novel Bi2O2CO3/polypyrrole/g-C3N4 nanocomposites loaded with different g-C3N4 contents was prepared to maximize the photocatalytic activity and optical nonlinearity. The structure, composition and morphology of the as-prepared samples were characterized by Fourier-transform infrared spectroscopy, X-ray powder diffraction, transmission electron microscopy, X-ray photoelectron spectroscopy and Raman spectroscopy. The wide absorption of the samples in the visible light region makes them suitable for photocatalytic and nonlinear transmission studies. Their photocatalytic performances were evaluated by degradation of rhodamine B. The associated photocatalytic activity of Bi2O2CO3/polypyrrole/g-C3N4 nanocomposites is shown to be dependent on the g-C3N4 loading. Compared to Bi2O2CO3, PPy, g-C3N4, BP and B/C0.5, the BP/C0.5 composite with 0.5 wt% g-C3N4 exhibits the highest photocatalytic activity. The nonlinear optical properties were investigated by open-aperture Z-scan measurements at 532 nm with 4 ns laser pulses. The results demonstrated that the BP/C0.5 composite exhibited improved properties for photocatalysis and optical nonlinearity, which is ascribed to a combination of mechanisms.
1. Introduction
Nanomaterials exhibit changes in their physical and chemical properties with respect to their shape, size and composition. Nowadays, nanoelectronics is the most prevalent form of nanotechnology. Improvements in nanodevice performance are anticipated, which accounts for the extensive worldwide research efforts in the development of nanoscale material hybrids. Given their low price and abundant sources on Earth, bismuth-containing semiconductors have been attracting extensive attention over the past two decades.1–5 Sillén-type Bi2O2CO3 with alternating Bi2O22+ and CO32− layers bears close resemblance to the Aurivillius-type oxides, which are important semiconductors that have long been used for medical and healthcare purposes.6 Nevertheless, BiO2CO3 with the forbidden band width of ca. 3.3 eV can only absorb UV light, resulting in the low utilization efficiency of solar radiation.1 According to the solar spectrum, UV light accounts for about 3–6% of the solar spectrum, whereas visible light comprises as much as 43%. Therefore, many scientists have paid much attention to how to broaden the photoabsorption region and improve the photocatalytic performance.7–12 The conjugated polymers bearing π conjugated electron systems, such as polypyrrole (PPy) and their derivatives, have been shown great promises because of their high absorption coefficients in the visible range of the spectrum and highly efficient electron transport properties.13,14 The conjugated structure and perfect conductivity of PPy can be useful to electron transfer during photocatalytic processes. Therefore, they can be used as a stable photosensitizer to modify semiconductors for improving photocatalytic efficiency. Graphitic carbon nitride (g-C3N4) is a novel metal-free photocatalyst with a band gap of ca. 2.8 eV, which is active for the degradation of some organic dyes such as rhodamine B under visible light irradiation,15 and has been coupled with many semiconductor materials to hinder electron–hole pair recombination and enhance photocatalytic efficiency.16,17
Meanwhile, the rapid development of nanoscience and nanotechnology provides lots of new opportunities for nonlinear optics. A growing number of nanomaterials have been shown to possess remarkable nonlinear optical properties, which promotes the design and fabrication of nanoscale optoelectronic and photonic devices in the field of optical limiting and optical switching.18–20 Optical limiter is the optical component which reduces the laser beam intensity for the protection of eyes and light sensors. Nonlinear optical properties of nanoscale materials can be adjusted by changing their composition, and likewise, control of their optical limiting performance is desirable for high end applications.21 Optical nonlinearity (including optical limiting) in individual nanoscale materials, i.e. carbon nitride nanotubes,22 Bi2O2CO3 (ref. 23) and PPy,24 and coupled systems (hybrids) such as g-C3N4 functionalized with nine-atom silver quantum clusters,25 zinc oxide doped bismuth26 and transition metal oxide nanoparticles grafted graphitic g-C3N4 (ref. 27) have been reported. Similarly, the role of semiconductors functionalized g-C3N4 for photocatalytic applications is distinctive due to the fact that they are simulated sunlight active.28 In view of the unique photoelectric properties of BiO2CO3, PPy and g-C3N4, it is of significant interest to design and prepare interesting functional materials combining the distinct optoelectronic properties of the individual components in suitable form. Particularly, the photocatalytic and nonlinear optical performances in coupled systems are expected to be better than those in independent systems due to the combination of the mechanisms (photocatalytic/nonlinear) occurring in the individual systems. To the best of our knowledge, there are no reports yet on the nonlinear optical properties and photocatalytic performances of the ternary system Bi2O2CO3/polypyrrole/g-C3N4.
Based on the above considerations, herein, we report the preparation of a series of novel Bi2O2CO3/PPy/g-C3N4 nanocomposites loaded with different g-C3N4 contents by a facile refluxing process. The composition and structure of the samples were investigated by means of Fourier transform infrared spectroscopy (FTIR), X-ray diffraction (XRD), transmission electron microscopy (TEM), XPS and Raman spectroscopy. The photocatalytic activity of the as-prepared composite materials was evaluated towards degradation of RhB under simulated sunlight and a possible mechanism of photocatalytic degradation is presented. In particular, the effect of the g-C3N4 content on RhB photodegradation was investigated in detail. We further explored the nonlinear optical properties of the samples at the visible spectral wavelength of 532 nm, using 4 ns laser pulses. The introduction of PPy and g-C3N4 yields a moderate enhancement of the nonlinearity when compared to the pristine Bi2O2CO3. Donor–acceptor complexes can be formed when the composites are photoexcited, and nonlinearity enhancement occurs due to a combination of mechanisms. The results demonstrate that the combination of the three materials (Bi2O2CO3, PPy and g-C3N4) in such a way can be a promising approach to improve the photoelectric performances, and the samples are capable of exhibiting dual functionality, both as efficient photocatalysts and optical limiters.
2. Experimental section
2.1. Materials and reagents
Pyrrole monomer (C4H4NH, analytical grade) was provided by Shanghai Sinopharm Chemical Reagent Co. Ltd, China, which was distilled under reduced pressure until it became colorless and stored under nitrogen in dark before use. Tetrahydrofuran (THF) were dried over metallic sodium and distilled before use. All other chemicals were of chemical and analytical grade used as received unless otherwise stated. Deionized water was used throughout the materials preparation process. g-C3N4 was prepared according to the literature as reported.29
2.2. Characterization
The as-prepared Bi2O2CO3/PPy/g-C3N4 nanocomposites were characterized by using different techniques. Fourier-transform infrared (FTIR) spectra of the samples were collected on a MB154S-FTIR spectrometer (Bomem, Canada) at room temperature using spectroscopic grade KBr pellets in the range 4000–400 cm−1. X-ray powder diffraction (XRD) analysis was performed at room temperature by means of a XD-3 diffractometer (Beijing Purkinje General Instrument Co. Ltd, China) using Cu Kα radiation (λ = 0.15418 nm) in the scanning range from 10° to 80° at a rate of 3° min−1. Transmission electron microscopy (TEM) experiments were carried out by using a JEM-2100 (JEOL) instrument working at 200 kV. The samples were thoroughly dispersed in ethanol. Then a drop of a dilute dispersion of the as-prepared products was coated on amorphous carbon-coated copper grids and then dried in air before it was transferred into the TEM sample chamber. Raman spectra were measured on a Renishaw Invia Raman Microscope with Ar+ radiation (532 nm); the laser light was focused onto samples by using a microscope equipped with a 100× objective. Energy dispersive X-ray spectroscopy (EDS) was also done for further chemical analysis using a JSM7001F (JEOL) equipped with an Oxford Instruments INCA system. X-ray photoelectron spectroscopy (XPS) analyses were performed on a RBD upgraded PHI-5000C ESCA (Perkin-Elmer) electron spectrometer with a Mg Kα line at 280 eV. Surface areas of the as-prepared samples were measured using Brunauer–Emmett–Teller (BET) method performed on a NOVA 2000e instrument. Pore size distributions were determined by the Barrett–Joyner–Halenda (BJH) method using adsorption data. Prior to the measurements, the samples were degassed at 150 °C for 3 h under vacuum. UV-Vis diffuse spectra were recorded using a Varian Cary 500 spectrophotometer at room temperature in the range 200–800 nm by using BaSO4 pellet as a reflectance standard. The fluorescence spectra were recorded on a Fluoro-Max-P spectrofluorimeter.
