DOI:
10.1039/C6RA28153B
(Paper)
RSC Adv., 2017,
7, 9704-9718
Syntheses, structural diversities and characterization of a series of coordination polymers with two isomeric oxadiazol-pyridine ligands†
Received
13th December 2016
, Accepted 27th January 2017
First published on 2nd February 2017
Abstract
In this work two positional-isomeric oxadiazol-pyridine ligands 3-(5-methyl-1,3,4-oxadiazol-2-yl)pyridine (L1) and 4-(5-methyl-1,3,4-oxadiazol-2-yl)pyridine (L2) have been designed and synthesized. A series of novel coordination polymers, namely [Cd2(μ2-L1)2(μ2-NCS)4]n (1), {[Cd(L1)(μ2-dca)2(H2O)]·H2O}n (2), {[Cu(μ2-L1)2(NCS)2]·0.5H2O}n (3), {[Ag2(μ2-L1)(μ3-L1)2]·2PF6}n (4), {[Ag3(μ2-L1)4(μ2-CF3SO3)(CF3SO3)]·CF3SO3} (5), {[Cd(L2)2(μ2-NCS)2]}n (6), [Ag(μ2-L2)(μ2-CF3SO3)]n (7) and {[Ag(μ2-L2)]·BF4}n (8) have been isolated. Both 1 and 2 are 2D CdII coordination polymers containing infinite {Cd–NCS–Cd} chains (for 1) or infinite {Cd–dca–Cd} layers (for 2), respectively. 3 is a 2D CuII coordination polymer, in which central metal ions are bridged via a bidentate bridging L1 ligand. While when different AgI salts were introduced into the reaction system, 1D AgI coordination polymers 4 and 5 with diverse coordination modes can be isolated. Furthermore, when the isomeric oxadiazol-pyridine L2 is used to replace L1 in the reaction system, 6–8 can be isolated. 6 is a 2D CdII coordination polymer containing {Cd–NCS–Cd} layers. 7 is a 2D neutral AgI coordination polymer while 8 is a 2D cationic AgI coordination polymer. Variable temperature magnetic susceptibility measurements (2–300 K) reveal anti-ferromagnetic interactions between central copper(II) ions for 3. Solid-state luminescent properties of 1, 2 and 4–8 have been investigated indicating strong fluorescent emissions. Additionally, luminescent measurements illustrate that complex 8 exhibits highly sensitive luminescence sensing for Cr2O72− ions in aqueous solutions with high quenching efficiency Ksv = 2.08 × 104 L mol−1 and low detection limit (0.19 μM (S/N = 3)). 8 also represents the first report of a coordination polymer based on oxadiazol-pyridine derivatives with a luminescence response to Cr2O72− anion pollutants in aqueous solutions.
Introduction
In the past several decades, metal organic coordination polymers have attracted much interest due to their promising applications for gas storage/separation, catalysis, luminescence, drug delivery, sensors and magnetic materials.1–9 Among these coordination materials, luminescent metal organic coordination polymers have received great interest because they can be applied in the selective and sensitive detection of environmental pollutants, which can further protect human health and the environment from harm by these pollutants.10 Their tunable channel size, good structural stability, and large surface area of luminescent coordination polymers make these coordination materials more competitive over other luminescent materials because more analytes can effectively interact with the channel surface areas of the coordination polymers, which can ultimately improve the rate of luminescence response, decreasing the fluorescence detection limit and improving the detection sensitivity.11
In the crystal engineering of functional coordination polymers, the variable selection of metal ions and organic ligands provides endless possible outcomes of the final products. Although these porous coordination polymers with targeted secondary building units (SBUs) and topologies can be synthesized by careful selection of the metal ions and organic ligands, the final structures of the products are still highly influenced by several factors including metal–ligand ratio, pH, solvent, temperature, and soon. In particular, judicious selection of ligands is important for deliberate structural changes and functional properties of coordination polymers.12 Therefore many new flexible or rigid ligands have been explored and assembled into metal–organic polymers via coordination bonds or supramolecular interactions such as hydrogen bonds, π–π stacking, and host–guest ionic interactions et al.13–16
1,3,4-Oxidazoles can be employed as one kind of efficient bridging ligands and offer a range of charge-balance requirements, alternative linking modes and different orientations of donor groups.17,18 Currently oxidazole-pyridine and their derivatives have gained more interest as ligands to bridge different metal ions to form functional coordination polymers because of their intriguing bridging fashions.19 It is noted that investigation of the substituent position isomerization influence of oxidazole-pyridine ligands on the formation of coordination polymers is still scarcely reported. Especially, to the best of our knowledge, 3-(5-methyl-1,3,4-oxadiazol-2-yl)pyridine (L1) and 4-(5-methyl-1,3,4-oxadiazol-2-yl)pyridine (L2) are never reported in the coordination chemistry. The development of new ligand systems is continuously an important aspect for the chemistry of metal–organic coordination polymer. Previously we also reported a series of functional coordination polymers using versatile N-containing ligands.20 In this work two position-isomeric oxadiazol-pyridine ligands L1 and L2 have been employed, a series of novel coordination polymers, namely [Cd2(μ2-L1)2(μ2-NCS)4]n (1), {[Cd(L1)(μ2-dca)2(H2O)]·H2O}n (2), {[Cu(μ2-L1)2(NCS)2]·0.5H2O}n (3), {[Ag2(μ2-L1)(μ3-L1)2]·2PF6}n (4), {[Ag3(μ2-L1)4(μ2-CF3SO3)(CF3SO3)]·CF3SO3} (5), {[Cd(L2)2(μ2-NCS)2]}n (6), [Ag(μ2-L2)(μ2-CF3SO3)]n (7) and {[Ag(μ2-L2)]·BF4}n (8) have been isolated. Variable temperature magnetic susceptibility measurements (2–300 K) reveal anti-ferromagnetic interactions between central copper(II) ions for 3. Solid-state luminescent properties of 1, 2 and 4–8 have been investigated indicating strong fluorescent emissions. Additionally, luminescent measurements illustrate that 8 exhibits highly sensitive luminescence sensing for Cr2O72− in aqueous solutions with high quenching efficiency Ksv = 2.08 × 104 L mol−1 and low detection limit (0.19 μM (S/N = 3)), which make it a promising candidate for sensing Cr2O72− ions in practical. Luminescent measurements illustrate that 8 also represents the first report of coordination polymers based on oxadiazol-pyridine derivatives as luminescent response to Cr2O72− ions in the water solutions. Different coordination modes of L1 and L2 are also briefly discussed, which indicates these isomeric oxadiazol-pyridine building blocks have great potential in the construction of these unique metal organic coordination polymers.
