DOI:
10.1039/C6RA28205A
(Paper)
RSC Adv., 2017,
7, 15236-15245
Identification of drug transporters involved in the uptake and efflux of rhein in hepatocytes
Received
14th December 2016
, Accepted 27th February 2017
First published on 7th March 2017
Abstract
Aim: Rhein is a herbal medicine with various bioactivities and is derived from an anthraquinone compound. In this study, we aimed to identify drug transporters involved in the uptake and efflux of rhein in hepatocytes. Methods: Rhein uptake and efflux were investigated in rat liver slices, primary rat hepatocytes, human transporter-transfected cells, membrane vesicles, and rats. Rhein was quantified using liquid chromatography-tandem mass spectrometry. Results: Rhein uptake was fitted to a Michaelis–Menten model with a Km = 229.4 ± 58.5 μM and Vmax = 428.7 ± 49.2 pmol mg−1 protein in liver slices and a Km = 129.6 ± 22.3 μM and Vmax = 9.1 ± 0.6 nmol mg−1 protein in primary rat hepatocytes. Rhein uptake by primary rat hepatocytes was inhibited by organic anion-transporting polypeptide (OATP) inhibitors (rifampicin and fluvastatin) and an organic anion transporter (OAT) inhibitor (probenecid). Rhein was taken up by HEK293 cells expressing OATP1B3, OATP2B1, and OAT2 and pumped out of MDCKII cells expressing breast cancer resistance protein (BCRP), but not from those expressing P-glycoprotein (P-gp) or from membrane vesicles expressing multidrug resistance-associated proteins (MRPs) 2 and 3. In rats, hepatic exposure to rhein was reduced by rifampicin and probenecid (AUC0–2 h decreased 0.61- and 0.12-fold, respectively), but increased by pantoprazole (AUC0–2 h increased 0.64-fold), a BCRP inhibitor. Conclusion: These results show that OATP1B3, OATP2B1, and OAT2 contribute to hepatic rhein uptake, while BCRP contributes to its hepatic efflux. The results provide new insights into the mechanisms of rhein uptake and efflux in hepatocytes.
Introduction
Rhei Radix et Rhizoma (RR), a herbal medicine prepared from the Polygonaceae plant family, is widely used to treat diseases including liver diseases,1,2 constipation,3 pancreatitis, cholecystitis, chronic renal failure,4,5 and upper gastrointestinal hemorrhage.6,7 Rhein (4,5-dihydroxy-9,10-dioxoanthracene-2-carboxylic acid; Fig. 1) is a major anthraquinone compound in RR and the most abundant anthraquinone detected in the blood and liver of animals orally administered RR extract.8,9 Rhein has a wide spectrum of bioactivities and is a candidate drug for the treatment of diabetic nephropathy.10 It is also the active metabolite of diacerein, a modern medicine used as a prodrug for the treatment of osteoarthritis.11
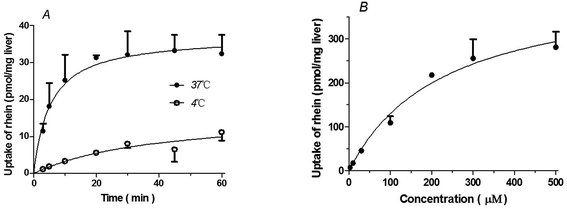 |
| Fig. 1 Time, temperature (A), and concentration-dependence (B) profiles of rhein uptake in liver slices. (A) The concentration of rhein used in these experiments was 10 μM. (B) The uptake of rhein in liver slices was measured at a concentration between 3 and 500 μM over 5 minutes at 37 °C. Data are presented as means ± S.D. (n = 3). | |
The liver is a target organ of rhein and the most important determinant organ of its pharmacokinetics; therefore, it is important to elucidate the mechanisms underlying the hepatic disposition of rhein. Reportedly, rhein is metabolized to rhein sulfate by sulfotransferase and to rhein glucuronide by UDP-glucuronosyltransferase.11 However, the mechanisms of rhein uptake and efflux in hepatocytes remain to be elucidated. In general, the bidirectional transport of compounds across hepatocyte membranes involves multiple transporters. Uptake of compounds from the portal bloodstream into hepatocytes involves transporters expressed on the basolateral membrane, including organic anion-transporting polypeptides (OATPs)12,13 and organic anion transporters (OATs),12,14 which are thought to exert a significant influence on the removal of various endogenous and xenobiotic substances from the bloodstream into hepatocytes.15 The efflux of compounds from hepatocytes involves transporters expressed on the canalicular membrane and blood sinus, including multidrug resistance-associated proteins (MRPs),16 breast cancer resistance protein (BCRP),17 and P-glycoprotein (P-gp).18,19 It has been reported that rhein is both an inhibitor and a substrate of OAT1/3;20,21 however, OAT1 and OAT3 are primarily expressed in the kidney and are rarely expressed in the liver. Further, rhein is reportedly an MRP1 substrate in colonic epithelial cells,22 which are expressed to a greater extent in the kidneys than in the liver. In addition, BCRP is involved in the intestinal transport of rhein;23 however, this has not been shown in hepatocytes. Therefore, little is known regarding the transporters involved in the hepatic uptake and efflux of rhein.
In this study, we aimed to investigate the mechanisms of rhein uptake and efflux in hepatocytes. Rat liver slices and primary rat hepatocytes were used as preliminary models to investigate the transporters involved in rhein hepatic transport. Next, in vitro studies using transporter-transfected cells and membrane vesicles were conducted to specify the transporters involved in rhein uptake and efflux in the liver. Finally, we determined the effects of specific transporter inhibitors on hepatic rhein concentrations in rats.