2.3. Measurement of photocatalytic activity
The photocatalytic activities of the samples were evaluated by the photocatalytic degradation of rhodamine (RhB) in aqueous solution under simulated light irradiation at room temperature. A 350 W Xe-lamp (Nanshen Company, Shanghai) was used as simulated sunlight source, which is located ca. 20 cm above the sample dish. In a typical procedure, some catalyst (ca. 50 mg) was suspended in 50 mL RhB aqueous solution (0.5 mM, pH value: 7) in a Pyrex reactor surrounded by circulating water to cool the lamp. Prior to irradiation, the suspension was stirred in the dark for about 15 min to reach the adsorption balance between the organic molecules and the catalyst surface. At given irradiation time intervals, about 3.0 mL of the reaction suspension was collected, and immediately separated by centrifugation; the RhB concentration after illumination was determined at ca. 554 nm by using a UV-vis spectrophotometer.
2.4. Electrochemical measurements
The photoelectrochemical measurements of the as-prepared samples were carried out in an aqueous solution containing 0.2 M Na2SO4 with an electrochemical workstation (CHI 614D, CH Instrument). Working electrodes were prepared as follows: the as-prepared samples (20 mg) and Nafion solution (5 wt%, 40 μL) were dispersed in 0.5 mL of water/ethanol (4/1, v/v) by ultrasonication (ultrasonic probe, 2 mm diameter, 130 W, 2 h) to form homogeneous ink. Then 40 μL of the catalyst ink was dropped onto the indium tin oxide (ITO) substrate. Next, these electrodes were dried in an oven at 60 °C for 30 min. A three-electrode configuration was employed in the measurements, with the as-prepared samples covered ITO as the working electrode, a Pt wire (1 × 1 cm2) as the counter electrode, and a silver/silver chloride (Ag/AgCl) electrode as the reference electrode. The ITO substrate covered with samples was assembled into a homemade Teflon electrochemical cell, with a defined area (0.7854 cm2) of the front surface of sample exposing to electrolyte during measurements. For photoelectrochemical measurements, a xenon lamp (350 W) was utilized as the light source, and the incident density of illumination was adjusted to 100 mW cm−2 before each experiment. The transient photocurrent measurement was performed at a constant potential of 0.95 V vs. Ag/AgCl. Electrochemical impedance spectroscopy was measured at an open-circuit voltage. A sinusoidal ac perturbation of 10 mV was used to the electrode over the frequency of 1 to 106 Hz.
2.5. Nonlinear optical measurements
The nonlinear optical property of the samples was measured by the Z-scan technique, which is widely used in optical nonlinearity, mainly due to the simplicity of the experimental setup and the easy interpretation of the results. The major advantage of this technique is that the magnitude and the sign of the nonlinearity can be provided simultaneously. The samples were dispersed in dimethylsulfoxide (DMSO) in a quartz cell of 2 mm thickness for the nonlinear optical measurements, which were performed with linearly polarized 4 ns pulsed laser radiation at 532 nm, generated from a mode-locked Nd:YAG laser with a repetition rate of 2 Hz. The optical pulses were of top-hat spatial profiles. DMSO solutions of the tested samples were positioned at the focal point (z = 0) of a lens with a focal length of 40 cm and the cells were then moved along the axis of the incident beam (z-direction) with respect to the focal point. Since the sample experiences different incident laser energy at each position as it moving along the z direction, any nonlinearity in transmission can be revealed by this measurement. The energy of the input (laser energy reaching the sample) and output (laser energy exiting the sample) laser pulses was monitored simultaneously by two energy detectors (Rjp-765 energy probe), which were linked to a computer by a GPIB interface. To measure the nonlinear absorption of the samples, the Z-dependent transmittance was measured by using the open-aperture Z-scan method. In this technique, the aperture is absent, and so the total transmitted energy is detected, from which the nature of the absorptive optical nonlinearity can be determined alone. Division of the Z-scan curve obtained with an aperture by that without an aperture gives a curve with nonlinear absorption effectively eliminated, and affords the nonlinear refraction response.
2.6. Preparation of nanocomposites
2.6.1. Preparation of Bi2O2CO3/PPy nanocomposites. PPy was prepared according to the reported method.8 Bi2O2CO3 nanosheets were obtained by using a hydrothermal method modified from a previous work.30 Briefly, bismuth nitrate pentahydrate (0.96 g), trisodium citrate dihydrate (1.76 g) and urea (0.48 g) was mixed with 30 mL deionized H2O and sonicated for 30 min at room temperature to obtain a homogeneous suspension. The resulting mixture was transferred into a 50 mL Teflon-lined stainless autoclave and heated at 200 °C for 24 h. Upon cooling to room temperature, the as-obtained precipitates were filtered, washed thoroughly with deionized H2O several times, rinsed with EtOH, and then dried under vacuum at room temperature for 12 h to produce a white powder of Bi2O2CO3. The Bi2O2CO3/PPy (BP) nanocomposites were prepared as follows: the obtained Bi2O2CO3 (200 mg) and PPy (2 mg) were added into THF (20 mL), subjected to ultrasound for 2 h, and then stirred for 24 h under reflux. Finally, the suspension was centrifuged and washed by deionized H2O and EtOH, and then dried under vacuum at room temperature for 12 h. For the purpose of comparison, the Bi2O2CO3/g-C3N4 (B/C0.5) composites with 0.5 wt% g-C3N4 were also prepared by the same method.
2.6.2. Preparation of Bi2O2CO3/PPy/C3N4 nanocomposites. In a typical procedure, the as-prepared BP composites (80 mg) were first sonicated in THF (20 mL) for 1 h, and then a certain amount of g-C3N4 was added to the above solution and stirred for another 1 h to obtain a homogenous suspension. Subsequently, the mixture solution was treated by refluxing for 12 h. After be cooled down to room temperature naturally, the resultant materials were collected, washed with EtOH and deionized water for several times. At last, the obtained samples were dried under vacuum at room temperature for 12 h. To investigate the effect of g-C3N4 on the photocatalytic degradation of the RhB solution, the weight percentage of g-C3N4 to the BP composites was varied from 0.1 to 2.0 (e.g. 0.1, 0.25, 0.5, 1.0, 1.5, 2.0 wt%), and the corresponding samples were labeled as BP/C0.1, BP/C0.25, BP/C0.5, BP/C1.0, BP/C1.5, and BP/C2.0, respectively.
3. Results and discussion
3.1. Structure, composition and morphology
FTIR spectroscopy was carried out to confirm the composition of samples. Fig. 1 displays the FTIR spectra of all the prepared samples. In the case of the pristine Bi2O2CO3, two intensive peaks at 1389 and 1469 cm−1 are ascribed to the antisymmetric vibrational mode ν3 of the CO32− group, while the peaks at 845 cm−1 is assigned to the out-of-plane bending mode ν2 of the CO32− group.31 Moreover, the absorption band at 549 cm−1 is attributed to the stretching mode of the Bi–O–Bi group.32 For the Bi2O2CO3/PPy/C3N4 composites, the characteristic bands corresponding to Bi2O2CO3 still remain, but the typical absorption peaks of PPy and g-C3N4 decrease dramatically in intensity or even disappear when compared to those of the pure PPy (for comparative purpose, the FTIR spectrum of PPy is also provided (Fig. S1 in ESI†)) and g-C3N4, which may be due to their low content in the composites. It is obvious that no impurities were detected, indicating the high purity of the Bi2O2CO3/PPy/C3N4 composites. Similar results are also observed for BP and B/C0.5. Raman spectroscopy is an efficient way to detect the purity and the structure of the composite with high sensitivity. Fig. 2 shows the Raman spectra of the Bi2O2CO3, BP, B/C0.5 and BP/C0.5 samples. For pure Bi2O2CO3, the strong bands below 600 cm−1 are mainly due to lattice vibration.30 The intense Raman band at 165 cm−1 is attributed to the external vibration mode of the carbonate ion, and the band at 355 cm−1 probably results from the motion of oxygen atoms in the polymeric (BiO)nn+ cation.33 The small band at 665 cm−1 in the Raman spectrum is indexed to the internal vibration ν4 of the CO32− group. The band at 1072 cm−1 is assigned to the internal vibration ν1 of the CO32− group. Two bands at 1374 and 1469 cm−1 are indexed to the internal vibration ν3 of the CO32− group.34 When compared to Bi2O2CO3, a down-shift (5 cm−1) corresponding to the external vibration mode of the carbonate ion was observed for BP. While the bands in the region 1200–1800 cm−1 show notable broadening relative to Bi2O2CO3, as a consequence of the introduction of PPy (for comparative purpose, the Raman spectrum of PPy is also provided (Fig. S2 in ESI†)). In the case of the BP/C0.5 composite, a similar down-shift can also be observed in comparison to BP. Furthermore, a new peak was observed for BP/C0.5 in the region 1200–1800 cm−1 when compared to BP and Bi2O2CO3, resulting from the doping of C3N4 (for comparative purpose, the Raman spectrum of g-C3N4 is also provided (Fig. S3 in ESI†)). Indeed, a broad band is also found for B/C0.5 due to the introduction of g-C3N4. All above results demonstrated the successful combination of the Bi2O2CO3 and PPy together with g-C3N4 in a single system.
