DOI:
10.1039/C6RA28335G
(Paper)
RSC Adv., 2017,
7, 11680-11683
Energetics competition in centrally four-coordinated water clusters and Raman spectroscopic signature for hydrogen bonding†
Received
17th December 2016
, Accepted 5th February 2017
First published on 15th February 2017
Abstract
Extending the electronic structure of four-coordinated hydrogen bonds (H-bonds) to medium sized water cages (H2O)n (n = 17, 19, 20, 21, 23 and 25), we separate the H-bonded neighbour molecules of their centrally four-coordinated water (C4CW) molecules from other molecules in outer cages and discover these two regions interact competitively with the central molecule, showing complementary interaction energy curves with respect to size changes. Raman spectral analyses clearly reveal the characteristic vibration response of water molecules to different H-bonding environment, where the C4CW structure is relatively sensitive. Our theoretical research advances a new perspective for the study of H-bonding interaction in liquid water.
Introduction
The hydrogen bond (H-bond) plays a significant role in physics, chemistry, biology and environmental sciences.1–5 In general, each water molecule within the static liquid water model is bonded to its four nearest neighbor water molecules via two donor and two acceptor bonds, to form the centrally four-coordinated water (C4CW) structure. Two kinds of H-bonds, strong and weak, exist in liquid water,6,7 which is a key factor for determining the properties of water.8 The exchange of strong and weak H-bonds enhances the competition between the open and collapsed structures.7 For these reasons, a great number of detailed spectroscopic and energetic studies have been carried out for C4CW structures.9–12 Theoretical studies9,13 have revealed the interactive strength of the C4CW molecule under the ambient conditions. In particular, some recent experimental and theoretical reports have revealed the covalent-like properties of the H-bond,8,14–16 which further implies the interaction complexity in water clusters through the brought electronic structure anisotropy. In fact, notable differences may exists when comparing the interaction between the C4CW molecule and its nearest neighbor four water (NN4W) molecules with that between the C4CW molecule and surface molecules of water cages (hereafter referred to as rest water molecules). Therefore, the aim of this work is to demonstrate the energetics relation between the C4CW molecule and other water molecules in medium-sized clusters, so that to help connecting the fundamental four-coordinated hydrogen bond model with the behavior of liquid water under ambient condition.
Water clusters are elementary functional units of liquid water meanwhile are used as ideal theoretical models for investigating the properties of H-bonds. Various reports have already indicated that the most stable structures of water clusters (H2O)n (n = 3–5) are cyclic structures17–22 and a transition from two-dimensional to three-dimensional structures appears at n = 6.23–25 For n > 6, the minimum energy structures are three-dimensional, with all molecules constructing the surface of the cluster.22,26,27 For n ≥ 17, stable structures with odd-numbered molecules are C4CW clusters9,28,29 and the recent experimental observations show that the C4CW cluster is also stable for n = 20.30 Consequently, C4CW clusters provide a suitable platform for investigating the medium sized water clusters which is capable to capture the nature of liquid water through providing H-bonds in obviously relatively complex coordination environments.