Materials and methods
Reagents
Estradiol-17β-glucuronide, glycyrrhizic acid, pantoprazole, and probenecid were purchased from the National Institute for the Control of Pharmaceutical and Biological Products (Beijing, China). Rhein (purity > 98%) was purchased from YuanYe Biological Technology Co. (Shanghai, China). Verapamil, rifampicin, fluvastatin, saquinavir, mycophenolic acid (internal standard, IS), and Ko143 were obtained from Sigma-Aldrich (St. Louis, MO, USA). Dulbecco's modified Eagle's medium (DMEM), MEM, nonessential amino acids, L-glutamine, penicillin, streptomycin, and fetal bovine serum were purchased from GIBCO (Invitrogen Corporation, Grand Island, NY). Biocoat™ Matrigel™ was purchased from BD Biosciences (Bedford, MA). Acetonitrile was the product of Merck (Darmstadt, Germany). Water was purified by a MilliQ water system (Millipore, Bedford, MA). Chromatogram-grade formic acid was purchased from Tedia Company Inc. (Denver, CO, USA). The bicinchoninic acid (BCA) protein assay kit was purchased from Beyotime Institute of Biotechnology (Shanghai, China). All other reagents were of analytical grade or better.
Animals
Male Sprague-Dawley rats (220–250 g body weight) were obtained from the Experimental Animal Center of Shanghai University of Traditional Chinese Medicine (Shanghai, China; Certificate No. SYXK 2014-0008) and housed in an air-conditioned animal room (22–24 °C) under a 12 h light/dark cycle for 1 week prior to use. Rats were allowed free access to water and chow diet; however, they were fasted for 12 h (with water ad libitum) before the experiments. All animal experimental protocols were approved by the Institutional Animal Care and Use Committee of Shanghai University of Traditional Chinese Medicine (Approval Number: 2015007), and all experiments were performed per the guidelines of this committee.
Cell culture
Human embryonic kidney (HEK293) cells stably expressing OAT2 (HEK293-OAT2) and mock-transfected cells (HEK293) obtained using the virus vector method were purchased from Shanghai GeneChem Co., Ltd. (Shanghai, China). Expression was confirmed by western blot analysis. HEK293-OATP1B1, HEK293-OATP2B1, and HEK293-OATP1B3 cell lines were obtained from Dr Xiaoyan Chen, Shanghai Institute of Materia Medica, Chinese Academy of Sciences (Shanghai, China). Madin–Darby canine kidney (MDCKII) cells and MDCKII-BCRP cell lines were obtained from Professor Xiaodong Liu, Center of Drug Metabolism and Pharmacokinetics, China Pharmaceutical University (Nanjing, China). The MDCKII-P-gp cell line was obtained from Professor Min Huang, School of Pharmaceutical Science, Sun Yat-sen University (Guangzhou, China). All cell lines were cultured at 37 °C with a 5% CO2 atmosphere and 95% relative humidity in high-glucose DMEM supplemented with 10% fetal bovine serum (Invitrogen), 1% penicillin–streptomycin, 2 mM L-glutamine, and 1% MEM nonessential amino acids. Cells were split at a 1
:
3 (HEK293 cells) or 1
:
6 (MDCKII cells) ratio every 3 or 4 days.19,24
Liquid chromatography-tandem mass spectrometry (LC-MS/MS) analysis
The concentration of rhein in various samples was measured using a validated LC-MS/MS method. Briefly, a triple-quadrupole mass spectrometer (Thermo Scientific TSQ Quantum Ultra; San Jose, CA, USA) equipped with an electrospray ionization (ESI) probe and a Shimadzu Prominence UFLCXR system (Shimadzu, Japan) was used. The samples were precipitated with acetonitrile. After centrifugation (17
000 rpm, 10 min, 4 °C), the supernatants were injected into the LC-MS/MS system. LC separations were achieved using a Hypersil Gold C18 analytical column (100 × 2.1 mm, 5 μm) with a guard column (10 mm × 2.1 mm, 5 μm). The mobile phase was composed of 0.1% v/v formic acid in water (A) and acetonitrile (B) at gradients of 10% B → 80% B (0 → 8 min), 80% B (8.0 min → 8.1 min), and 10% B → 10% B (8.1 min → 10.0 min) at a flow rate of 0.3 mL min−1. The ESI source was set to negative ion mode. Data acquisition was performed using multiple-reaction monitoring of the transitions from m/z 283.0 → 239.0 and 319.0 → 191.0 for rhein and mycophenolic acid (internal standard), respectively.
Uptake of rhein by rat liver slices
Rat liver slices were prepared as described previously.25 In brief, rats were euthanized with 25% urethane. The livers were sampled and then placed in ice-cold incubation buffer saturated with carbogen (95% O2/5% CO2). The livers were then sliced at a thickness of 200–300 μm using a ZQP-86 tissue slicer (Zhixin Co., Ltd., Shanghai, China). Liver slices were selected randomly and pre-incubated in a 24-well culture plate with 0.5 mL incubation buffer in each well under a carbogen atmosphere at 4 °C or 37 °C for 3 min. Absorption conditions, including the concentration of rhein and the incubation time, were optimized to ensure the linearity of rhein absorption and minimal rhein metabolism. After this pre-incubation, the buffer was replaced with an incubation buffer containing various concentrations of rhein. Each slice was then rapidly removed at the designated time point from the incubation buffer, washed and mechanically homogenized essentially as described previously,26 but with modifications. In brief, each liver slice was transferred to a centrifuge tube with a steel ball, a volume of water three times the weight of the slice was added, and the tube was placed in the tissue homogenizer (TissueLyserII® from QIAGEN). After homogenization for 8 min at a frequency setting of 25 s−1, methanol was added and the contents were mixed by vortexing. The tube was then centrifuged at 4 °C with 16
000 rpm min−1 for 10 min and the supernatant was used to determine the concentration of rhein in liver using the LC-MS/MS method described above.
Uptake of rhein by primary rat hepatocytes
Primary hepatocytes were isolated from rats using a previously described two-step collagenase digestion method.27,28 Hepatocyte viability was determined to be greater than 85% by trypan blue exclusion. Hepatocytes were plated at a density of 2 × 105 cells per well in 24-well Biocoat™ plates (BD Biosciences Discovery Labware, Bedford, MA) and allowed to attach for 3–4 h at 37 °C in a humidified chamber with a 95% air/5% CO2 atmosphere. After attachment, the medium was aspirated to remove non-adherent cells, replaced with fresh Hank's balanced salt solution (HBSS), and incubated for 10 min at 37 °C before conducting rhein uptake studies. Absorption conditions, including the concentration of rhein and the incubation time, were optimized to ensure linearity of rhein absorption and minimal rhein metabolism. The effects of transporter inhibitors, including rifampicin, glycyrrhizic acid, fluvastatin, saquinavir, and probenecid, on rhein uptake were investigated in the presence of 10 μM rhein for 1 min. After removal of the incubation buffer, the reaction was terminated by adding ice-cold buffer. Cells were then washed and homogenized by ultra-sonication. The homogenate was extracted using a single-step protein precipitation procedure. Protein concentrations were determined using a BCA protein assay kit, and rhein concentrations were measured using the LC-MS/MS method described above.