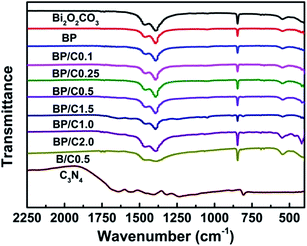 |
| Fig. 1 FTIR spectra of the as-prepared samples. | |
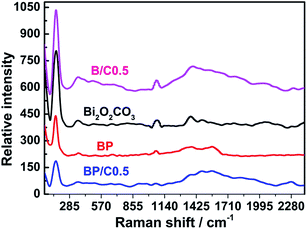 |
| Fig. 2 Raman spectra of Bi2O2CO3, BP, B/C0.5 and BP/C0.5. | |
The crystal quality of the as-prepared samples was analyzed by XRD, the results being shown in Fig. 3. All the diffraction peaks can be readily indexed to the standard diffraction data of tetragonal Bi2O2CO3 (JCPDS no. 41-1488). For Bi2O2CO3, the main diffraction peak positions appear at 13.05°, 23.98°, 30.42°, 32.79°, 42.46°, 47.10°, 52.3° and 56.99°, which correspond to the (002), (011), (013), (110), (114), (020), (017) and (123) lattice planes of Bi2O2CO3.14 No characteristic peaks from other crystalline impurities can be observed, implying that the sample is highly pure. The XRD patterns of the BP, B/C0.5 and BP/C0.5 samples exhibit almost the same peak positions and shapes although with different intensities, suggesting that the modification of Bi2O2CO3 with PPy and g-C3N4 did not influence the lattice structure of Bi2O2CO3. In particular, no diffraction peaks attributed to PPy and g-C3N4 (for comparative purpose, the XRD patterns of PPy and g-C3N4 are also provided (Fig. S4 in ESI†)) can be observed, which also indicates that the amount of PPy and g-C3N4 is small, and is consistent with results of FTIR spectra. The intense and relatively sharp diffraction peaks imply the well crystallization of the samples. In addition, it should be noted that the intensity ratio of the (110) peak to the (013) peak of Bi2O2CO3 is higher than the standard value (0.386), indicating the anisotropic growth of Bi2O2CO3 along the (110) plane.35
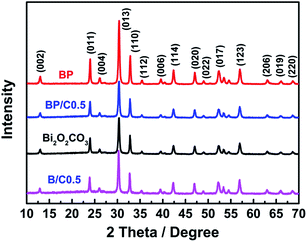 |
| Fig. 3 XRD patterns of Bi2O2CO3, BP, B/C0.5 and BP/C0.5. | |
The structure and morphologies of the as-prepared samples were further inspected with TEM. The obtained results are shown in Fig. 4 (for comparative purpose, the TEM spectra of PPy, g-C3N4 and B/C0.5 are also provided (Fig. S5 in ESI†)). Fig. 4a shows the typical TEM image of pure Bi2O2CO3. It can be seen that the Bi2O2CO3 products are composed of large scale, irregular platelet-like nanoplates with width of nanometer. For the sample BP (Fig. 4b), the rodlike PPy polymers (darker part) are visible on the Bi2O2CO3 surface (brighter part). For the BP/C0.5 composites (Fig. 4c), the platelet-like Bi2O2CO3, rodlike PPy and sheet-like g-C3N4 can be observed. Fig. 4d displays the EDS analysis of the BP/C0.5 composites. The samples contain bismuth, oxygen, carbon and nitrogen, confirming the formation of final BP/C0.5 composites. The elemental composition is corresponding to Bi2O2CO3, g-C3N4 and PPy. XPS analysis was carried out on the BP/C0.5 composites to further determine the surface chemical composition. The survey XPS spectrum (Fig. 5a) displays that the main elements on the surface of the sample are bismuth, oxygen, carbon and nitrogen. The asymmetrical and broad features of the observed C 1s XPS peaks (Fig. 5b) indicate the co-existence of distinguishable models.30,36,37 The curve fitting suggests chemically different C species in BP/C0.5 composites, with four C 1s binding energy peaks located at ca. 284.48, 285.08, 288.58, and 289.18 eV, respectively. The peak at 284.48 and 285.08 eV could be ascribed to the C–C and C
C functional groups and the peak with binding energy of 288.58 indicated the presence of sp2 C atoms bonded to aliphatic amine (–NH2 or –NH–) in the aromatic rings,38–40 while the peak observed at 289.18 eV is close to the literature value of the carbon of carbonate in Bi2O2CO3.41 The results of XPS analysis was in accordance with the EDS measurement, which further confirmed the coexistence of Bi2O2CO3, PPy and g-C3N4 in the BP/C0.5 materials. The Bi2O2CO3 and PPy attach on the g-C3N4 surface to assemble the BP/C0.5 composites. Actually, the layered nature favors the self-assembly of the units to form the characteristic platelet-like nanostructure of the materials. However, the Bi2O2CO3 and PPy assembled onto the g-C3N4 are not uniform (Fig. 4), and there is a stacking of irregular nanoplates on the surface of the sheet-like g-C3N4. The deposition of two-dimensional (2D) Bi2O2CO3 and PPy on the surface of 2D g-C3N4 nanoplates may induce tight contact. The BP composite can interact with the g-C3N4 sheets through physisorption, electrostatic binding and charge transfer interactions.42 As a result, the close interaction may enable fast transfer of electrons during photo-excitation processes. In addition, lager contact area can be obtained through the formation of three-component nanojunction system, which is expected to offer more adsorption sites. All of these would be beneficial to the transferring of charges, and may induce higher separation efficiency of photo-generated electron–hole pairs, thus enhance the photocatalytic activity.
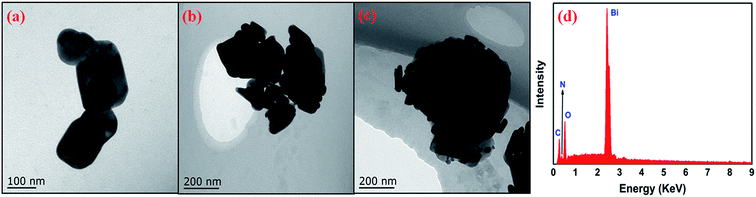 |
| Fig. 4 TEM images of (a) Bi2O2CO3, (b) BP, and (c) BP/C0.5, and (d) EDS spectrum of BP/C0.5. | |
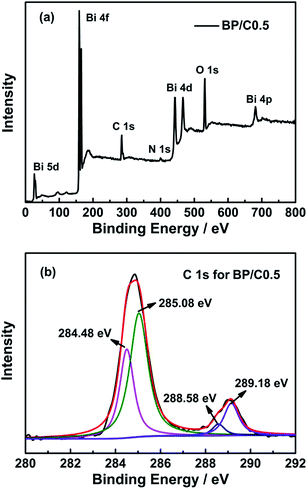 |
| Fig. 5 Survey XPS spectra (a) and high-resolution XPS spectra (b) of the as-prepared BP/C0.5. | |
3.2. Photocatalytic performance
g-C3N4 hybridized BP nanocomposites with different mass ratios from 0.1% to 2.0% were prepared to investigate the effect of g-C3N4 loading amount on the photocatalytic performances, which are denoted as BP/C0.1, BP/C0.25, BP/C0.5, BP/C1.0, BP/C1.5, and BP/C2.0, respectively. The photocatalytic performance of the as-prepared samples is investigated for the photodegradation of RhB under simulated sunlight irradiation after the adsorption–desorption equilibrium between the sample and RhB was achieved. Fig. 6 shows the temporal evolution of RhB concentration by the spots of C/C0 versus irradiation time in the presence of the as-prepared samples, where C is the concentration of the rest RhB, and C0 is the concentration of RhB solution after adsorption equilibrium. Before studying and comparing the activities of the as-prepared samples, the bleaching of RhB in the absence of any catalysts was first examined. Control experiment results suggest that the RhB degradation performance could be ignored in the absence of photocatalyst, suggesting that RhB is degraded via photocatalytic process.43 In addition, it seems very important to consider whether photocatalysis could still happen effectively after the adsorption of RhB to the as-prepared samples reached equilibrium. For Bi2O2CO3, only 46.6% of RhB can be photodegraded after 90 min irradiation, but about 62.8% of RhB was photodegraded for BP under the same conditions. This may be due to the rapid electron hole separation and slow recombination efficiency resulting from the synergic effect of PPy and Bi2O2CO3.14 The adding of g-C3N4 can dramatically influence the photoreactivity of BP. It can be found that the efficiency on the degradation of RhB by the prepared samples under simulated sunlight was quite different. The photocatalytic activities of the BP/C samples are a function of the mass ratios between g-C3N4 and BP. The photocatalytic activity increases with the increasing of g-C3N4 loading content from 0.1% to 0.5%. Further increase in g-C3N4 loading content leads to a decrease in the photocatalytic activity and negative trend was observed. For example, when the loading content of g-C3N4 is 1.5%, a dramatic decrease was observed in the photocatalytic activity. As expected, all of the BP/C composites exhibited higher activity than the pristine Bi2O2CO3. As the mass ratio of g-C3N4/BP increases to 0.5%, the highest photocatalytic efficacy is achieved and RhB was almost completely degraded after 90 min of simulated sunlight irradiation. In particular, the photocatalytic efficacy of BP/C0.5 is larger than those of BP and B/C0.5. All these results clearly confirm that the photocatalytic activity of Bi2O2CO3 can be significantly enhanced through coupling with a suitable amount of PPy and g-C3N4. Our results are in agreement with previous studies,44,45 in which suitable g-C3N4 content is crucial for optimizing the photocatalytic performance of the BP/C nanocomposites.