It is important to understand the nature of liquid water. The covalent-like characteristics of H-bonds between water molecules prompt us to explore the interaction mechanism in water clusters from the perspective of an electronic-structure based division of their geometrical structures. Here, we report a theoretical study of C4CW clusters (H2O)n (n = 17, 19, 20, 21, 23 and 25), based on the hierarchical models, which divide every C4CW cluster into three parts: a C4CW molecule, NN4W molecules and rest water molecules. Apparently, the same level of calculation is helpful in obtaining accurate results for the three parts. So geometry optimizations employed the empirical dispersion-corrected density functional theory (DFT-D3) method with hybrid generalized gradient-approximation (hybrid GGA) at PBE0-D3/6-31+G(d, p) level.31–34 To confirm the obtained structures are truly energy minimum, vibrational frequency verifications have also been carried out at the same level of theory. Furthermore, isotopic substitution was calculated to explore Raman spectra in water clusters. The calculations are performed in the Gaussian 09 program.35
Results and discussion
First of all, we show the stable structures of C4CW clusters in Fig. 1. Moreover, the Cartesian coordinates for all optimized structures provided are in part 1 of the (ESI†). It can be seen that the C4CW molecule for each structure is bonded to NN4W molecules via two donor and two acceptor bonds. To verify the reliability of the corresponding region-divided models, we analyzed the electron density difference taking the C4CW molecule and the whole outer water cage as two interacting monomers. The electron density difference was calculated using the following formula, ρ = ρtotal − ρC4CW, where ρtotal is the electron density of the whole water cluster and ρC4CW is the electron density of C4CW molecule. The results show that electron accumulation and depletion mainly appears between the C4CW molecule and NN4W molecules, without exception. This indicates the NN4W molecules dominate the electronic structure response in the interaction between the center molecule and the outer cage. Dividing NN4W molecules form the rest part of the cage could be reasonable and may provide insights for understanding the total interaction.
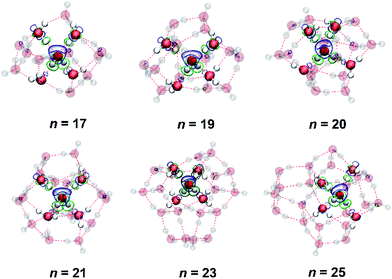 |
| Fig. 1 Electron density difference of C4CW-centered (H2O)n clusters (n = 17, 19, 20, 21, 23 and 25). Oxygen atoms appear in red, hydrogen in white and the broken red lines denote the O:H nonbonds. The C4CW molecule with its NN4W molecules are drawn in solid atoms while the rest molecules in outer cage are transparent. The blue and green regions represent the electron accumulation and depletion, respectively. | |
The interaction between the C4CW molecule and its outer water cage could be different due to the adding of water molecules in the cage. Hence, the interaction energies between the C4CW molecule and the overall water cage (Eint), between the C4CW molecule and its NN4W molecules (Eint(C-4)) and between the C4CW molecule and the rest water molecules (Eint(C-rest)) were respectively calculated and compared among different clusters (see Fig. 2, the details are provided in part 2 of the ESI†). The results have been corrected for basis set superposition error through the counterpoise correction. Fig. 2a shows that the interaction represented by Eint(C-4) is always stronger than that by Eint(C-rest) for different cluster size n, and a stronger Eint(C-4) should correspond to a weaker Eint(C-rest), indicating two parts of the water cage always interacts competitively with the C4CW molecule. Furthermore, another common rule is noteworthy that, two curves of Eint and Eint(C-4) show the same trend thus Eint is dominated by the Eint(C-4), which is in consistent with the previous implication of electronic structure response results in Fig. 1. In addition, we also calculated the average interaction energy per water molecule (E2, black line in Fig. 2b) and the total number of H-bonds for these C4CW clusters (blue line in Fig. 2b). The results show that with the increasing n, the absolute value of E2 and the total number of H-bonds generally increase, while the E2 deviation of this trend for n = 20 and 23 may be attributed to the complexity of geometric structures.