Uptake of rhein in transporter-transfected cells
Uptake of rhein by OATP1B3, OATP2B1, OATP1B1, and OAT2 was evaluated using transporter-transfected cell lines as described previously.29 Briefly, HEK293 cells stably transfected with one of these transporters or an empty vector were seeded at a density of 3.0 × 105 cells per well on 24-well plates coated with BioCoat poly-D-lysine (BD Biosciences). After 36 h, the cells were incubated with HBSS at 37 °C for 10 min. Uptake was then initiated by adding 0.2 mL HBSS containing 10 μM rhein. Uptake was terminated 1 min later by aspirating the medium and washing the cells twice with ice-cold HBSS. Cells were lysed by freeze–thaw cycles between −80 °C and 37 °C. The homogenates were extracted using a single-step protein precipitation procedure. Protein concentrations were determined using a BCA protein assay kit, and rhein concentrations were measured using the LC-MS/MS method described above.
Transcellular transport of rhein in transporter-transfected cells
Transcellular transport studies were conducted as described previously.30 Briefly, MDCKII cells stably transfected with either P-gp or BCRP were seeded at a density of 1.4 × 105 cells per well in 24-well transwell inserts (12 mm diameter, 0.6 cm2 growing surface area, 0.4 μm pore size) obtained from Corning Costar (Acton, MA) or Millipore (Billerica MA). After 3–5 days, MDCKII cells (>200 Ω cm2 for Costar and > 1000 Ω cm2 for Millipore) were incubated with or without inhibitors for 60 min. Ko143 (a BCRP inhibitor, 5 μM) or verapamil (a P-gp inhibitor, 100 μM) were added to both the apical and basolateral sides. Subsequently, rhein (10 μM), cimetidine (5 μM, a BCRP substrate), or rhodamine 123 (Rho123, 10 μM, a P-gp substrate) were loaded on the apical (400 μL) or basolateral (600 μL) side of the transfected MDCKII cell monolayer. Samples (50 μL) were collected from both sides of each transwell at 1, 2, 3, and 4 h and replaced with the same volume of transport buffer. The concentration of rhein, cimetidine,31 and Rho123 (ref. 32) in these samples was determined using the LC-MS/MS method described above.
Efflux of rhein in membrane vesicles
Experiments were conducted using a modified rapid filtration technique based on the manufacturer's protocol (BD Biosciences). In brief, MRP2 or MRP3 membrane vesicles prepared from insect cells (Sf9) overexpressing MRP2 or MRP3 (50 μg protein) were incubated with the test compounds (rhein and a positive control substrate, estradiol-17β-glucuronide) and 2 mM L-glutathione in the presence of 25 mM ATP or 25 mM AMP in a total volume of 60 μL (pH 7.4). Transport was terminated at the designated time-point by the addition of 200 μL ice-cold wash buffer. The incubation mix was rapidly transferred to a Millipore 96-well glass fiber filter plate (Millipore Corporation, Billerica, MA) and then washed five times with ice-cold wash buffer. The compound trapped in the membrane vesicles was retained on the filters and released by the addition of 200 μL methanol. The concentration of compounds in these samples was determined using the LC-MS/MS method described above.
Effect of transporter inhibitors on the concentration of rhein in the liver of rats
Effects of rifampicin and probenecid. Rats were randomly divided into 12 groups and intravenously injected with 40 mg kg−1 rifampicin, 50 mg kg−1 probenecid, or normal saline; after 5 min, all rats were intravenously injected with 1.4 mg kg−1 rhein. The rhein dose was based on pharmacological studies.33 Rats were sacrificed 15, 30, 60, or 120 min after receiving rhein, and the livers were sampled. Liver homogenates were prepared in ice-cold saline, and rhein concentrations were determined using the LC-MS/MS method described above.
Effects of pantoprazole. Rats were randomly divided into 8 groups and intravenously injected with 40 mg kg−1 pantoprazole or normal saline; after 15 min, all rats were intravenously injected with 1.4 mg kg−1 rhein. Rats were sacrificed 15, 30, 60, or 120 min after receiving rhein, and the livers were sampled. Liver homogenates were prepared in ice-cold saline, and the concentration of rhein was determined using the LC-MS/MS method described above.
Data analysis
Rhein concentrations were calculated using a standard curve. Transport kinetic parameters were obtained by fitting the data to the Michaelis–Menten model V = Vmax[S]/(Km + [S]), where V is the uptake velocity of the substrate, [S] is the concentration of the substrate in the medium, Km is the Michaelis–Menten constant, and Vmax is the maximum uptake rate. Km and Vmax were calculated using GraphPad Prism 5.0 (GraphPad Prism Software, San Diego, CA). The pharmacokinetic parameters were calculated using a non-compartmental analysis (WinNonLin Pro 6.1).
Permeability calculations and efflux ratio (ER). The apparent permeability coefficients (Papp) were calculated using eqn (1), where slopes (dQ/dt) were obtained in the initial linear range (up to 240 min) from the plots of amount transported versus time. The efflux ratio (ER) was calculated as the ratio of the Papp for basolateral-to-apical transport to the Papp for apical-to-basolateral transport. |
Papp = (dQ/dt)/(A × C0)
| (1) |
(A is the area of the transwell membrane, and C0 is the initial donor concentration).All data are presented as the means ± standard deviation. The in vitro effects of inhibitors were analyzed by one-way analysis of variance (ANOVA). When the ANOVA identified a statistically significant difference of P < 0.05, Dunnett's t-test was applied. The significance of differences between two groups was analyzed by student's t-test. P < 0.05 was considered statistically significant.
Results
Uptake of rhein by rat liver slices
Rhein uptake by liver slices was time- and temperature-dependent. Rhein uptake at 37 °C was greater than that observed at 4 °C (P < 0.01) (Fig. 1A). Rhein uptake by liver slices showed the best fit to the Michaelis–Menten model with a Km = 229.4 ± 58.49 μM and Vmax = 428.7 ± 49.16 pmol mg−1 protein (Fig. 1B).