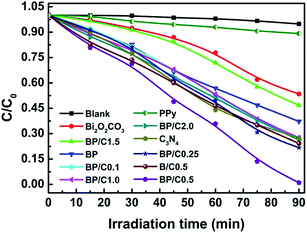 |
| Fig. 6 Photocatalytic performances of the as-prepared samples for the photodegradation of RhB aqueous solutions under ambient conditions. The relative standard deviation is less 0.65%. | |
For a better understanding of the photocatalytic efficiency at various illumination times, the photodegradation process of RhB was investigated by the UV-vis absorption spectra. Fig. 7 displays the time dependent spectroscopic changes occurred in the dyes catalyzed by using BP/C0.5 under light irradiation. It is clearly seen that the absorption band of RhB decreased gradually with a blue shift during photocatalytic degradation, which indicates cleavage of the whole conjugated structure in the degradation process, corresponding to the stepwise formation of a series of N-deethylated intermediates.46 Correspondingly, the pink color of the starting RhB solutions gradually faded during the process of photodegradation (not shown). The absorption maximum of these intermediates located at 554–505 nm in the visible spectral region. The photodegradation efficiencies of BP/C0.5 were found to increase gradually with increasing irradiation time, reaching the maximum degradation efficiency of 98.94% after 90 min. Most importantly, for the photodegradation of RhB by BP/C0.5, the peak intensity in the UV region (250 nm < λ < 370 nm) also decreased upon increasing irradiation time. This further suggests that the chromophoric structure of the dye was decomposed.
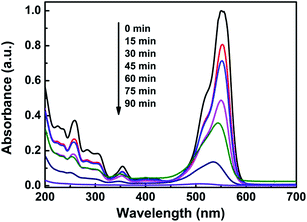 |
| Fig. 7 UV-vis spectral changes of RhB aqueous solutions in the presence of the BP/C0.5 sample as a function of the irradiation time. | |
Based on the key principles of green chemistry, the effective catalysis and catalytic recovery were crucial to feasibility of the wastewater treatment process. Thus, estimating the recyclable performance of photocatalyst is indispensable for its possible continuous use in the practical applications of the BP/C0.5 composites. The separated photocatalysts were washed with deionized water and dried after every 90 min of photodegradation. Fig. 8 shows the photocatalytic stabilities of BP/C0.5 evaluated in a recycle test of five runs under identical conditions. As shown in Fig. 8, during the first 2 cycles, the photocatalytic degradation efficiency was 98.94% and 97.85%, respectively. The catalytic activity slightly dropped in runs 3 and 4, giving 95.62% and 94.60% degradation efficiency, respectively. In the 5th run, the catalyst exhibited 92.61% degradation efficiency. The lowering of photocatalytic efficiency may be due not only to some leaching of active component and/or weight loss during the repeated filtration process to separate the catalyst from RhB solution, but also to the accumulation of residual RhB in the inner pores.13,42,47 The results indicate that the as-prepared BP/C0.5 sample remains effective after five cycles within a relative standard deviation of 0.76%, and therefore it is basically stable for the photosensitized degradation of organic pollutants under simulated sunlight irradiation and promising for environmental remediation.
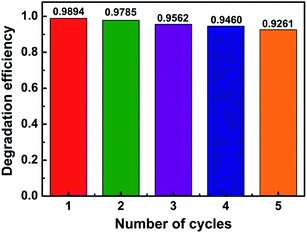 |
| Fig. 8 The photocatalytic stabilities of RhB degradation over BP/C0.5. | |
3.3. Possible mechanism of photocatalytic activity enhancement
To probe the mechanism on the enhancement of photocatalytic activity for the BP/C0.5 composites, several experiments are carried out. The good photocatalytic efficiency may be ascribed to three factors. First of all, surface area of a nanomaterial is closely related to the number of adsorption sites as well catalytic activities, which is an indispensable factor in photocatalysis.48 The specific surface area and porosity of the as-prepared samples were investigated by nitrogen adsorption–desorption measurement. As shown in Fig. 9, a typical IV isotherm with H3-type hysteresis is observed for all samples, indicating that the existence of mesoporous structure.49 The specific surface area of BP, B/C0.5 and BP/C0.5 were calculated to be 11.469, 15.065, and 17.142 m2 g−1, respectively, which are larger than pure Bi2O2CO3 (7.753 m2 g−1). The large surface area of BP/C0.5 benefiting from the special hierarchical structure is expected to accelerate the photocatalytic activity by providing more active sites and promoting the separation efficiency of photocarriers. In addition, the Barrett–Joyner–Halenda (BJH) method was employed to determine the pore size distributions of Bi2O2CO3, BP, B/C0.5, and BP/C0.5. The pore size distribution is mono-modal for all samples. The mesoporous in the samples most likely originated from their inter-nanosheet spacing, which is in accordance with the TEM analysis.
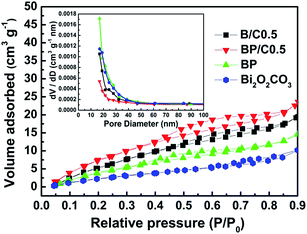 |
| Fig. 9 Nitrogen adsorption–desorption isotherms and the corresponding pore-size distribution curves (inset) of Bi2O2CO3, BP, B/C0.5 and BP/C0.5. | |
Second, the improved light-harvesting ability can be considered to be one major reason for the superior photocatalytic performance of the BP/C0.5 sample.50 The light absorption property of g-C3N4, Bi2O2CO3, BP, B/C0.5 and BP/C0.5 is investigated by UV-vis diffuse reflectance spectra (for comparative purpose, the UV-vis diffuse reflectance spectrum of PPy is also provided (Fig. S6 in ESI†)). As shown in Fig. 10, The pure Bi2O2CO3 displays significant light absorption in the UV region, and has almost no absorption at λ > 350 nm. However, a slight red shift in the UV region and an obvious absorption in the visible light region were observed for BP compared to Bi2O2CO3, which may lead to a possible charge-transfer transition at the interface between PPy and Bi2O2CO3.14 As a consequence, the BP composites can be easier to produce electron–hole pairs under the same light irradiation and exhibit improved photocatalytic performance. However, only a slight change was observed for B/C0.5 when compared to Bi2O2CO3. The pristine g-C3N4 exhibits a fundamental absorption edge at 450 nm, originating from the charge transfer response of g-C3N4 from the valence band populated by N 2p orbital to the conduction band formed by C 2p orbital.51 After the introduction of g-C3N4 in the BP composites, the visible absorption intensities of the BP/C0.5 composites are obviously enhanced and the absorption edge also displays a slight red shift, which may allow for more utilization of the solar spectrum to produce photogenerated electrons and holes.