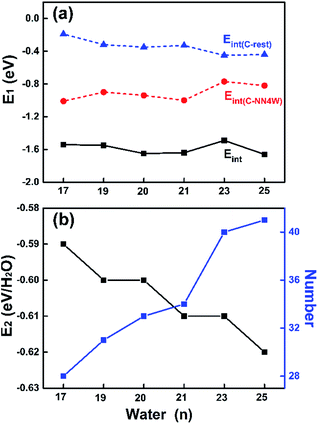 |
| Fig. 2 (a) Total interaction energy of the C4CW molecule with its NN4W molecules (Eint(C-4), in red), the rest molecules (Eint(C-rest), in blue) and the overall water cage (Eint, in black). (b) Average interaction energy per water molecule (E2, in black) of the C4CW cluster and the total number of H-bonds (in blue). In detail, Eint = Etotal − EC − Ecage, Eint(C-NN4W) = EC-NN4W − EC − ENN4W and Eint(C-rest) = EC-rest − EC − Erest, where Etotal, EC-NN4W and EC-rest is respectively the total energy of all molecules, the C4CW molecule with its NN4W molecules and the C4CW molecule with the rest outer cage molecules. EC, ENN4W and Ecage is the total energy of the C4CW molecule, the NN4W molecules and all molecules in the outer cage, respectively. | |
To further illustrate the interaction behavior in medium-sized water clusters and to possibly facilitate experimental structure recognitions through spectroscopic means, we calculated and analyzed Raman spectra for these six clusters. The characteristic range for stretching vibrations of O–H covalent bonds are chosen. This region reported as a signature is the focused on previous studies.36,37 These O–H bonds are classified into different groups according to their intermolecular H-bonding environments. Correspondingly, 4 distinguish parts appear from the total spectra in Fig. 3 (the mass-weighted atomic displacements of these O–H stretching modes for n = 17). The part A is from those H-bonded ones whose oxygen atoms possess the other H atom dangling outside the cluster, which can be found around some vertexes of the cluster. The part B is from those belonging to the C4CW molecule and have involved in its H-bonding four-coordination. The part C is from those in undercoordinated water molecules without dangling O–H groups, which can be found around some concave regions of the cluster. The sharp part D is from those dangling ones. At last, there are still some residual peaks in the total spectra curve in Fig. 3. Shown in this implicit way, these are the fifth contribution from O–H covalent bonds in NN4W molecules, given NN4W molecules satisfy all exception conditions above as no dangling groups, not at the center and not undercoordinated. In addition, isotopic substitution is employed to investigate Raman spectra. This technique has been widely used in spectroscopy studies.38,39 We studied two isotopic mixtures, one is deuterated rest of the water molecules apart (see Fig. S1†), the other is deuterated C4CW molecule (see Fig. S2†). A comparison of the deuterated vs. undeuterated systems showed practically the same characteristic vibration response in the Raman spectra. These results provide further support to the hierarchical models.
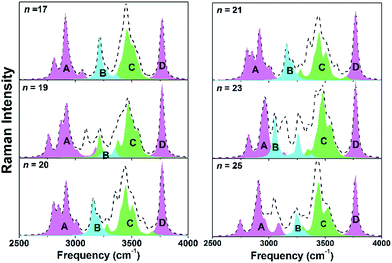 |
| Fig. 3 Raman spectra for O–H bond stretching vibrations in C4CW water clusters (H2O)n (n = 17, 19, 20, 21, 23 and 25) (scaled by 0.9547). The black dashed lines show features from all bonds in entire clusters. Part A is from those H-bonded ones whose oxygen atoms possess the other H atom dangling outside the cluster. Part B is from bonds belonging to the C4CW molecule. Part C is from those in the undercoordinated water molecules without dangling O–H groups and the sharp part D corresponds to the dangling ones. | |
It is shown that, O–H stretching Raman differences among six water clusters are most clearly reflected by the shift and deformation of the C4CW part B and NN4W residual peaks. Comparisons between n = 19 and 20 as well as between n = 21 and 23 provide two most typical cases for this phenomenon. It implies the vibration response of C4CW structures might be able to characterize the size revolution of medium-sized water clusters. Besides, the synergy between the spectral response of part B and NN4W signals also indicates the C4CW molecule interacts with the NN4W molecules relatively strong so that two regions could influence each other through their H-bonding. This is in consistent with above energetic and electronic structure analyses and further supports our region-divided model. On the other hand, part A, C and D are less sensitive to the size changes. Part D almost show no notable response since these O–H dangling bonds are hardly influenced by the surrounding intermolecular environment. Similar stiffen effects for such stiffer frequencies have also been observed in other water systems and are attributed to the undercooridnation.40,41 Reasonably, a common feature of parts A, C and D is corresponding O–H bonds are all in undercoordinated water molecules.