Uptake of rhein by primary rat hepatocytes
Uptake of rhein by primary rat hepatocytes was time- and temperature-dependent. Rhein uptake at 37 °C was greater than that observed at 4 °C (P < 0.01) (Fig. 2A). Rhein uptake in rat hepatocytes fit the Michaelis–Menten model with a Km = 129.6 ± 22.31 μM and Vmax = 9.07 ± 0.55 nmol mg−1 protein (Fig. 2B). Furthermore, rhein uptake was inhibited by OATP inhibitors such as rifampicin (P < 0.01), glycyrrhizic acid (P < 0.001), and saquinavir (P < 0.01), as well the OAT inhibitor, probenecid (P < 0.01) and fluvastatin (P < 0.01) (Fig. 2C).
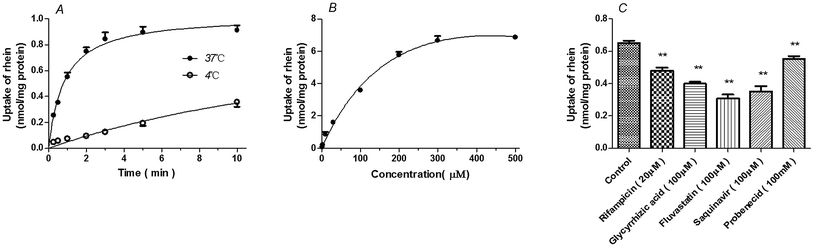 |
| Fig. 2 Time, temperature (A), and concentration-dependence (B) profiles of rhein uptake, as well as the effects of transporter inhibitors on the intracellular concentration of rhein (C) in primary rat hepatocytes. (A) The concentration of rhein used in these experiments was 10 μM. (B) The uptake of rhein in primary rat hepatocytes was measured at a concentration between 1 and 500 μM for 1 min at 37 °C. (C) Cells were incubated with 10 μM rhein for 1 min in the presence of the indicated inhibitor or vehicle (control). **P < 0.01 versus the control group (means ± S.D., n = 3). | |
Uptake of rhein by transporter-transfected cells
Rhein (10 μM) uptake by HEK293 cells expressing OATP1B3 (P < 0.001), OATP2B1 (P < 0.01), and OAT2 (P < 0.01) was higher than that observed in vector-transfected HEK293 cells (Fig. 3). However, there were no significant differences between HEK293 cells expressing OATP1B1 (P > 0.05) and vector-transfected HEK293 cells.
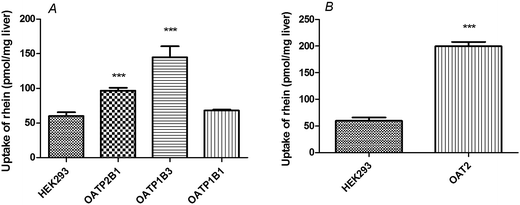 |
| Fig. 3 Rhein uptake in OATP- and OAT2-transfected HEK293 cells. Cells were incubated with 10 μM rhein for 1 min. ***P < 0.001 versus the Mock-HEK293 group (means ± S.D., n = 3). | |
Transcellular transport of rhein in BCRP and P-gp transfected MDCKII cells
The basolateral-to-apical transport of rhein was greater than its apical-to-basolateral transport in BCRP-MDCKII cells (P < 0.01). In the presence of 5 μM Ko143, a BCRP inhibitor, the basolateral-to-apical transport of rhein decreased and the rhein efflux ratio decreased from 5.0 to 2.1 (Fig. 4A, Table 1) (P < 0.01). This effect was also observed in the presence of cimetidine, a BCRP substrate (Fig. 4C). However, there were no significant differences between the basolateral-to-apical and apical-to-basolateral transport of rhein in P-gp-MDCKII cells (P > 0.05). Furthermore, verapamil, a P-gp inhibitor, did not affect rhein transport (Fig. 4B, Table 1) (P > 0.05). These results differ from those obtained using Rho123 (Fig. 4D).
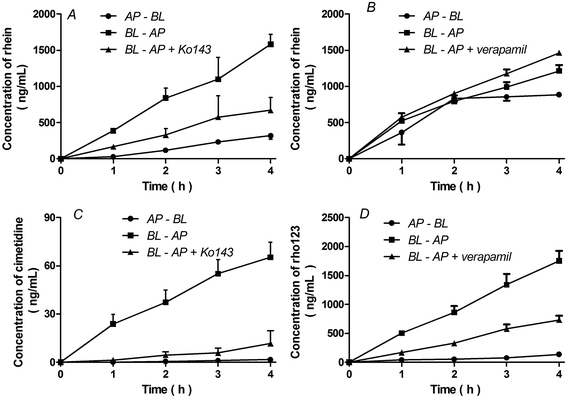 |
| Fig. 4 Transcellular transport of rhein (10 μM) in MDCKII cells expressing BCRP (A) or P-gp (B) and incubated with 5 μM cimetidine (BCRP substrate control), (C), 10 μM Rho123 (P-gp substrate control, (D), 5 μM Ko143 (BCRP inhibitor), or 100 μM verapamil (P-gp inhibitor), as indicated. AP, apical; BL, basolateral. Data are presented as means ± S.D. (n = 3). | |
Table 1 The apparent permeability coefficients (Papp) and efflux ratio (ER) of rhein with or absence of inhibitor in MDCKII cells
Cell lines |
Time (h) |
Rhein |
Rhein + Ko143 for BCRP or verapamil for P-gp |
AP-to-BL Papp |
BL-to-AP Papp |
ER |
BL-to-AP Papp |
ER |
BCRP-MDCKII |
1 |
1.9 × 10−6 |
2.5 × 10−5 |
13.0 |
1.1 × 10−5 |
5.6 |
2 |
3.8 × 10−6 |
2.7 × 10−5 |
7.2 |
1.1 × 10−5 |
2.8 |
3 |
5.0 × 10−6 |
2.4 × 10−5 |
4.7 |
1.3 × 10−5 |
2.5 |
4 |
5.2 × 10−6 |
2.6 × 10−5 |
5.0 |
1.1 × 10−5 |
2.1 |
P-gp-MDCKII |
1 |
1.3 × 10−5 |
2.0 × 10−6 |
1.6 |
1.8 × 10−5 |
1.4 |
2 |
1.4 × 10−5 |
1.6 × 10−5 |
1.1 |
1.4 × 10−5 |
1.0 |
3 |
9.9 × 10−6 |
1.4 × 10−5 |
1.4 |
1.2 × 10−5 |
1.2 |
4 |
7.7 × 10−6 |
1.3 × 10−5 |
1.7 |
1.0 × 10−5 |
1.4 |
Efflux of rhein from membrane vesicles
We found no significant difference between transport in the presence of ATP and that in the presence of AMP in membrane vesicles expressing MRP2 or MRP3 (P > 0.05 for both) (Fig. 5, Table 2). This demonstrated that no significant ATP-dependent rhein transport occurred in either MRP2 or MRP3 vesicles. In contrast, the ratio of ATP-dependent uptake to nonspecific adsorption (ATP/AMP ratio) for estradiol-17β-glucuronide, a known MRP substrate, was 22.4-fold in MRP2-expressing vesicles and 10-fold in MRP3-expressing vesicles, respectively (P < 0.01) (Fig. 5, Table 2).