Experimental section
General methods
Ligands L1 and L2 were prepared by literature methods.21 All the other reagents were purchased commercially and used without further purification. Deionized water was used as solvent in this work. C, H, and N microanalyses were carried out with a Perkin-Elmer 240 elemental analyzer. FT-IR spectra were recorded from KBr pellets in the range 4000–400 cm−1 on a Bio-Rad FTS 135 spectrometer. Variable-temperature magnetic susceptibilities were measured using a MPMS-7 SQUID magnetometer. Diamagnetic corrections were made with Pascal's constants for all constituent atoms. Photoluminescence spectra were measured by MPF-4 fluorescence spectrophotometer with a xenon arc lamp as the light source.
Synthesis of 3-(5-methyl-1,3,4-oxadiazol-2-yl)pyridine (L1). 35 mmol of the nicotinohydrazide were suspended in 95 mmol (2.7 eq.) triethyl orthoacetate. The reaction mixture was heated under reflux for 12 hours. After cooling to ambient temperature the formed solid was filtered off, washed twice with 15 mL of diethylether and dried in air. Yield: 70% of theory. 1H-NMR (400 MHz, DMSO-d6): δ = 2.61 ppm (s, 3H, CH3); 7.88–7.90 (m, 2H, arom. C–H); 8.80–8.82 (m, 2H, arom. C–H). 13C-NMR (100 MHz, DMSO-d6): δ = 10.7 ppm (s, 1C, CH3); 119.9 (s, 2C, arom. C–H); 130.5 (s, 1C, Cq); 150.9 (s, 2C, arom. C–H); 162.4 (s, 1C, Cq
N); 165.0 (s, 1C, Cq
N). FT-IR (cm−1, KBr): 1565(m), 1549(w), 1465(m), 1430(m), 1349(w), 1247(w), 1131(m), 1086(m), 1044(m), 1014(m), 823(m), 705(m).
Synthesis of 4-(5-methyl-1,3,4-oxadiazol-2-yl)pyridine (L2). 35 mmol of the 4-pyridinecarboxylic hydrazide were suspended in 95 mmol (2.7 eq.) triethyl orthoacetate. The reaction mixture was heated under reflux for 12 hours. After cooling to ambient temperature the formed solid was filtered off, washed twice with 15 mL of diethylether and dried in air. Yield: 55% of theory. 1H-NMR (400 MHz, DMSO-d6): δ = 2.60 ppm (s, 3H, CH3); 7.61–7.64 (m, 1H, arom. C–H); 8.32–8.34 (m, 1H, arom. C–H); 8.78–8.79 (m, 1H, arom. C–H); 9.13 (s, 1H, arom. N
C–H). 13C{1H}-NMR (100 MHz, DMSO-d6): δ = 10.6 ppm (s, 1C, CH3); 120.0 (s, 1C, Cq); 124.3 (s, 1C, Cq); 133.9 (s, 1C, arom. C–H); 146.9 (s, 1C, arom. C–H); 152.2 (s, 1C, arom. C–H); 164.4 (s, 1C, arom. C–H); 162.1 (s, 1C, Cq
N). FT-IR (cm−1, KBr): 1585(m), 1538(w), 1413(m), 1430(m), 1354(w), 1271(m), 1142(m), 1033(m), 834(m), 770(m).
Preparation of compounds 1–8
[Cd2(μ2-L1)2(μ2-NCS)4]n (1). A mixture of CdCl2 (18.33 mg, 0.10 mmol), L1 (17.71 mg, 0.10 mmol), NH4NCS (15.23 mg, 0.20 mmol) and water (20 mL) was refluxed and stirred for 12 h, then the resulting solutions were filtered. The filtrate was kept in a CaCl2 desiccator. After a few days suitable colorless single crystals were obtained for X-ray diffraction study, yield 55%. Elemental analysis calcd (%) for C20H14Cd2N10O2S4: C 30.82%, H 1.81%, N 17.97%; found: C 30.97%, H 1.93%, N 18.16%. FT-IR (cm−1, KBr): 2090(s), 1572(m), 1558(w), 1465(m), 1263(w), 1131(m), 1098(m), 1033(m), 1014(m), 814(m), 719(m).
{[Cd(μ2-L1)(μ2-dca)2(H2O)]·H2O}n (2). A mixture of CdCl2 (18.33 mg, 0.10 mmol), L1 (17.71 mg, 0.10 mmol), Nadca (17.81 mg, 0.20 mmol) and water (20 mL) was refluxed and stirred for 12 h, then the resulting solutions were filtered. The filtrate was kept in a CaCl2 desiccator. After a few days suitable colorless single crystals were obtained for X-ray diffraction study, yield 57%. Elemental analysis calcd (%) for C21H11CdN9O3: C 45.88%, H 2.02%, N 22.93%; found: C 46.15%, H 2.16%, N 23.12%. FT-IR (cm−1, KBr): 3500(m), 2170(s), 1570(m), 1550(w), 1352(m), 1260(w), 1129(m), 1080(m), 1035(m), 1015(m), 815(m), 720(m).