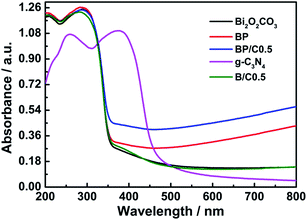 |
| Fig. 10 UV-vis diffuse reflection spectra of g-C3N4, Bi2O2CO3, BP, B/C0.5 and BP/C0.5. | |
Third, the charge separation and migration also play a very important role on the photocatalytic reaction process.52 Thus, the photocurrent response and electrochemical impedance experiments were performed to investigate the photogenerated charge carrier behaviors. Fig. 11 shows the photocurrent responses of Bi2O2CO3, BP, g-C3N4, PPy, B/C0.5 and BP/C0.5 after deposition on ITO under the simulated sunlight irradiation. The rise and fall of the photocurrent response are apparent for each switch-on/off cycle in all the electrodes (Bi2O2CO3, BP, g-C3N4, PPy, B/C0.5 and BP/C0.5). The photocurrent transients have different shape for each electrode. As forecasted, the weak photocurrent density of pure Bi2O2CO3 can be detected due to the large energy gap.53 In contrast to Bi2O2CO3, PPy and g-C3N4, the BP, B/C0.5 and BP/C0.5 composites exhibit a remarkably enhanced photocurrent response. By comparison, the highest photocurrent intensity was observed for BP/C0.5, which is about 3.75 times as high as that of Bi2O2CO3 and 1.75 times of BP. The trend of photocurrent intensity is clearly consistent with the photocatalytic activity. The increased photocurrent intensity implies that more electrons are transferred to photoelectrode, indicating greater separation of the photoinduced electrons and holes.12,47,54
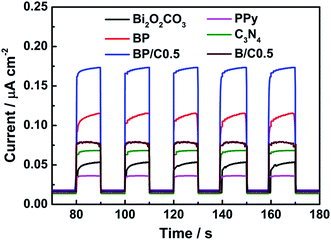 |
| Fig. 11 The photocurrent responses of Bi2O2CO3, BP, g-C3N4, PPy, B/C0.5 and BP/C0.5. | |
Fig. 12 shows the electrochemical impedance spectra Nyquist plots of pure BiO2CO3, BP and BP/C0.5 composites. The arc radii of BP/C0.5 electrode are much smaller than those of Bi2O2CO3 and BP electrodes. It should be noted that the large arc radius observed for the electrode is indicative of a high interfacial charge-transfer resistance, due to the poor electrical conductivity of materials, whereas the smaller arc radius corresponds to an electrode closer to the ideal capacitor.30,55 Thus, the reduced arc radius for BP/C0.5 indicates diminished resistance of working electrodes, implying a decrease in the solid state interface layer resistance. Furthermore, the arc radius on the electrochemical impedance spectra Nyquist plot also reveals reaction rate occurring at the surface of the electrodes.56 It is therefore that a more effective separation of photogenerated electron–hole pairs and a faster interfacial charge transfer occurs on BP/C0.5 surface under this condition, agreeing well with the photocurrent measurements. The efficiency of charge trapping and recombination of photoinduced electron–hole pairs for the as-prepared samples is further investigated by the photoluminescence spectra, which is of importance to evaluate the photocatalytic response of the semiconductor materials. A low photoluminescence intensity is generally indicative of a high separation efficiency of electron–hole pairs.57,58 As shown in Fig. 13, the similar photoluminescence peaks were observed for the as-prepared samples (BiO2CO3, BP and BP/C0.5). Specifically, BP/C0.5 exhibits an obviously decreased emission signal when compared to BiO2CO3 and BP. These results demonstrate that the introduction of g-C3N4 and PPy in BiO2CO3 really decreases the carrier recombination rate and improves the separation efficiency of photogenerated electrons and holes. So the high photocatalytic performance of BP/C0.5 can be ascribed to the efficient separation of electron–hole pairs.
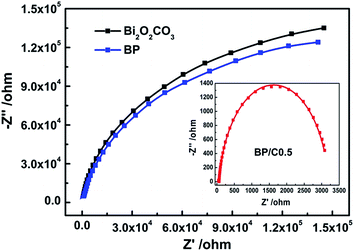 |
| Fig. 12 Electrochemical impedance spectra of Bi2O2CO3, BP and BP/C0.5. | |
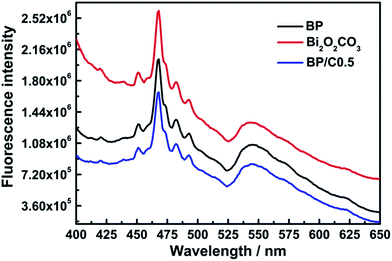 |
| Fig. 13 Photoluminescence spectra of the pure Bi2O2CO3, BP and BP/C0.5 samples upon excitation at 360 nm. | |
According to the above experimental results, the enhanced photocatalytic performance of the BP/C0.5 composites can be ascribed to the combined effect of several factors. In the case of PPy, two ways can be presented to explain the enhanced photon efficiency.14 On the one hand, PPy as a hole acceptor may produce a series of actives species on its surface under simulated sunlight irradiation.13 On the other hand, the high mobility of charge carriers can be expected in the circulatory system of BP/C0.5. As a semiconducting material, PPy could absorb the UV light, producing photogenerated carriers and consequently induced electron transition from the highest occupied molecular orbital to the lowest unoccupied molecular orbital, while the holes will be left in the highest occupied molecular orbital of PPy.59 The electrons in the valence band of BiO2CO3 can migrate to the highest occupied molecular orbital of PPy to recombine with holes, and the holes are generated in the valence band of BiO2CO3. For g-C3N4, it can be excited by the simulated sunlight and produce photogenerated electrons and holes.60 Similar to PPy, the weak holes excited from g-C3N4 could also be quickly transferred to Bi2O2CO3, which is faster than the electron–hole recombination between the valence band and conduction band of g-C3N4.43 Then the photogenerated electrons and holes could annihilate at Bi2O2CO3. That is to say the Bi2O2CO3 paired between the g-C3N4 and PPy can participate in the transition process of the photogenerated charge carrier. Meanwhile, the strong reductive photoelectrons would be left on the conduction band of PPy, and the strong oxidizing holes would be left on the valence band of g-C3N4. It is therefore that the photogenerated electron–holes pairs will be separated effectively in the circulatory system of BP/C0.5, and the recombination of electron–hole pairs can be reduced, which favor the interfacial charge transfer. The separated electrons and holes are then free to initiate reactions with the reactants adsorbed on the photocatalyst surfaces, resulting in enhanced photocatalytic performance of the BP/C0.5 composites.
3.4. Nonlinear optical properties
The efficient separation of electron–hole pairs and charge transfer implies that these nanostructures are candidates for optical limiting applications.18,25 Nonlinear optical responses can arise from charge-transfer complexes due to the possibility of excited absorption, free carrier absorption, exciton absorption, multiphoton absorption and nonlinear scattering, which can give rise to reverse saturable absorption effects in optical limiting.27,61 To assess this possibility, Z-scan experiments employing 4 ns laser pulses at 532 nm have been performed on the Bi2O2CO3, BP and BP/C0.5 samples dispersed in DMSO. To facilitate comparison, all sample concentrations were adjusted to 0.2 mg mL−1.
Fig. 14 contains the open-aperture Z-scan traces of Bi2O2CO3, BP and BP/C0.5 using 4 ns laser pulses. As shown in Fig. 14, all samples display decreased transmittances as they are brought closer to the focal point (z = 0), the extent of the reduction varying with the on-focus intensity, which is consistent with a strong nonlinear optical absorptive effect with 4 ns laser pulse excitation. The valleys of the transmittance scans indicate that laser pulses experience a strong reverse absorption behavior in all of the tested samples, typical of an induced positive nonlinear absorption of incident light.62,63 Materials exhibiting such performance are suitable candidates as optical limiters for protection of eyes and optical sensors from intense laser pulses. For an optical limiter, the depth of the valley in the open-aperture Z-scan data is in line with the optical limiting merit.64 Although efficient nonlinear optical properties were observed for the three samples, the greater decreased valley observed for BP/C0.5 among the transmittance curves indicate that it should have the best optical limiting performance. In addition, when the PPy is lightly introduced to Bi2O2CO3, the nonlinear optical absorption efficiency of the resultant composite (BP) is somewhat improved, but not so remarkably.
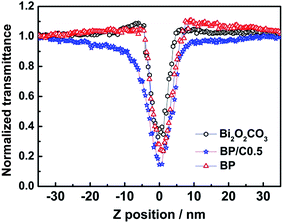 |
| Fig. 14 Open-aperture Z-scan traces of Bi2O2CO3, BP and BP/C0.5 in DMSO, obtained under 4 ns, 532 nm laser excitation. | |
The nonlinear refractive components were investigated by dividing the normalized Z-scan data obtained from the closed-aperture configuration, the results being shown in Fig. 15. The prefocal valley followed by postfocal peak indicates that the sign of refractive index is positive, corresponding to a self-focusing effect and thus positive nonlinearity.65 Observation of negative nonlinearity upon excitation with ns pulses is common and is ascribed to the thermal effects generated by high fluence of ns laser pulses, so the origin of the self-focusing effect for the three samples is other than the thermal effect.66 The positive nonlinear refraction may be assigned to the population transitions among the singlet states, the refraction volume of the singlet excited state is larger than that of the ground state.65 It is clear that the differences between the normalized transmittance values and valley positions for BP/C0.5 and BP are larger than that of Bi2O2CO3, indicating that the BP/C0.5 and BP composites possess larger nonlinear refraction response than the pristine Bi2O2CO3 (Fig. 15), presumably a result of the introduction of PPy and g-C3N4. Particularly, the BP/C0.5 composites possess larger nonlinear refractive index than BP, which is consistent with the results of nonlinear optical absorption response (Fig. 14).