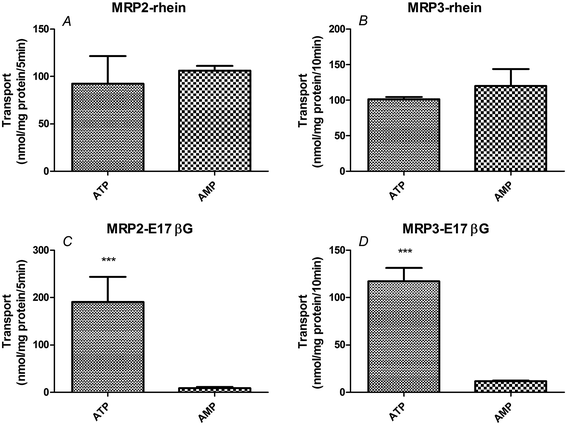 |
| Fig. 5 Transport in membrane vesicles expressing MRP2 or MRP3. 10 μM rhein or 1 μM estradiol-17β-glucuronide (E17βG; MRP substrate) were incubated with membrane vesicles expressing the indicated transporter in the presence of ATP (25 mM)or AMP (25 mM) for 5 min (MRP2) or 10 min (MRP3) (n = 3). ATP group means added 25 mM ATP in assay uptake buffer while AMP group means added same concentration of AMP to the buffer as control. ***P < 0.001 versus the control (AMP) group (means ± S.D., n = 3). | |
Table 2 The membrane vesicles type and the ATP/AMP ratios in each group
Membrane vesicles type |
Group |
Unit |
ATP |
AMP |
ATP/AMP ratio |
MRP2 |
E17βG |
(nmol mg−1 protein/5 min) |
190.6 ± 53.2 |
8.5 ± 2.9 |
22.4 |
Rhein |
92.6 ± 28.9 |
106.2 ± 4.9 |
0.9 |
MRP3 |
E17βG |
(nmol mg−1 protein/10 min) |
117.2 ± 14.2 |
11.7 ± 0.6 |
10.0 |
Rhein |
101.5 ± 2.8 |
120.3 ± 23.4 |
0.8 |
Effect of transporter inhibitors on the concentration of rhein in rat livers
As shown in Fig. 6, rifampicin decreased (P < 0.05, Fig. 6A), but pantoprazole increased (P < 0.05, Fig. 6C) rhein concentrations in the liver. However, probenecid only decreased the concentration of rhein in the liver after 15 min (P < 0.01, Fig. 6B).
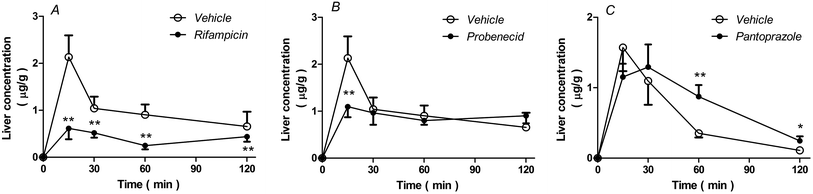 |
| Fig. 6 Concentration of rhein in the liver (A, B, and C) at the indicated time-points. Rats received either intravenous rifampicin (40 mg kg−1), probenecid (50 mg kg−1), or saline separately, followed by rhein (1.4 mg kg−1) after 5 min or pantoprazole (40 mg kg−1) or saline followed by rhein (1.4 mg kg−1) after 15 min. Data represent the means ± S.D., n = 3. **P < 0.01; *P < 0.05 versus vehicle-treated rats. | |
As shown in Table 3, AUC0–2 h values from the liver of rats treated with rifampicin or probenecid were 0.61-fold and 0.12-fold lower than those of vehicle-treated rats, respectively. The AUC0–2 h values from the liver of rats treated with pantoprazole were 0.64-fold higher than those from vehicle-treated rats.
Table 3 Pharmacokinetic parameters of rhein in rat livers pretreated with rifampicin, probenecid and pantoprazole
Parameters |
Uptake inhibitors |
Efflux pump inhibitor |
Vehicle |
Rifampicin |
Fold |
Probenecid |
Fold |
Vehicle |
Pantoprazole |
Fold |
AUC0–2 h (μg h g−1) |
1.929 |
0.753 |
0.39 |
1.689 |
0.88 |
0.867 |
1.426 |
1.64 |
C15 min (μg g−1) |
2.133 |
0.614 |
0.29 |
1.10 |
0.52 |
1.57 |
1.20 |
0.76 |
MRT0–2 h (h) |
0.86 |
1.01 |
1.17 |
1.04 |
1.21 |
0.48 |
0.86 |
1.79 |
Discussion
In the present study, transporter inhibitors were used to study the role of transporters on the concentration of rhein in cultured cells and rat livers. Because inhibitor selectivity is influenced by concentration, we referred to the literature to choose transporter inhibitors and appropriate concentrations. All transporter substrates and inhibitors used are listed in Table 4.