{[Cu(μ2-L1)2(NCS)2]·0.5H2O}n (3). A mixture of CuCl2 (17.05 mg, 0.10 mmol), L1 (17.71 mg, 0.10 mmol), NH4NCS (15.23 mg, 0.20 mmol) and water (20 mL) was refluxed and stirred for 12 h, then the resulting solutions were filtered. The filtrate was kept in a CaCl2 desiccator. After a few days suitable green single crystals were obtained for X-ray diffraction study, yield 37%. Elemental analysis calcd (%) for C18H14.5CuN8O2.5S2: C 42.35%, H 2.86%, N 21.95%; found: C 42.46%, H 2.97%, N 22.13%. FT-IR (cm−1, KBr): 3410(m), 2090(s), 1557(m), 1420(m), 1359(w), 1268(m), 1093(m), 816(m), 719(m).
{[Ag2(μ2-L1)3]·2PF6}n (4). A mixture of AgPF6 (25.29 mg, 0.10 mmol), L1 (17.71 mg, 0.10 mmol) and water (20 mL) was refluxed and stirred for 12 h, then the resulting solutions were filtered. The filtrate was kept in a CaCl2 desiccator. After a few days colorless suitable single crystals were obtained for X-ray diffraction study, yield 59%. Elemental analysis calcd (%) for C24H21Ag2F12N9O3P2: C 29.14%, H 2.14%, N 12.74%; found: C 29.34%, H 2.38%, N 12.97%. FT-IR (cm−1, KBr): 1589(m), 1420(m), 1270(m), 1110(m), 838(s), 704(m).
{[Ag3(μ2-L1)4(μ2-CF3SO3)(CF3SO3)]·CF3SO3}n (5). A mixture of AgCF3SO3 (25.69 mg, 0.10 mmol), L1 (17.71 mg, 0.10 mmol) and water (20 mL) was refluxed and stirred for 12 h, then the resulting solutions were filtered. The filtrate was kept in a CaCl2 desiccator. After a few days suitable single crystals were obtained for X-ray diffraction study, yield 39%. Elemental analysis calcd (%) for C35H28Ag3F9N12O13S3: C 29.70%, H 1.99%, N 11.87%; found: C 29.96%, H 2.06%, N 11.96%. FT-IR (cm−1, KBr): 1589(m), 1410(m), 1275(m), 1168(m), 820(m), 1031(bm), 827(m), 720(m).
{[Cd(L2)2(μ2-NCS)2]}n (6). 6 was prepared through the similar synthetic method of 3 only using L2 (17.71 mg, 0.10 mmol) to replace L1 (17.71 mg, 0.10 mmol) in the reaction system. After a few days suitable colorless single crystals were obtained for X-ray diffraction study, yield 41%. Elemental analysis calcd (%) for C18H14CdN8O2S2: C 39.24%, H 2.56%, N 20.34%; found: C 39.57%, H 2.79%, N 20.59%. FT-IR (cm−1, KBr): 2100 (s), 1617(m), 1567(m), 1251(m), 728(m).
[Ag(μ2-L2)·(μ2-CF3SO3)]n (7). 7 was prepared through the similar synthetic method of 5 only using L2 (17.71 mg, 0.10 mmol) to replace L1 (17.71 mg, 0.10 mmol) in the reaction system. After a few days suitable colorless single crystals were obtained for X-ray diffraction study, yield 36%. Elemental analysis calcd (%) for C9H7Ag2F3N3O4S: C 20.55%, H 1.34%, N 7.99%; found: C 20.76%, H 1.57%, N 8.16%. FT-IR (cm−1, KBr): 1616(m), 1565(m), 1418(m), 1272(m), 1142(m), 1033(m), 834(m), 707(m).
{[Ag(μ2-L2)]·BF4}n (8). A mixture of AgBF4 (0.10 mmol), L2 (0.10 mmol) and water (20 mL) was refluxed and stirred for 12 h, then the resulting solutions were filtered. The filtrate was kept in a CaCl2 desiccator. After a few days suitable single crystals were obtained for X-ray diffraction study, yield 46%. Elemental analysis calcd (%) for C16H14AgBF4N6O2: C 37.17%, H 2.73%, N 16.26%; found: C 37.34%, H 2.97%, N 16.46%. FT-IR (cm−1, KBr): 1616(m), 1566(m), 1416(m), 1266(m), 1051(bm), 836(m), 725(m).