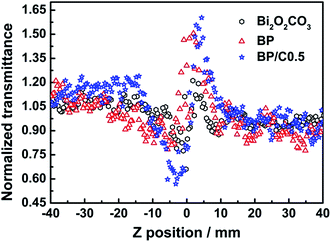 |
| Fig. 15 Closed-aperture Z-scan traces of Bi2O2CO3, BP and BP/C0.5 in DMSO, with 4 ns pulse durations. | |
Because Bi2O2CO3, BP and BP/C0.5 exhibit significant linear absorption at the excitation wavelength (532 nm) used in the Z-scan experiment, which can be evident from the UV-visible absorption spectra (Fig. 10), the efficient nonlinear optical performance may be more reasonable to be ascribed to the photo-induced free carrier (hole–electron pairs) absorption generated by interband transitions.61,67 When the samples are excited with the nanosecond laser beams at 532 nm, interband transitions will generate free carriers in the lowest unoccupied molecular orbital level. The generated free carriers have a tendency to absorb additional photons through a photon-assisted process, and thus a strong nonlinear optical response is facilitated through this free carrier absorption phenomenon.25,68 In addition, besides the free carrier absorption, we have observed nonlinear scattering performance in the nanosecond regime exhibited by the BP/C0.5 composites, and we think the contribution is minimal since it is not as dominant as seen in other nanostructures like graphene suspensions.69 Similar to nonlinear scattering, the reverse absorption performance originating from PPy and g-C3N4 may also play a role to somewhat for the improved optical nonlinearity,24,25 though their content is very low in the as-prepared composites. Another possible reason for the enhanced nonlinear optical performance for BP/C0.5 in comparison to those of Bi2O2CO3 and BP may be assigned to an intersystem excitation transfer mechanism, typical via the effective charge transfer process, contributes to increasing the free carrier population,70 and this results in the fluorescence quenching and energy releasing, and therefore the limiting action. The effective charge transfer process can be supported by the results of fluorescence, photocurrent response and electrochemical impedance experiments (Fig. 11–13). Therefore, the improved nonlinear optical performances for the BP/C0.5 composites should arise from a combination of mechanisms.
4. Conclusions
In this work, a series of novel Bi2O2CO3/PPy/g-C3N4 composites loaded with different g-C3N4 contents was prepared by a facile refluxing process, and characterized by FTIR, XRD, Raman, XPS and TEM. Sensitization of Bi2O2CO3 with PPy and g-C3N4 broadened the light absorption range of Bi2O2CO3, which has been utilized for photocatalytic and optical limiting studies. Among the as-prepared samples, the BP/C0.5 composites exhibit the highest photocatalytic performance, and a total photodegradation efficiency of 98.94% towards RhB was achieved within 90 min. In addition, g-C3N4 loadings larger than 0.5% result in a decrease in photocatalytic activity. The improved photocatalytic activity of BP/C0.5 can be ascribed mainly to its better light absorption property, large surface area and efficient separation efficiency of photogenerated electron–hole pairs, which are demonstrated by the results of nitrogen adsorption–desorption isotherms, UV-vis diffuse reflectance spectra, fluorescence, photocurrent response and electrochemical impedance experiments. The composite photocatalysts could be effectively recycled and re-used for five times without significant change in photocatalytic performance, which evidenced the catalyst stability as well as the repeatability of the present approach. The viability of the Bi2O2CO3/PPy/g-C3N4 composites as nonlinear optical materials has been examined using the Z-scan technique at 532 nm with 4 laser ns pulses. The BP/C0.5 composites are found to exhibit enhanced nonlinear optical properties compared to Bi2O2CO3 and BP due to a combination of mechanisms. This composite is a promising candidate for optical limiting, solar-energy conversion and storage applications. This work opens new possibilities to provide some insights into the design of novel nanoscale stable materials with photocatalytic and optical limiting properties.
Acknowledgements
This research was supported financially by the National Key Technology Support Program (2015BAD21B06), the National Natural Science Foundation of China (51506077, 21171076, 51602130), the Natural Science Foundation of Jiangsu Province (BK20150488), the Natural Science Foundation of Jiangsu High School (15KJB430007, 15KJB610003, 15KJD150002, 16KJD430002), the China Postdoctoral Foundation (2016M601733) and Research Foundation of Jiangsu University (13JDG066, 15JDG156, 1283300004).
References
- Y. S. Xu and W. D. Zhang, Anion exchange strategy for construction of sesame-biscuit-like Bi2O3CO3/Bi2MoO6 nanocomposites with enhanced photocatalytic activity, Appl. Catal., B, 2013, 140–141, 306–316 CrossRef CAS.
- Y. Bai, L. Q. Ye, L. Wang, X. Shi, P. Q. Wang, W. Bai and P. K. Wong, g-C3N4/Bi4O5I2 heterojunction with I3-/I− redox mediator for enhanced photocatalytic CO2 conversion, Appl. Catal., B, 2016, 194, 98–104 CrossRef CAS.
- Y. L. Zhang, D. Y. Li, Y. G. Zhang, X. F. Zhou, S. J. Guo and L. B. Yang, Graphene-wrapped Bi2O2CO3 core-shell structures with enhanced quantum efficiency profit from an ultrafast electron transfer process, J. Mater. Chem. A, 2014, 2, 8273–8280 CAS.
- J. L. Lv, K. Dai, J. F. Zhang, L. Geng, C. H. Liang, Q. C. Liu, G. P. Zhu and C. Chen, Facile synthesis of Z-scheme graphitic-C3N4/Bi2MoO6 nanocomposite for enhanced visible photocatalytic properties, Appl. Surf. Sci., 2015, 358, 377–384 CrossRef CAS.
- D. Yuan, L. Y. Huang, Y. P. Li, Y. G. Xu, H. Xu, S. Q. Huang, J. Yan, M. Q. He and H. M. Li, Synthesis and photocatalytic activity of g-C3N4/BiOI/BiOBr ternary composites, RSC Adv., 2016, 6, 41204–41213 RSC.
- Z. L. Ni, Y. J. Sun, Y. X. Zhang and F. Dong, Fabrication, modification and application of (BiO)2CO3-based photocatalysts: A review, Appl. Surf. Sci., 2016, 365, 314–335 CrossRef CAS.
- T. Xiong, H. W. Huang, Y. J. Sun and F. Dong, In situ synthesis of a C-doped (BiO)2CO3 hierarchical self-assembly effectively promoting visible light photocatalysis, J. Mater. Chem. A, 2015, 3, 6118–6127 CAS.
- P. Madhusudan, J. R. Ran, J. Zhang, J. G. Yu and G. Liu, Novel urea assisted hydrothermal synthesis of hierarchical BiVO4/Bi2O2CO3 nanocomposites with enhanced visible-light photocatalytic activity, Appl. Catal., B, 2011, 110, 286–295 CrossRef CAS.
- C. M. Li, G. Chen, J. X. Sun, Y. J. Feng and H. J. Dong, Ultrathin nanoflakes constructed erythrocyte-like Bi2WO6 hierarchitecture via anionic self-regulation strategy for improving photocatalytic activity and gas-sensing property, Appl. Catal., B, 2015, 163, 415–423 CrossRef CAS.
- Y. H. Ao, L. Y. Xu, P. F. Wang, C. Wang, J. Hou, J. Qian and Y. Li, Graphene and TiO2-modified flower-like Bi2O2CO3: A novel multi-heterojunction photocatalyst with enhanced photocatalytic activity, Appl. Surf. Sci., 2015, 355, 411–418 CrossRef CAS.
- J. Q. Li, H. Yuan and Z. F. Zhu, Photoelectrochemical performances of g-C3N4/Au/BiPO4 Z-scheme composites to improve the mineralization property under solar light, RSC Adv., 2016, 6, 70563–70572 RSC.
- M. Yan, F. F. Zhu, W. Gu, L. Sun, W. D. Shi and Y. Q. Hua, Construction of nitrogen-doped graphene quantum dots-BiVO4/g-C3N4 Z-scheme photocatalyst and enhanced photocatalytic degradation of antibiotics under antibiotics under visible light, RSC Adv., 2016, 6, 61162–61174 RSC.
- F. A. Harraz, A. A. Ismail, S. A. Al-Sayari and A. Al-Hajry, Novel α-Fe2O3/polypyrrole nanocomposite with enhanced photocatalytic performance, J. Photochem. Photobiol., A, 2015, 299, 18–24 CrossRef CAS.