Table 4 Transporters and the corresponding concentration of Substrates or inhibitor in vitro and in vivo
|
Substrates |
Inhibitors |
Concentration |
Transporters |
in vitro |
|
Rifampicin13 |
20 μM |
OATPs |
|
Glycyrrhizic acid13 |
100 μM |
OATP1A2 |
|
Saquinavir42,43 |
100 μM |
OATPs |
|
Probenecid44,45 |
100 μM |
OATs |
|
Ko143 (ref. 46) |
5 μM |
BCRP |
|
Verapamil47 |
100 μM |
P-gp |
|
Fluvastatin48 |
100 μM |
OATs |
Cimetidine46 |
|
5 μM |
BCRP |
Rho123 (ref. 49) |
|
10 μM |
P-gp |
Estradiol-17β-glucuronide50 |
|
1 μM |
MRP2, MRP3 |
in vivo |
|
Rifampicin51 |
40 mg kg−1 |
Oatps |
|
Probenecid52,53 |
50 mg kg−1 |
Oats |
|
Pantoprazole54 |
40 mg kg−1 |
Bcrp |
Drug transporters involved in the uptake of rhein in hepatocytes were first identified. We observed that the uptake of rhein was temperature dependent (37 °C > 4 °C) and concentration dependent (Michaelis–Menten uptake at 37 °C) in rat liver slices and primary hepatocytes, indicating that its uptake was active and involved transporters. We further observed that rhein uptake by rat primary hepatocytes was reduced by OATP and OAT inhibitors (rifampicin, glycyrrhizic acid, saquinavir, fluvastatin and probenecid), suggesting the involvement of these transporters. Furthermore, rhein uptake was identified using HEK293 cells over-expressing OATP1B1, OATP1B3, OATP2B1, and OAT2. These results verify that OATP1B3, OATP2B1, and OAT2 contribute to the hepatic uptake of rhein.
An earlier study reported the efflux of rhein in intestinal transport.34 In that study, BCRP was considered to be involved in rhein transport, based on decreased basolateral-to-apical rhein transport by BCRP inhibition in Caco-2 cells and increased basolateral-to-apical rhein transport in MDCKII-BCRP cells.34 However, there was insufficient evidence of rhein being a BCRP substrate, because the efflux ratio of rhein was not provided in the experiment using MDCKII-BCRP cells,34 in which an efflux ratio >1.5 was indicative of BCRP-mediated active transport.35,36 In the present study, we found that the efflux ratio of rhein was >1.5, which decreased along with basolateral-to-apical rhein transport in the presence of BCRP inhibitors in BCRP-MDCKII cells, which provided a more reliable evidence of rhein efflux mediated by BCRP. In addition, we did not observe significant ATP-dependent rhein transport in either MRP2 or MRP3 vesicles, excluding their participation in rhein efflux and adding to the above report, which only addressed MRP2.34 Our results showing a lack of significant directionality differences in rhein permeability in P-gp-MDCKII cells were similar to those in the above report.34 In summary, our findings definitively indicate that BCRP is involved in rhein efflux in hepatocytes.
Furthermore, the effects of these transporters on the liver concentration of rhein were investigated in vivo using transporter inhibitors in rats. The results show that inhibition of OATPs (by rifampicin) obviously decreases the hepatic concentration of rhein. In contrast, inhibition of OATs by probenecid only slightly decreased the liver concentration of rhein. It is known that probenecid also inhibits UDP-glucuronosyltransferase, and the effects on drug metabolizing enzymes might counteract the decreasing effects of OAT inhibition on the hepatic concentration of rhein by reducing its metabolism. To minimize costs and maintain effectiveness of the inhibitors used, Ko143 was used as a BCRP inhibitor for the in vitro experiments and pantoprazole was used as a BCRP inhibitor for the in vivo experiments. Inhibition of BCRP by pantoprazole markedly increased the concentration of rhein in the liver. In summary, these results suggest that inhibition of OATPs and OATs reduces the liver concentration of rhein, whereas inhibition of BCRP increases the liver concentration of rhein.
Clinical herb–drug interactions due to cytochrome P450 enzymes/drug transporters in the liver have been reported.37,38 Our results indicate that OATP inhibitors such as rifampicin or OAT inhibitors such as probenecid may influence hepatic uptake of rhein, ultimately altering rhein bioactivity. According to earlier reports, cyclosporine A prevented rhein-induced apoptosis induced in hepatocytes.39 Based on our experiments, we speculate that the protective effects of cyclosporine A may be associated with decreased OATP-mediated cellular uptake of rhein because cyclosporine A was shown to inhibit OATPs.40,41
Reportedly, rhein is metabolized to its sulfate (R-S) by sulfotransferase (SULT) and to its glucuronide (R-G) by UDP-glucuronosyltransferase 1A (UGT1A) in the liver.33 Preliminary experiments demonstrated that inhibitors of UGT1A and SULT also influence the concentration of rhein in hepatocytes (data not shown). Given that RR is a frequently used botanical drug in Asian countries and that rhein is currently in phase II clinical trials in China, more studies should be carried out to carefully characterize and evaluate such influences.
In conclusion, the results show that OATP1B3, OATP2B1, and OAT2 contribute to hepatic rhein uptake, whereas BCRP contributes to its hepatic efflux. These results provide new insights into the mechanisms of rhein uptake and efflux in hepatocytes.
Authorship contributions
Yue-Ming MA and Yan DAI designed the research; Yan DAI and Min ZHENG performed the research; Yan DAI and Yuan–Yuan LI contributed to the analytic tools; Yan DAI and Tian-Ming WANG analyzed the data; Yan DAI, Yue-Ming MA and Bing-Liang MA wrote the paper.