X-ray crystallography
Diffraction intensities for complexes 1–8 were collected on a Bruker SMART 1000 CCD diffractometer with graphite-monochromated Mo-Kα radiation (λ = 0.71073 Å) by using the ω–φ scan technique. Lorentz polarization and MULTI-SCAN absorption corrections were applied. The structures were solved by direct methods and refined with the full-matrix least-squares technique using the SHELXS-97 and SHELXL-97 programs.22,23 Anisotropic thermal parameters were assigned to all non-hydrogen atoms. The positions of hydrogen atoms of the water molecules were located from difference maps and refined with isotropic temperature factors. The positions of other hydrogen atoms were generated geometrically. Analytical expressions of neutral-atom scattering factors were employed, and anomalous dispersion corrections were incorporated. The crystallographic data and details of refinements for compounds 1–8 are summarized in Table 1. Selected bond lengths and angles are listed in Table 2. Corresponding hydrogen bonds lengths and angles for 1–8 are listed in Table S1.†
Table 1 Crystal data and structure refinement information for compounds 1–8
|
1 |
2 |
3 |
4 |
5 |
6 |
7 |
8 |
R1 = Σ||Fo| − |Fc||/|Fo|, wR2 = [Σw(Fo2 − Fc2)2/Σw(Fo2)2]1/2. |
Empricial formula |
C20H14Cd2N10O2S4 |
C21H11CdN9O3 |
C18H14.5CuN8O2.5S2 |
C24H21Ag2F12N9O3P2 |
C35H28Ag3F9N12O13S3 |
C18H14CdN8O2S2 |
C9H7Ag2F3N3O4S |
C16H14AgBF4N6O2 |
Fw |
779.45 |
441.70 |
510.54 |
989.18 |
1415.48 |
550.89 |
418.11 |
517.01 |
Crystal syst. |
Monoclinic |
Monoclinic |
Monoclinic |
Triclinic |
Triclinic |
Monoclinic |
Monoclinic |
Monoclinic |
Temperature (K) |
299(2) |
298(2) |
300(2) |
296(2) |
296(2) |
298(2) |
296(2) |
127.3(6) |
Space group |
C2/c |
C2 |
P2(1)/n |
P![[1 with combining macron]](https://www.rsc.org/images/entities/char_0031_0304.gif) |
P![[1 with combining macron]](https://www.rsc.org/images/entities/char_0031_0304.gif) |
P2(1)/c |
P2(1)/n |
I2/a |
Space group number |
15 |
12 |
11 |
2 |
2 |
14 |
14 |
15 |
Flack parameter |
— |
0 |
— |
— |
— |
— |
— |
— |
a (Å) |
12.402(4) |
25.8009(18) |
8.9845(5) |
9.0183(13) |
8.6773(11) |
14.0427(13) |
8.1723(5) |
13.7987(5) |
b (Å) |
15.8257(5) |
8.5402(6) |
10.6321(6) |
10.8981(17) |
15.4665(19) |
10.0230(10) |
19.7588(12) |
11.4490(5) |
c (Å) |
14.732(5) |
7.8195(5) |
11.8557(7) |
19.524(3) |
18.997(2) |
7.5702(7) |
8.6182(5) |
12.1306(6) |
α (°) |
90 |
90 |
90 |
104.313(3) |
77.458(3) |
90 |
90 |
90 |
β (°) |
112.998(11) |
100.454(2) |
107.885(2) |
91.093(3) |
82.510(3) |
102.733(3) |
107.0410(10) |
96.794(4) |
γ (°) |
90 |
90 |
90 |
112.549(3) |
76.521(2) |
90 |
90 |
90 |
V (Å3) |
2661.6(15) |
1694.4(2) |
1077.78(11) |
1703.2(4) |
2411.6(5) |
1039.30(17) |
1330.52(14) |
1902.95(15) |
Z |
4 |
4 |
2 |
2 |
2 |
2 |
4 |
4 |
F(000) |
1520 |
872 |
519 |
968 |
1392 |
548 |
816 |
1024 |
ρ (mg m−3) |
1.945 |
1.731 |
1.573 |
1.929 |
1.949 |
1.760 |
2.087 |
1.805 |
Abs coeff (mm−1) |
1.952 |
1.322 |
1.243 |
1.353 |
1.444 |
1.286 |
1.727 |
1.124 |
Data/restraints/params |
2596/0/176 |
3295/1/227 |
2104/0/153 |
1281/30/97 |
11794/0/676 |
2025/0/143 |
2753/0/190 |
1685/49/160 |
GOF |
1.168 |
1.011 |
1.045 |
1.005 |
1.016 |
1.056 |
1.069 |
1.094 |
R1a (I = 2σ(I)) |
0.0292 |
0.0220 |
0.0340 |
0.0549 |
0.0482 |
0.0297 |
0.0289 |
0.0414 |
wR2a (all data) |
0.0363 |
0.0231 |
0.0445 |
0.0858 |
0.0956 |
0.0363 |
0.0343 |
0.0439 |
Table 2 Selected bond lengths (Å) and angles (°) for 1–8a
Symmetry transformations used to generate equivalent atoms: for 1: (A) −x, y, −z + 1/2; (B) x + 1, y, z. For 2: (A) x, y, z − 1. For 3: (A) −x, −y + 1, −z + 1. For 4: (A) x + 1, y, z. For 5: (A) x + 2, y − 1, z + 1. For 6: (A) −x + 2, −y + 1, −z; (B) x, −y + 1/2, z − 1/2. For 7: (A) x − 1, −y + 3/2, z + 1/2. For 8: (A) 3/2 − x, y, 2 − z; (B) 1 − x, 1/2 + y, 3/2 − z. |