- Q. Z. Wang, L. H. Zheng, Y. T. Chen, J. F. Fan, H. H. Huang and B. T. Su, Synthesis and characterization of novel PPy/Bi2O2CO3 composite with improved photocatalytic activity for degradation of Rhodamine-B, J. Alloys Compd., 2015, 637, 127–132 CrossRef CAS.
- B. C. Zhu, P. F. Xia, W. K. Ho and J. G. Yu, Isoelectric point and
adsorption activity of porous g-C3N4, Appl. Surf. Sci., 2015, 344, 188–195 CrossRef CAS.
- J. Q. Wen, J. Xie, X. B. Chen and X. Lin, A review on g-C3N4-based photocatalysts, Appl. Surf. Sci., 2017, 391, 72–123 CrossRef CAS.
- L. Shi, J. L. Gou, L. Liang, F. X. Wang and J. M. Sun, The crystal phase transformation of Ag2WO4 through loading onto g-C3N4 sheets with enhanced visible-light photocatalytic activity, RSC Adv., 2016, 6, 96861–96869 RSC.
- J. Balapanuru, J. X. Yang, S. Xiao, Q. L. Bao, M. Jahan, L. Polavarapu, J. Wei, Q. H. Xu and K. P. Loh, A graphene oxide-organic dye ionic complex with DNA-sensing and optical limiting properties, Angew. Chem., Int. Ed., 2010, 49, 6549–6553 CrossRef CAS PubMed.
- Y. F. Xu, Z. B. Liu, X. L. Zhang, Y. Wang, J. G. Tian, Y. Huang, Y. F. Ma, X. Y. Zhang and Y. S. Chen, A graphene hybrid material covalently functionalized with porphyrin: Synthesis and optical limiting property, Adv. Mater., 2009, 21, 1275–1279 CrossRef CAS.
- J. H. Zhu, Y. X. Li, Y. Chen, J. Wang, B. Zhang, J. J. Zhang and W. J. Blau, Graphene oxide covalently functionalized with zinc phthalocyanine for broadband optical limiting, Carbon, 2011, 49, 1900–1905 CrossRef CAS.
- A. P. R. Mary, C. S. S. Sandeep, T. N. Narayanan, R. Philip, M. Padraig, P. M. Ajayan and M. R. Anantharaman, Nonlinear and magneto-optical transmission studies on magnetic nanofluids of non-interacting metallic nickel nanoparticles, Nanotechnology, 2011, 22, 375702 CrossRef PubMed.
- G. L. Chai, C. S. Lin, J. Wei, M. Y. Zhang and W. D. Cheng, Nonlinear optical properties of carbon nitride nanotubes, Phys. Chem. Chem. Phys., 2012, 14, 835–839 RSC.
- H. W. Huang, N. Tian, S. F. Jin, Y. H. Zhang and S. B. Wang, Syntheses, characterization and nonlinear optical properties of a bismuth subcarbonate Bi2O2CO3, Solid State Sci., 2014, 30, 1–5 CrossRef CAS.
- A. J. Wang, W. Zhao and W. Yu, Effect of acid/base on the third-order optical nonlinearity of polypyrrole, J. Mol. Struct., 2015, 1099, 291–296 CrossRef CAS.
- K. Sridharan, P. Sreekanth, T. J. Park and R. Philip, Nonlinear optical investigations in nine-atom silver quantum clusters and graphitic carbon nitride nanosheets, J. Phys. Chem. C, 2015, 119, 16314–16320 CAS.
- S. Abed, K. Bouchouit, M. S. Aida, S. Taboukhat, Z. Sofiani, B. Kulyk and V. Figa, Nonlinear optical properties of zinc oxide doped bismuth thin films using Z-scan technique, Opt. Mater., 2016, 56, 40–44 CrossRef CAS.
- K. Sridharan, T. Kuriakose, R. Philip and T. J. Park, Transition metal (Fe, Co and Ni) oxide nanoparticles grafted graphitic carbon nitrides as efficient optical limiters and recyclable photocatalysts, Appl. Surf. Sci., 2014, 308, 139–147 CrossRef CAS.
- M. R. Gholipour, G. T. Dinh, F. Béland and T. O. Do, Nanocomposite heterojunctions as sunlight-driven photocatalysts for hydrogen production from water splitting, Nanoscale, 2015, 7, 8187–8208 RSC.
- X. Wang, K. Maeda, A. Thomas, K. Takanabe, G. Xin, J. M. Carlsson, K. Domen and M. Antonietti, A metal-free polymeric photocatalyst for hydrogen production from water under visible light, Nat. Mater., 2009, 8, 76–80 CrossRef CAS PubMed.
- P. Madhusudan, J. G. Yu, W. G. Wang, B. Cheng and G. Liu, Facile synthesis of novel hierarchical graphene-Bi2O2CO3 composites with enhanced photocatalytic performance under visible light, Dalton Trans., 2012, 41, 14345–14353 RSC.
- W. L. Cen, T. Xiong, C. Y. Tang, S. D. Yuan and F. Dong, Effects of morphology and crystallinity on the photocatalytic activity of (BiO)2CO3 nano/microstructures, Ind. Eng. Chem. Res., 2014, 53, 15002–15011 CrossRef CAS.
- N. Tian, H. W. Huang, Y. X. Guo, Y. He and Y. H. Zhang, A g-C3N4/Bi2O2CO3 composite with high visible-light-driven photocatalytic activity for rhodamine B degradation, Appl. Surf. Sci., 2014, 322, 249–254 CrossRef CAS.
- R. Keuleers, H. O. Desseyn, B. Rousseau and C. Van Alsenoy, Vibrational analysis of urea, J. Phys. Chem. A, 1999, 103, 4621–4630 CrossRef CAS.
- G. E. Tobon-Zapata, S. B. Etcheverry and E. J. Baran, Vibrational spectrum of bismuth subcarbonate, J. Mater. Sci. Lett., 1997, 16, 656–657 CrossRef CAS.
- H. Cheng, B. Huang, K. Yang, Z. Wang, X. Qin, X. Zhang and Y. Dai, Facile template-free synthesis of Bi2O2CO3 hierarchical microflowers and their associated photocatalytic activity, ChemPhysChem, 2010, 11, 2167–2173 CrossRef CAS PubMed.
- J. Di, J. X. Xia, Y. Huang, M. X. Ji, W. M. Fan, Z. G. Chen and H. M. Li, Constructing carbon quantum dots/Bi2SiO5 ultrathin nanosheets with enhanced photocatalytic activity and mechanism investigation, Chem. Eng. J., 2016, 302, 334–343 CrossRef CAS.
- J. Di, J. X. Xia, M. X. Ji, L. Xu, S. Yin, Q. Zhang, Z. G. Chen and H. M. Li, Carbon quantum dots in situ coupling to bismuth oxyiodide via reactable ionic liquid with enhanced photocatalytic molecular oxygen activation performance, Carbon, 2016, 98, 613–623 CrossRef CAS.
- Y. Z. Hong, C. S. Li, G. Y. Zhang, Y. D. Meng, B. X. Yin, Y. Zhao and W. D. Shi, Efficient and stable Nb2O5 modified g-C3N4 photocatalyst for removal of antibiotic pollutant, Chem. Eng. J., 2016, 299, 74–84 CrossRef CAS.
- J. X. Xia, M. X. Ji, J. Di, B. Wang, S. Yin, Q. Zhang, M. Q. He and H. M. Li, Construction of ultrathin C3N4/Bi4O5I2 layered nanojunctions via ionic liquid with enhanced photocatalytic performance and mechanism insight, Appl. Catal., B, 2016, 191, 235–245 CrossRef CAS.
- S. Q. Huang, Y. G. Xu, M. Xie, H. Xu, M. Q. He, J. X. Xia, L. Y. Huang and H. M. Li, Synthesis of magnetic CoFe2O4/g-C3N4 composite and its enhancement of photocatalytic ability under visible-light, Colloids Surf., A, 2015, 478, 71–80 CrossRef CAS.
- Z. Y. Zhao, Y. Zhou, F. Wang, K. H. Zhang, S. Yu and K. Cao, Polyaniline-decorated {001} facets of Bi2O2CO3 nanosheets: In situ oxygen vacancy formation and enhanced visible light photocatalytic activity, ACS Appl. Mater. Interfaces, 2015, 7, 730–737 CAS.
- M. Xiong, L. Chen, Q. Yuan, J. He, S. L. Luo, C. T. Au and S. F. Yin, Facile fabrication and enhanced photosensitized degradation performance of the g-C3N4-Bi2O2CO3 composite, Dalton Trans., 2014, 43, 8331–8337 RSC.