Conclusions
In conclusion, this study gives an access to the quantitative analysis on interaction nature of C4CW clusters, from the perspective of electronic-structure based region-divided models. The energetics results demonstrate the NN4W molecules and the rest outer-cage water molecules interact competitively with the C4CW molecule in medium-sized water clusters. O–H bond Raman spectra present distinguish features with the respect to different H-bonding environments and indicate the O–H bonds of the C4CW water molecules and their NN4W molecules have size-sensitive vibrational frequencies. Besides, further study on the dynamic simulation42 and methodology43 of water clusters are also the direction of our efforts. Hence, we hope that our findings could promote the understanding of the interaction nature towards the liquid water and help to predict the complex behavior of this most ubiquitous substance.
Acknowledgements
The work was supported by the National Natural Science Foundation of China (11374004 and 11674123). Z. W. also acknowledges the Fok Ying Tung Education Foundation (142001) and High Performance Computing Center of Jilin University.
Notes and references
- G. C. Pimentel and A. L. McClellan, The Hydrogen Bond, W. H. Freeman, San Francisco, 1960 Search PubMed.
- S. Scheiner, Hydrogen Bonding: A Theoretical Perspective, Oxford University Press, New York, 1997 Search PubMed.
- G. A. Jeffrey and W. Saenger, Hydrogen Bonding in Biological Structures, Springer-Verlag, Berlin, 1994 Search PubMed.
- G. Gilli and P. Gilli, The Nature of the Hydrogen Bond: Outline of a Comprehensive Hydrogen Bond Theory, Oxford University Press, New York, 2009 Search PubMed.
- C. Q. Sun and Y. Sun, The Attribute of Water, Springer, Singapore, 2016 Search PubMed.
- J. C. Li and A. I. Kolesnikov, J. Mol. Liq., 2002, 100, 1 CrossRef CAS.
- Y. Tu and H. Fang, Phys. Rev. E: Stat., Nonlinear, Soft Matter Phys., 2009, 79, 016707 CrossRef PubMed.
- H. Elgabarty, R. Z. Khaliullin and T. D. Kuhne, Nat. Commun., 2015, 6, 8318 CrossRef CAS PubMed.
- J. P. Furtado, A. P. Rahalkar, S. Shanker, P. Bandyopadhyay and S. R. Gadre, J. Phys. Chem. Lett., 2012, 3, 2253 CrossRef CAS PubMed.
- T. D. Kuhne and R. Z. Khaliullin, Nat. Commun., 2013, 4, 1450 CrossRef PubMed.
- T. Hamashima, K. Mizus and A. Fujii, J. Phys. Chem. A, 2011, 115, 620 CrossRef CAS PubMed.
- A. Hermann, W. G. Schmidt and P. Schwerdtfeger, Phys. Rev. Lett., 2008, 100, 207403 CrossRef CAS PubMed.
- F. F. Wang, G. Jenness, W. A. Al-Saidi and K. D. Jordan, J. Chem. Phys., 2010, 132, 134303 CrossRef PubMed.
- J. Zhang, P. Chen, B. Yuan, W. Ji, Z. Cheng and X. Qiu, Science, 2013, 342, 611 CrossRef CAS PubMed.
- B. Wang, W. Jiang, Y. Gao, B. K. Teo and Z. Wang, Nano Res., 2016, 9, 2782 CrossRef CAS.
- B. Wang, W. Jiang, X. Dai, Y. Gao, Z. Wang and R. Q. Zhang, Sci. Rep., 2016, 6, 22099 CrossRef CAS PubMed.
- M. B. Day, K. N. Kirschner and G. C. Shields, J. Phys. Chem. A, 2005, 109, 6773 CrossRef CAS PubMed.
- K. Kiu, M. G. Brown, J. D. Cruzan and R. J. Saykally, Science, 1996, 271, 62 Search PubMed.
- N. Pugliano and R. J. Saykally, Science, 1992, 257, 1937 CAS.
- J. C. Howard and G. S. Tschumper, J. Chem. Theory Comput., 2015, 11, 2126 CrossRef CAS PubMed.