1 |
Cd(1)–N(4A) |
2.302(4) |
Cd(1)–N(4) |
2.302(4) |
Cd(2)–N(1) |
2.402(3) |
Cd(1)–N(3A) |
2.431(3) |
Cd(1)–N(3) |
2.431(3) |
Cd(2)–N(1A) |
2.402(3) |
Cd(1)–S(2A) |
2.7140(15) |
Cd(1)–S(2) |
2.7140(15) |
Cd(2)–N(5B) |
2.291(4) |
Cd(2)–N(5) |
2.291(4) |
N(4)–Cd(1)–S(2A) |
93.70(10) |
N(3A)–Cd(1)–S(2A) |
81.44(9) |
N(4A)–Cd(1)–N(4) |
102.64(18) |
N(4A)–Cd(1)–N(3A) |
170.09(13) |
N(4A)–Cd(1)–S(2) |
93.69(10) |
N(4)–Cd(1)–N(3A) |
83.24(12) |
N(4A)–Cd(1)–N(3) |
83.24(12) |
N(3A)–Cd(1)–S(2) |
94.27(9) |
N(4)–Cd(1)–N(3) |
170.09(13) |
N(3A)–Cd(1)–N(3) |
92.13(16) |
S(2A)–Cd(1)–S(2) |
173.86(6) |
![[thin space (1/6-em)]](https://www.rsc.org/images/entities/char_2009.gif) |
2 |
Cd(1)–N(4) |
2.261(3) |
Cd(1)–N(6A) |
2.264(4) |
Cd(1)–N(1) |
2.358(3) |
Cd(1)–N(7) |
2.326(4) |
Cd(1)–N(9A) |
2.326(3) |
Cd(1)–O(2) |
2.363(2) |
N(4)–Cd(1)–N(6A) |
98.40(13) |
N(4)–Cd(1)–N(7) |
92.38(14) |
N(4)–Cd(1)–N(1) |
90.76(11) |
N(6A)–Cd(1)–N(7) |
91.66(17) |
N(4)–Cd(1)–N(9A) |
168.22(14) |
N(7)–Cd(1)–N(1) |
173.45(13) |
N(6A)–Cd(1)–N(9A) |
93.34(14) |
N(7)–Cd(1)–N(9A) |
86.39(16) |
N(4)–Cd(1)–O(2) |
84.10(11) |
N(6)–Cd(1)–N(1) |
93.57(14) |
N(7)–Cd(1)–O(2) |
86.59(13) |
N(6A)–Cd(1)–O(2) |
177.01(13) |
N(9A)–Cd(1)–N(1) |
89.37(13) |
N(1)–Cd(1)–O(2) |
88.01(9) |
N(9A)–Cd(1)–O(2) |
84.13(12) |
![[thin space (1/6-em)]](https://www.rsc.org/images/entities/char_2009.gif) |
3 |
Cu(1)–N(4) |
1.974(2) |
Cu(1)–N(4A) |
1.974(2) |
Cu(1)–N(1A) |
2.0578(19) |
Cu(1)–N(1) |
2.0578(19) |
N(4A)–Cu(1)–N(1A) |
88.99(8) |
N(1)–Cu(1)–N(1A) |
180.0 |
N(4)–Cu(1)–N(4A) |
180.0 |
N(4)–Cu(1)–N(1) |
88.99(8) |
N(4)–Cu(1)–N(1A) |
91.01(8) |
N(4A)–Cu(1)–N(1) |
91.01(8) |
N(4)–Cu(1)–N(1A) |
91.01(8) |
|
|
![[thin space (1/6-em)]](https://www.rsc.org/images/entities/char_2009.gif) |
4 |
Ag(1)–N(2) |
2.277(4) |
Ag(1)–N(4A) |
2.307(4) |
Ag(2)–N(7) |
2.421(4) |
Ag(1)–N(8) |
2.309(4) |
Ag(1)–N(3) |
2.406(4) |
Ag(2)–N(1) |
2.554(4) |
Ag(2)–N(6) |
2.225(4) |
Ag(2)–N(9A) |
2.231(4) |
N(4A)–Ag(1)–N(3) |
98.30(17) |
N(2)–Ag(1)–N(4A) |
124.79(16) |
N(2)–Ag(1)–N(8) |
111.05(14) |
N(6)–Ag(2)–N(9A) |
152.34(16) |
N(4A)–Ag(1)–N(8) |
109.87(16) |
N(2)–Ag(1)–N(3) |
102.49(14) |
N(8)–Ag(1)–N(3) |
108.07(14) |
![[thin space (1/6-em)]](https://www.rsc.org/images/entities/char_2009.gif) |
5 |
Ag(1)–N(4) |
2.209(4) |
Ag(1)–N(12A) |
2.211(4) |
Ag(3)–N(10) |
2.154(3) |
Ag(1)–N(1) |
2.458(4) |
Ag(2)–N(7) |
2.284(3) |
Ag(3)–N(9) |
2.152(3) |
Ag(2)–N(3) |
2.297(4) |
Ag(2)–N(6) |
2.352(4) |
Ag(2)–O(5) |
2.591(4) |
N(4)–Ag(1)–N(1) |
103.68(13) |
N(12A)–Ag(1)–N(1) |
104.13(14) |
N(3)–Ag(2)–O(5) |
77.79(13) |
N(7)–Ag(2)–N(3) |
143.19(14) |
N(7)–Ag(2)–N(6) |
109.20(13) |
N(9)–Ag(3)–N(10) |
175.45(15) |
N(3)–Ag(2)–N(6) |
104.96(13) |
N(7)–Ag(2)–O(5) |
94.06(14) |
N(6)–Ag(2)–O(5) |
123.73(15) |
![[thin space (1/6-em)]](https://www.rsc.org/images/entities/char_2009.gif) |
6 |
Cd(1)–N(4) |
2.325(3) |
Cd(1)–N(4A) |
2.325(3) |
Cd(1)–S(1A) |
2.7253(9) |
Cd(1)–N(1) |
2.364(2) |
Cd(1)–N(1A) |
2.364(2) |
Cd(1)–S(1B) |
2.7253(9) |
N(4)–Cd(1)–N(4A) |
180.00(15) |
N(4)–Cd(1)–N(1) |
90.51(9) |
N(4)–Cd(1)–S(1A) |
91.75(8) |
N(4A)#1–Cd(1)–N(1) |
89.49(9) |
N(1)–Cd(1)–S(1B) |
90.37(6) |
N(1)–Cd(1)–S(1A) |
89.63(6) |
![[thin space (1/6-em)]](https://www.rsc.org/images/entities/char_2009.gif) |
7 |
Ag(1)–N(1) |
2.165(2) |
Ag(1)–N(3A) |
2.184(2) |
Ag(1)–O(2A) |
2.623(2) |
Ag(1)–O(4) |
2.710(2) |
N(1)–Ag(1)–N(3A) |
164.72(9) |
N(1)–Ag(1)–O(4) |
94.19(1) |
N(1)–Ag(1)–O(2A) |
99.27(1) |
N(3A)–Ag(1)–O(4) |
83.54(1) |
|
|
![[thin space (1/6-em)]](https://www.rsc.org/images/entities/char_2009.gif) |
8 |
Ag(1)–N(1) |
2.282(3) |
Ag(1)–N(1) |
2.282(3) |
Ag(1)–N(3A)#1 |
2.349(3) |
Ag(1)–N(3B) |
2.349(3) |
N(1)–Ag(1)–N(3A) |
125.45(12) |
N(3A)–Ag(1)–N(3B) |