- Q. Zhang, H. Y. Wang, S. Z. Hu, G. Lu, J. Bai, X. X. Kang, D. Liu and J. Z. Gui, Synthesis and properties of visible light responsive g-C3N4/Bi2O2CO3 layered herterojunction nanocomposites, RSC Adv., 2015, 5, 42736–42743 RSC.
- X. J. Wang, Q. Wang, F. T. Li, W. Y. Yang, Y. Zhao, Y. J. Hao and S. J. Liu, Novel BiOCl-C3N4 heterojunction photocatalysts:
In situ preparation via an ionic-liquid-assisted solvent-thermal route and their visible-light photocatalytic activities, Chem. Eng. J., 2013, 234, 361–371 CrossRef CAS.
- C. S. Pan, J. Xu, Y. J. Wang, D. Li and Y. F. Zhu, Dramatic activity of C3N4/BiPO4 photocatalyst with core/shell structure formed by self-assembly, Adv. Funct. Mater., 2012, 22, 1518–1524 CrossRef CAS.
- Y. S. Xu, Z. J. Zhang and W. D. Zhang, Facile preparation of heterostructured Bi2O3/Bi2MoO6 hollow microspheres with enhanced visible-light-driven photocatalytic and antimicrobial activity, Mater. Res. Bull., 2013, 48, 1420–1427 CrossRef CAS.
- D. Wang, Y. Xu, F. Sun, Q. H. Zhang, P. Wang and X. Y. Wang, Enhanced photocatalytic activity of TiO2 under sunlight by MoS2 nanodots modification, Appl. Surf. Sci., 2016, 377, 221–227 CrossRef CAS.
- J. Di, J. X. Xia, M. X. Ji, B. Wang, S. Yin, H. Xu, Z. G. Chen and H. M. Li, Carbon quantum dots induced ultrasmall BiOI nanosheets with assembled hollow structures for broad spectrum photocatalytic activity and mechanism insight, Langmuir, 2016, 32, 2075–2084 CrossRef CAS PubMed.
- J. Di, J. X. Xia, X. W. Li, M. X. Ji, H. Xu, Z. G. Chen and H. M. Li, Constructing confined surface carbon defects in ultrathin graphitic carbon nitride for photocatalytic free radical manipulation, Carbon, 2016, 107, 1–10 CrossRef CAS.
- W. B. Li, C. Feng, S. Y. Dai, J. G. Yue, F. X. Hua and H. Hou, Fabrication of sulfur-doped g-C3N4/Au/CdS Z-scheme photocatalyst to improve the photocatalytic performance under visible light, Appl. Catal., B, 2015, 168–169, 465–471 CrossRef CAS.
- S. W. Cao, J. X. Low, J. G. Yu and M. Jaroniec, Polymeric photocatalysts based on graphitic carbon nitride, Adv. Mater., 2015, 27, 2150–2176 CrossRef CAS PubMed.
- K. H. Ye, Z. S. Cha, J. W. Gu, X. Yu, C. X. Zhao, Y. M. Zhang and W. J. Mai, BiOI-BiVO4 photoanodes with significantly improved solar water splitting capability: p–n junction to expand solar adsorption range and facilitate charge carrier dynamics, Nano Energy, 2015, 18, 222–231 CrossRef CAS.
- Z. Y. Wang, Y. Huang, W. K. Ho, J. J. Cao, Z. X. Shen and S. C. Lee, Fabrication of Bi2O2CO3/g-C3N4 heterojunctions for efficiently photocatalytic NO in air removal: In-situ self-sacrificial synthesis, characterizations and mechanistic study, Appl. Catal., B, 2016, 199, 123–133 CrossRef CAS.
- G. P. Dai, J. G. Yu and G. Liu, Synthesis and enhanced visible-light photoelectrocatalytic activity of p–n junction BiOI/TiO2 nanotube arrays, J. Phys. Chem. C, 2011, 115, 7339–7346 CAS.
- J. Xu, Y. J. Wang and Y. F. Zhu, Nanoporous graphitic carbon nitride with enhanced photocatalytic performance, Langmuir, 2013, 29, 10566–10572 CrossRef CAS PubMed.
- F. C. Lei, Y. F. Sun, K. T. Liu, S. Gao, L. Liang, B. C. Pan and Y. Xie, Oxygen vacancies confined in ultrathin indium oxide porous sheets for promoted visible-light water splitting, J. Am. Chem. Soc., 2014, 136, 6826–6829 CrossRef CAS PubMed.
- Y. H. Ao, J. Q. Bao, P. F. Wang, C. Wang and J. Hou, Bismuth oxychloride modified titanium phosphate nanoplates: A new p–n type heterostructured photocatalyst with high activity for the degradation of different kinds of organic pollutants, J. Colloid Interface Sci., 2016, 476, 71–78 CrossRef CAS PubMed.
- X. Zhang, P. Zhang, L. J. Wang, H. Q. Gao, J. T. Zhao, C. H. Liang, J. H. Hu and G. S. Shao, Template-oriented synthesis of monodispersed SnS2@SnO2 hetero-nanoflowers for Cr(VI) photoreduction, Appl. Catal., B, 2016, 192, 17–25 CrossRef CAS.
- H. Zhang, R. L. Zong, J. C. Zhao and Y. F. Zhu, Dramatic visible photocatalytic degradation performances due to synergetic effect of TiO2 with PANI, Environ. Sci. Technol., 2008, 42, 3803–3807 CrossRef CAS PubMed.
- Y. B. Li, H. M. Zhang, P. R. Liu, D. Wang, Y. Li and H. J. Zhao, Cross-linked g-C3N4/rGO nanocomposites with tunable band structure and enhanced visible light photocatalytic activity, Small, 2013, 9, 3336–3344 CAS.
- L. W. Tutt and T. F. Boggess, A review of optical limiting mechanisms and devices using organics, fullerenes, semiconductors and other materials, Prog. Quantum Electron., 1993, 17, 299–338 CrossRef CAS.
- J. Y. Yang, Y. L. Song, W. J. Zhu, X. Y. Su and H. Y. Xu, Investigation of optical nonlinearities and transient dynamics in a stilbenzene derivative, J. Phys. Chem. B, 2012, 116, 1221–1225 CrossRef CAS PubMed.
- G. H. Li, A. J. Wang, J. B. Song and Q. Zhou, A novel zinc tetraphenylporphyrinate substituted in the axial position with one E-stilbazole: Synthesis, structure, and nonlinear optics, Inorg. Chem. Commun., 2015, 57, 47–50 CrossRef CAS.
- Z. Guo, F. Du, D. M. Ren, Y. S. Chen, J. Y. Zheng, Z. B. Liu and J. G. Tian, Covalently porphyrin-functionalized single-walled carbon nanotubes: a novel photoactive and optical limiting donor-acceptor nanohybrid, J. Mater. Chem., 2016, 16, 3021–3030 RSC.
- L. Jiang, T. G. Jiu, Y. L. Li, Y. B. Li, J. Y. Yang, J. B. Li, C. H. Li, H. B. Liu and Y. L. Song, Excited-state absorption and sign tuning of nonlinear refraction in porphyrin derivatives, J. Phys. Chem. B, 2008, 112, 756–759 CrossRef CAS PubMed.
- B. Shanmugavelu, V. V. R. K. Kumar, R. Kuladeep and D. N. Rao, Third order nonlinear optical properties of bismuth zinc borate glasses, J. Appl. Phys., 2013, 114, 243103 CrossRef.
- M. Molli, S. Parola, L. A. A. Chunduri, S. Aditha, V. S. Muthukumar, T. M. Rattan and V. Kamisetti, Solvothermal synthesis and study of nonlinear optical properties of nanocrystalline thallium doped bismuth telluride, J. Solid State Chem., 2012, 189, 85–89 CrossRef CAS.
- R. Philip, P. Chantharasupawong, H. Qian, R. Jin and J. Thomas, Evolution of nonlinear optical properties: From gold atomic clusters to plasmonic nanocrystals, Nano Lett., 2012, 12, 4661–4667 CrossRef CAS PubMed.
- J. Wang, Y. Hernandez, M. Lotya, J. N. Coleman and W. J. Blau, Broadband nonlinear optical response of graphene dispersions, Adv. Mater., 2009, 21, 2430–2435 CrossRef CAS.
- S. Kumar, E. S. Shibu, T. Pradeep and A. K. Sood, Ultrafast photoinduced enhancement of nonlinear optical response in 15-atom gold clusters on indium tin oxide conducting film, Opt. Express, 2013, 21, 8483–8492 CrossRef CAS PubMed.
Footnote |
† Electronic supplementary information (ESI) available. See DOI: 10.1039/c6ra28346b |
|
This journal is © The Royal Society of Chemistry 2017 |
Click here to see how this site uses Cookies. View our privacy policy here.