Abbreviations
BCRP | Breast cancer resistance protein |
HBSS | Hank's balanced salt solution |
HEK293 | Human embryonic kidney 293 cells |
LC-MS/MS | Liquid chromatography-tandem mass spectrometry |
MDCKII cells | Madin–Darby canine kidney strain II cells |
MRP | Multidrug resistance-associated protein |
m/z | Mass-to-charge ratio |
OAT | Organic anion transporter |
OATP | Organic anion-transporting polypeptide |
P-gp | P-Glycoprotein |
UGT | UDP-glucuronosyltransferase |
R-G | Glucuronide of rhein |
R-S | Sulfate of rhein |
Acknowledgements
We thank Dr Xiaoyan Chen (Shanghai Institute of Materia Medica, Chinese Academy of Sciences, Shanghai, China) for the transfecting HEK293-cell lines. We thank Professor Xiaodong Liu (Center of Drug Metabolism and pharmacokinetic, China Pharmaceutical University, Nanjing, China) for providing human BCRP-MDCKII cells. We also thank Professor Min Huang (School of Pharmaceutical Science, Sun Yat-sen University, Guangzhou, China) for providing human MDR1-MDCKII cells. This work was financially supported by the National Natural Science Foundation of China [No. 81273658]; the Doctoral Program Foundation of Institutions of Higher Education of China [20113107110005]; and “085” First-Class Discipline Construction of Science and Technology Innovation [085ZY1205].
References
- F. Muller, J. Konig, E. Hoier, K. Mandery and M. F. Fromm, Biochem. Pharmacol., 2013, 86, 808–815 CrossRef PubMed.
- M. G. Ramos-Zavala, M. Gonzalez-Ortiz, E. Martinez-Abundis, J. A. Robles-Cervantes, R. Gonzalez-Lopez and N. J. Santiago-Hernandez, Diabetes Care, 2011, 34, 1591–1594 CrossRef CAS PubMed.
- A. K. Aggarwal and S. Singh, PDA J. Pharm. Sci. Technol., 2012, 66, 201–213 CrossRef CAS PubMed.
- Y. H. Tien, B. H. Chen, G. S. Wang Hsu, W. T. Lin, J. H. Huang and Y. F. Lu, Am. J. Chin. Med., 2014, 42, 1385–1398 CrossRef PubMed.
- M. S. Hohmann, R. D. Cardoso, V. Fattori, N. S. Arakawa, J. C. Tomaz, N. P. Lopes, R. Casagrande and W. A. Verri Jr, Phytother. Res., 2015, 29, 1097–1101 CrossRef CAS PubMed.
- D. H. Jiao, Y. H. Ma, S. J. Chen, C. T. Liu, H. N. Shu and C. M. Chu, Pharmacology, 1980, 20(1), 128–130 CrossRef PubMed.
- E. Song, J. Fu, X. Xia, C. Su and Y. Song, PLoS One, 2014, 9, e107405 Search PubMed.
- S. Y. Wei, W. X. Yao, W. Y. Ji, J. Q. Wei and S. Q. Peng, Food Chem., 2013, 141, 1710–1715 CrossRef CAS PubMed.
- F. Fang, J. B. Wang, Y. L. Zhao, C. Jin, W. J. Kong, H. P. Zhao, H. J. Wang and X. H. Xiao, J. Ethnopharmacol., 2011, 137, 1492–1497 CrossRef CAS PubMed.
- Z. H. Jia, Z. H. Liu, J. M. Zheng, C. H. Zeng and L. S. Li, Exp. Clin. Endocrinol. Diabetes, 2007, 115, 571–576 CrossRef CAS PubMed.
- T. Tamura, N. Kosaka, J. Ishiwa, T. Sato, H. Nagase and A. Ito, Osteoarthritis Cartilage, 2001, 9, 257–263 CrossRef CAS PubMed.
- S. Khamdang, M. Takeda, M. Shimoda, R. Noshiro, S. Narikawa, X. L. Huang, A. Enomoto, P. Piyachaturawat and H. Endou, J. Pharmacol. Sci., 2004, 94, 197–202 CrossRef CAS.
- A. Zhang, C. Wang, Q. Liu, Q. Meng, J. Peng, H. Sun, X. Ma, X. Huo and K. Liu, Drug Metab. Dispos., 2013, 41, 994–1003 CrossRef CAS PubMed.
- Y. Uwai, H. Saito and K. Inui, Eur. J. Pharmacol., 2000, 409, 31–36 CrossRef CAS PubMed.
- N. F. Smith, W. D. Figg and A. Sparreboom, Expert Opin. Drug Metab. Toxicol., 2005, 1, 429–445 CrossRef CAS PubMed.
- K. Kock, B. C. Ferslew, I. Netterberg, K. Yang, T. J. Urban, P. W. Swaan, P. W. Stewart and K. L. Brouwer, Drug Metab. Dispos., 2014, 42, 665–674 CrossRef PubMed.
- K. Natarajan, Y. Xie, M. R. Baer and D. D. Ross, Biochem. Pharmacol., 2012, 83, 1084–1103 CrossRef CAS PubMed.
- X. C. Huang, Y. L. Sun, A. A. Salim, Z. S. Chen and R. J. Capon, Biochem. Pharmacol., 2013, 85, 1257–1268 CrossRef CAS PubMed.
- I. A. de Lannoy and M. Silverman, Biochem. Biophys. Res. Commun., 1992, 189, 551–557 CrossRef CAS PubMed.
- L. Wang, X. Pan and D. H. Sweet, Biochem. Pharmacol., 2013, 86, 991–996 CrossRef CAS PubMed.
- L. Ma, L. Zhao, H. Hu, Y. Qin, Y. Bian, H. Jiang, H. Zhou, L. Yu and S. Zeng, J. Ethnopharmacol., 2014, 153, 864–871 CrossRef CAS PubMed.
- B. A. van Gorkom, H. Timmer-Bosscha, S. de Jong, D. M. van der Kolk, J. H. Kleibeuker and E. G. de Vries, Br. J. Cancer, 2002, 86, 1494–1500 CrossRef CAS PubMed.
- L. Ye, L. L. Lu, Y. Li, S. Zeng, X. S. Yang, W. Y. Chen, Q. Feng, W. Liu, L. Tang and Z. Q. Liu, Food Chem. Toxicol., 2013, 58, 301–305 CrossRef CAS PubMed.
- Q. Mao and J. D. Unadkat, AAPS J., 2015, 17, 65–82 CrossRef CAS PubMed.
- Z. Tan, R. Zhu, R. Shi, J. Zhong, Y. Ma, C. Wang, X. Wang and N. Cheng, J. Pharm. Sci., 2013, 102, 1333–1342 CrossRef CAS PubMed.