94.65(17) |
N(1)–Ag(1)–N(1) |
121.29(18) |
N(1)–Ag(1)–N(3) |
125.45(12) |
N(1)–Ag(1)–N(3) |
94.85(12) |
N(1)–Ag(1)–N(3) |
94.85(12) |
|
|
|
|
Results and discussion
The crystal samples of complexes 1–8 can retain their crystalline integrity at room temperature for two weeks. As shown in Schemes 1 and 2, only nitrogen atoms of oxadiazole and pyridine rings can participate in coordination and the steric effect prevents oxadiazole oxygen atoms from metal coordination. There are five different coordination modes in 1–8: for 1, 3 and 5, these L1 ligands in these coordination polymers contain bridging coordination modes a. For 2, these L1 ligands contain mono-dentate coordination mode b, while in 4 two different coordination (bidentate bridging mode a and tridenate bridging mode c) for L1 can be observed. For 6, mono-dentate coordination mode d for L2 can be observed while for 7–8 bi-dentate bridging coordination mode for L2 can be observed. These various different coordination modes also draw the self-assembly of coordination polymers to form different coordination polymers.
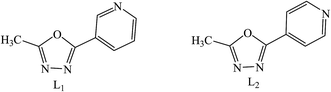 |
| Scheme 1 Two isomeric oxadiazol-pyridine ligands 3-(5-methyl-1,3,4-oxadiazol-2-yl)pyridine (L1) and 3-(5-methyl-1,3,4-oxadiazol-2-yl)pyridine (L2). | |
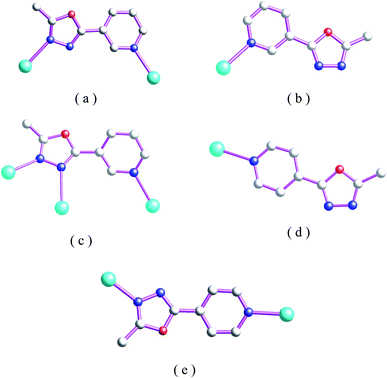 |
| Scheme 2 Five different coordination modes of L1 and L2 in 1–8 (color code: cyan, metal; blue, N; light grey, C). | |
Structure of [Cd2(μ2-L1)2(μ2-NCS)4]n (1)
As shown in Fig. 1, the asymmetric structural unit of [Cd2(μ2-L1)2(μ2-NCS)4]n (1) contains two central CdII ions (Cd1 and Cd2), two μ2-bridging L1 ligands and two μ2-bridging SCN− groups. The central CdII atom is six-coordinated by two nitrogen atoms from L1, two SCN− sulfur atoms and two SCN− nitrogen atoms forming CdN4S2 donor set. The Cd–N distances vary from 2.302(4) to 2.431(2) Å, Cd–S distances are 2.7140(15) Å, all the N–Cd–N and N–Cd–S angles fall in the range of 83.24(12)–94.27(9)°, such coordination mode is in line with an octahedrally coordinated CdII center.
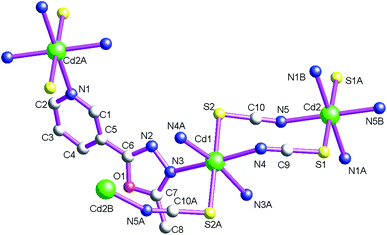 |
| Fig. 1 The fundamental structural unit of [Cd2(μ2-L1)2(μ2-NCS)4]n (1). | |
As shown in Fig. 2, these thiocyanate ligands adopt bidentate bridging coordination mode, which link these neighboring CdII centers forming {Cd–NCS–Cd} chains. The shortest Cd⋯Cd distance within the {Cd–NCS–Cd} chains is 5.714(20) Å. On the other hand, as listed in Scheme 2(a), these L1 ligands in 1 adopt μ2-bridging coordination mode. These {Cd–NCS–Cd} chains are further interlinked via bi-dentate L1 ligands forming the 2D coordination polymer. These L1 carbon atoms (C(4) and C(8)) and SCN− nitrogen and sulfur atoms (N(4) and S(1)) generate these non-classical hydrogen bonds (C(4)–H(4)⋯N(4), 3.448(6) Å; C(8)–H(8B)⋯S(1), 3.652(5) Å), which further stabilize the 2D coordination polymer 1.24,25
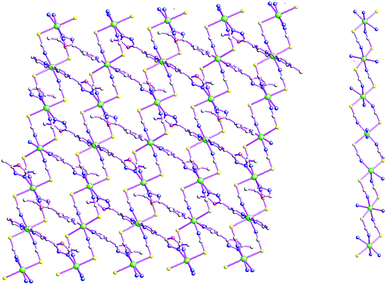 |
| Fig. 2 2D coordination polymer [Cd2(μ2-L1)2(μ2-NCS)4]n (1) containing infinite {Cd–NCS–Cd} chains. | |
Structure of {[Cd(L1)(μ2-dca)2(H2O)]·H2O}n (2)
The asymmetric structural unit of {[Cd(μ2-L1)(μ2-dca)2(H2O)]·H2O}n (2) contains one central CdII ion (Cd1), one μ2-bridging L1 ligand, two μ2-bridging dca− groups and one coordinated water molecule (O2) and one lattice aqua molecule (O3) (Fig. 3). The central CdII atom is six-coordinated by one nitrogen atom (N1) from one L1, four dca− nitrogen atoms (N4, N7, N6A and N9A) and one terminal aqua molecule (O2) forming CdN5O donor set. The Cd–N distances are 2.261(3)–2.326(4) Å, Cd–O distances are 2.363(2) Å. All the N–Cd–N and N–Cd–O angles fall in the range of 84.10(11)–98.40(13)°, such coordination mode is in accordance with an octahedrally coordinated CdII center.