- R. J. Saykally and D. J. Wales, Science, 2012, 336, 814 CrossRef CAS PubMed.
- P. Qian, W. Song, L. Lu and Z. Yang, Int. J. Quantum Chem., 2010, 110, 1923 CAS.
- M. Losada and S. Leutwyler, J. Chem. Phys., 2002, 117, 2003 CrossRef CAS.
- Y. Wang, V. Babin, J. M. Bowman and F. Paesani, J. Am. Chem. Soc., 2012, 134, 11116 CrossRef CAS PubMed.
- J. J. I. Foley and D. A. Mazziotti, J. Phys. Chem. A, 2013, 117, 6712 CrossRef CAS PubMed.
- H. M. Lee, H. M. S. B. Suh, J. Y. Lee, P. Tarakeshwar and K. S. Kim, J. Chem. Phys., 2000, 112, 9759 CrossRef CAS.
- S. Bulusu, S. Yoo, E. Apra, S. Xantheas and X. C. Zeng, J. Phys. Chem. A, 2006, 110, 11781 CrossRef CAS PubMed.
- S. Yoo, E. Apra, X. C. Zeng and S. S. Xantheas, J. Phys. Chem. Lett., 2010, 1, 3122 CrossRef CAS.
- J. Cui, H. Liu and K. D. Jordan, J. Phys. Chem. B, 2006, 110, 18872 CrossRef CAS PubMed.
- C. C. Pradzynski, C. W. Dierking, F. Zurheide, R. M. Forck, U. Buck, T. Zeuch and S. S. Xantheas, Phys. Chem. Chem. Phys., 2014, 16, 26691 RSC.
- S. Grimme, J. Antony, S. Ehrlich and H. Krieg, J. Chem. Phys., 2010, 132, 154104 CrossRef PubMed.
- J. P. Perdew, K. Burke and M. Ernzerhof, Phys. Rev. Lett., 1996, 77, 3865 CrossRef CAS PubMed.
- C. Adamo and V. Barone, J. Chem. Phys., 1999, 110, 6158 CrossRef CAS.
- J. P. Perdew, K. Burke and M. Ernzerhof, Phys. Rev. Lett., 1997, 78, 1396 CrossRef CAS.
- M. J. Frisch, G. W. Trucks, H. B. Schlegel, G. E. Scuseria, M. A. Robb, J. R. Cheeseman, et al., Gaussian 09, Revision D.01, Gaussian, Inc., Wallingford, CT, 2009 Search PubMed.
- Q. Du, E. Freysz and Y. R. Shen, Science, 1994, 264, 826 CAS.
- C. S. Tian and Y. R. Shen, Proc. Natl. Acad. Sci. U. S. A., 2009, 106, 15148 CrossRef CAS PubMed.
- C. S. Tian and Y. R. Shen, J. Am. Chem. Soc., 2009, 131, 2790 CrossRef CAS PubMed.
- M. Shao, J. Keum, J. Chen, Y. He, W. Chen, J. F. Browning, J. Jakowski, B. G. Sumpter, I. N. Ivanov, Y. Z. Ma, C. M. Rouleau, S. C. Smith, D. B. Geohegan, K. Hong and K. Xiao, Nat. Commun., 2014, 5, 3180 Search PubMed.
- U. Buck and F. Huisken, Chem. Rev., 2000, 100, 3863 CrossRef CAS PubMed.
- K. E. Otto, Z. Xue, P. Zielke and M. A. Suhm, Phys. Chem. Chem. Phys., 2014, 16, 9849 RSC.
- V. Ravi Kumar, C. Verma and S. Umapathy, J. Chem. Phys., 2016, 144, 064302 CrossRef PubMed.
- M. J. Gillan, D. Alfè and A. Michaelides, J. Chem. Phys., 2016, 144, 130901 CrossRef PubMed.
Footnotes |
† Electronic supplementary information (ESI) available. See DOI: 10.1039/c6ra28335g |
‡ These authors contributed equally to this work. |
|
This journal is © The Royal Society of Chemistry 2017 |