- B. Smith, N. Li, A. S. Andersen, H. C. Slotved and K. A. Krogfelt, Open Microbiol. J., 2011, 5, 14–17 CrossRef CAS PubMed.
- X. L. Liu, L. J. Li and Z. Chen, Hepatobiliary Pancreatic Dis. Int., 2002, 1, 77–79 Search PubMed.
- X. Liu, K. L. Brouwer, L. S. Gan, K. R. Brouwer, B. Stieger, P. J. Meier, K. L. Audus and E. L. LeCluyse, Pharm. Res., 1998, 15, 1533–1539 CrossRef CAS.
- H. Shimada, Y. Nakamura, T. Nakanishi and I. Tamai, Biochem. Pharmacol., 2015, 98, 629–638 CrossRef CAS PubMed.
- Y. J. Wang, R. J. Kathawala, Y. K. Zhang, A. Patel, P. Kumar, S. Shukla, K. L. Fung, S. V. Ambudkar, T. T. Talele and Z. S. Chen, Biochem. Pharmacol., 2014, 90, 367–378 CrossRef CAS PubMed.
- R. Zhao, T. J. Raub, G. A. Sawada, S. C. Kasper, J. A. Bacon, A. S. Bridges and G. M. Pollack, Drug Metab. Dispos., 2009, 37, 1251–125832 CrossRef CAS PubMed.
- M. Kageyama, K. Fukushima, T. Togawa, K. Fujimoto, M. Taki, A. Nishimura, Y. Ito, N. Sugioka, N. Shibata and K. Takada, Biol. Pharm. Bull., 2006, 29, 779–784 CAS.
- K. Hao, Q. Qi, P. Wan, J. Zhang, H. Hao, Y. Liang, L. Xie, G. Wang and J. Sun, Basic Clin. Pharmacol. Toxicol., 2014, 114, 160–167 CrossRef CAS PubMed.
- L. Ye, L. Lu, Y. Li, S. Zeng, X. Yang, W. Chen, Q. Feng, W. Liu, L. Tang and Z. Liu, Food Chem. Toxicol., 2013, 58, 301–305 CrossRef CAS PubMed.
- L. Zhang, S. Y. Du, Y. Lu, C. Liu, Z. H. Tian, C. Yang, H. C. Wu and Z. Wang, Drug Des., Dev. Ther., 2016, 10, 2227–2237 CrossRef PubMed.
- A. Crowe and A. M. Tan, Toxicol. Appl. Pharmacol., 2012, 260, 294–302 CrossRef CAS PubMed.
- F. Ruschitzka, P. J. Meier, M. Turina, T. F. Luscher and G. Noll, Lancet, 2000, 355, 548–549 CrossRef CAS.
- F. Borrelli and A. A. Izzo, AAPS J., 2009, 11, 710–727 CrossRef CAS PubMed.
- G. K. Panigrahi, A. Yadav, A. Srivastava, A. Tripathi, S. Raisuddin and M. Das, Chem. Res. Toxicol., 2015, 28, 1133–1143 CrossRef CAS PubMed.
- T. Takahashi, T. Ohtsuka, Y. Uno, M. Utoh, H. Yamazaki and T. Kume, Biopharm. Drug Dispos., 2016, 37, 479–490 CrossRef PubMed.
- R. Spence, A. Mandagere, D. B. Richards, M. H. Magee, C. Dufton and R. Boinpally, Clin. Pharmacol. Therapeut., 2010, 88, 513–520 CrossRef CAS PubMed.
- Z. W. Ye, P. Augustijns and P. Annaert, Drug Metab. Dispos., 2008, 36, 1315–1321 CrossRef CAS PubMed.
- P. Annaert, Z. W. Ye, B. Stieger and P. Augustijns, Xenobiotica, 2010, 40, 163–176 CrossRef CAS PubMed.
- H. Watanabe, Y. Sakaguchi, R. Sugimoto, K. Kaneko, H. Iwata, S. Kotani, M. Nakajima, Y. Ishima, M. Otagiri and T. Maruyama, Clin. Exp. Nephrol., 2014, 18, 814–820 CrossRef CAS PubMed.
- Y. Zhu, Q. Meng, C. Wang, Q. Liu, H. Sun, T. Kaku and K. Liu, Peptides, 2012, 33, 265–271 CrossRef CAS PubMed.
- P. Pavek, G. Merino, E. Wagenaar, E. Bolscher, M. Novotna, J. W. Jonker and A. H. Schinkel, J. Pharmacol. Exp. Ther., 2005, 312, 144–152 CrossRef CAS PubMed.
- J. Rautio, J. E. Humphreys, L. O. Webster, A. Balakrishnan, J. P. Keogh, J. R. Kunta, C. J. Serabjit-Singh and J. W. Polli, Drug Metab. Dispos., 2006, 34, 786–792 CrossRef CAS PubMed.
- M. Takeda, R. Noshiro, M. L. Onozato, A. Tojo, H. Hasannejad, X. L. Huang, S. Narikawa and H. Endou, Eur. J. Pharmacol., 2004, 483, 133–138 CrossRef CAS PubMed.
- M. Mesgari Abbasi, H. Valizadeh, H. Hamishekar, L. Mohammadnejad and P. Zakeri-Milani, Adv. Pharm. Bull., 2016, 6, 111–118 CrossRef PubMed.
- Y. Cui, J. Konig, J. K. Buchholz, H. Spring, I. Leier and D. Keppler, Mol. Pharmacol., 1999, 55, 929–937 CAS.
- J. He, Y. Yu, B. Prasad, J. Link, R. S. Miyaoka, X. Chen and J. D. Unadkat, Mol. Pharmacol., 2014, 11, 2745–2754 CrossRef CAS PubMed.
- K. Tollner, C. Brandt, K. Romermann and W. Loscher, Eur. J. Pharmacol., 2015, 746, 167–173 CrossRef PubMed.
- T. Minematsu, T. Hashimoto, T. Aoki, T. Usui and H. Kamimura, Drug Metab. Dispos., 2008, 36, 1496–1504 CrossRef CAS PubMed.
- S. Kunimatsu, T. Mizuno, M. Fukudo and T. Katsura, Drug Metab. Dispos., 2013, 41, 1592–1597 CrossRef CAS PubMed.
|
This journal is © The Royal Society of Chemistry 2017 |