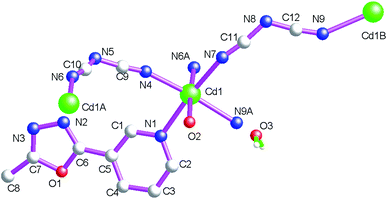 |
| Fig. 3 The fundamental structural unit of {[Cd(L1)(μ2-dca)2(H2O)]·H2O}n (2). | |
As shown in Fig. 4, these bidentate bridging dca− ligands connect these neighboring CdII centers forming {Cd–dca–Cd} layers. The shortest Cd⋯Cd distances within the {Cd–dca–Cd} layers are 7.819(1) Å and 8.575(4) Å. On the other hand, as listed in Scheme 2(b), these L1 ligands in 2 adopt mono-dentate coordination mode. Therefore these {Cd–NCS–Cd} layers are further decorated via these mono-dentate L1 ligands. These coordinated aqua molecules (O(2)), lattice aqua molecules (O(3)), L1 nitrogen atoms (N(3)) and dca− nitrogen atoms (N(8)) generate these classical hydrogen bonds (O(2)–H(2A)⋯O(3), 2.740(4) Å; O(2)–H(2B)⋯N(3), 2.854(4) Å; O(3)–H(3A)⋯O(2), 2.965(4) Å; O(3)–H(3B)⋯N(8), 2.970(6) Å), which further stabilize the 2D coordination polymer 2.
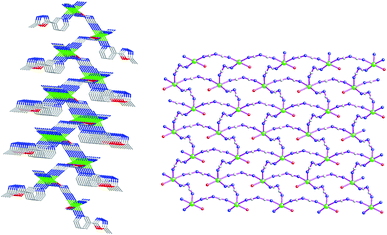 |
| Fig. 4 Side view of 2D coordination polymer {[Cd(L1)(μ2-dca)2(H2O)]·H2O}n (2) containing infinite {Cd–dca–Cd} layers. | |
Structure of {[Cu(μ2-L1)2(NCS)2]·0.5H2O}n (3)
As shown in Fig. 5, the asymmetric structural unit of 3 contains one central CuII ion (Cu1), one μ2-bridging L1 ligand and two terminal bridging SCN− groups. The central CuII atom is six-coordinated by four nitrogen atoms (N1, N1A, N3A and N3B) from four L1, two thiocyanate nitrogen atoms (N4 and N4A) forming the CuN6 donor set. The Cu–N distances are 1.974(2)–2.0578(19) Å, all the N–Cu–N angles fall in the range of 88.99(8)–91.01(8)°, such coordination mode is in accordance with an octahedrally coordinated CuII center.
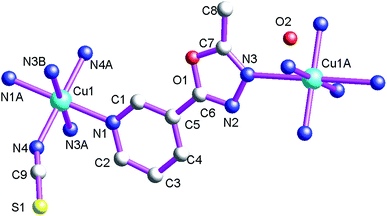 |
| Fig. 5 The fundamental structural unit of {[Cu(μ2-L1)2(NCS)2]·0.5H2O}n (3). | |
As shown in Fig. 6 and Scheme 2(a), these L1 ligands in 3 also adopt μ2-bridging coordination mode. These L1 ligands connect neighboring CuII centers forming the 2D coordination polymer 3. The corresponding Cu⋯Cu distance within the 2D coordination polymer is 9.997(5) Å. These L1 carbon molecules (C(1)) and L1 nitrogen atoms (N(2)) generate these non-classical hydrogen bonds (C(1)–H(1)⋯N(2), 3.304(3) Å), which also further extend 3 into a 3D supramolecular architecture. Such weak intermolecular interactions are key for the stabilization of the 2D coordination polymer 3.
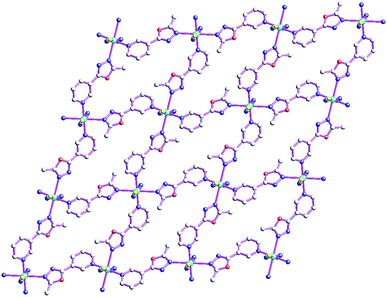 |
| Fig. 6 2D coordination polymer {[Cu(μ2-L1)2(NCS)2]·0.5H2O}n (3). | |
Structure of {[Ag2(μ2-L1)(μ3-L1)2]·2PF6}n (4)
When AgBF4 are used, 1D Ag(I) coordination polymers, {[Ag2(μ3-L1)2(μ2-L1)]·2PF6}n (4), can be isolated. For 4, the coordination polymer crystallizes in triclinic P
space group with one formula unit in the asymmetric unit. The asymmetric unit contains two AgI atoms (Ag1 and Ag2), two tri-dentate bridging L1, one bi-dentate bridging L1 and two free PF6− anions (Fig. 7). The central AgI atom (Ag1) is four-coordinated by four nitrogen atoms (N2, N4, N3A and N4A) from four L1 forming AgN4 donor set. The other central AgI atom (Ag2) is also four-coordinated by four nitrogen atoms (N1, N6, N7 and N9A) from four L1 forming AgN4 donor set. The Ag–N distances are 2.225(4)–2.554(4) Å, all the N–Ag–N angles are in the range of 98.30(17)–152.34(16)°, such coordination mode is in accord with a tetrahedrally coordinated AgI center.
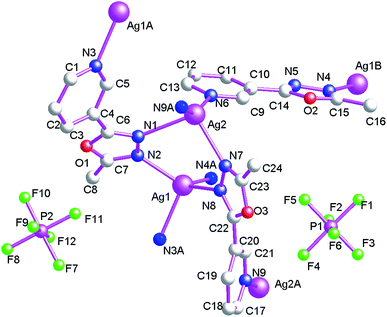 |
| Fig. 7 The fundamental structural unit of {[Ag2(μ2-L1)(μ3-L1)2]·2PF6}n (4). | |
As shown in Fig. 8, Ag1 and Ag2 are inter-linked via oxadiazole nitrogen atoms forming di-nuclear Ag(I) clusters, neighboring Ag⋯Ag distances within di-nuclear Ag(I) clusters are 3.845(6) Å. These bridging L1 ligands connect adjacent AgI ions forming a cluster-based 1D double chain coordination polymer, which is shown along the crystallographic a-axis. These non-classical hydrogen bonds (C(8)–H(8B)⋯F(12), 3.341(9) Å; C(17)–H(17)⋯F(7), 3.190(10) Å; C(17)–H(17)⋯F(8), 3.347(10) Å; C(21)–H(21)⋯N(5), 3.504(8) Å) also can be observed, which further extend 4 into a 3D supramolecular architecture. Such weak intermolecular interactions are important for the stabilization of the 1D cluster-based AgI coordination polymer 4.
 |
| Fig. 8 1D AgI coordination polymer {[Ag2(μ2-L1)(μ3-L1)2]·2PF6}n (4). | |
Structure of {[Ag3(μ2-L1)4(μ2-CF3SO3)(CF3SO3)]·CF3SO3} (5)
When AgCF3SO3 is used, 1D Ag(I) coordination polymer, {[Ag3(μ2-L1)4(μ2-CF3SO3)(CF3SO3)]·CF3SO3} (5), can be isolated. For 5, the coordination polymer also crystallizes in triclinic P
space group with one formula unit in the asymmetric unit. The asymmetric unit contains three AgI atoms (Ag1, Ag2 and Ag3), four bi-dentate bridging L1, two terminal CF3SO3− anions and one free CF3SO3− anion (Fig. 9). The central AgI atom (Ag1) is three-coordinated by four nitrogen atoms (N4, N1 and N12A) from three L1 forming AgN3 donor set. The central AgI atom (Ag2) is four-coordinated by three nitrogen atoms (N3, N6 and N7) from three L1 and one CF3SO3− oxygen atom (O5) forming AgN3O donor set. The central AgI atom (Ag3) is three-coordinated by two nitrogen atoms (N9 and N10) from two L1 and one CF3SO3− oxygen atom (O9) forming AgN2O donor set. The Ag–N distances vary from 2.209(4) Å to 2.458(4) Å, Ag–O distances are 2.591(4) Å, all the N–Ag–N and N–Ag–O angles fall in the range of 77.79(13)–143.19(14)°, All the bond distances and bond angles all fall into the normal range.
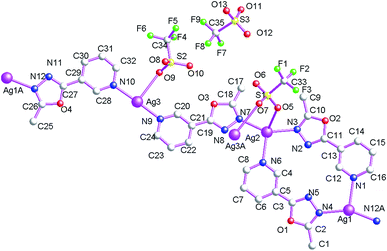 |
| Fig. 9 The fundamental structural unit of {[Ag3(μ2-L1)4(CF3SO3)2]·CF3SO3}(5). | |
As shown in Fig. 10, L1 adopt bi-dentate bridging coordination mode, which connect neighboring Ag1, Ag2 and Ag3, forming 1D double chain coordination polymer 5. The shortest neighboring Ag⋯Ag distances within 1D double chain are 4.478(2) and 4.858(8) Å. These non-classical hydrogen bonds (C(1)–H(1A)⋯O(13), 3.348(7) Å; C(4)–H(4)⋯N(2), 3.259(6) Å; C(12)–H(12)⋯N(5), 3.241(6) Å; C(15)–H(15)⋯O(11), 3.393(7) Å) also can be observed, which further extend 5 into a 3D supramolecular architecture. Such weak intermolecular interactions are key for the stabilization of 1D AgI double chain coordination polymer 5.
 |
| Fig. 10 1D AgI coordination polymer {[Ag3(μ2-L1)4(μ2-CF3SO3)(CF3SO3)]·CF3SO3}(5). | |
Structure of {[Cd(L2)2(μ2-NCS)2]}n (6)
As shown in Fig. 11, the asymmetric structural unit of {[Cd(L2)2(μ2-NCS)2]}n (6) contains one central CdII ions (Cd1), two terminal coordinated L1 ligands and two μ2-bridging NCS− groups. The central CdII atom is six-coordinated by two nitrogen atoms (N1 and N1A) from two L2, two nitrogen atoms (N4 and N4A) and two sulfur atoms (S1A and S1B) form four NCS− groups forming CdN4S2 donor set. The Cd–N distances vary from 2.325(3) to 2.364(2) Å, Cd–S distances are 2.7253(9) Å. All the N–Cd–N and N–Cd–O angles are in the range of 89.63(6)–91.75(8)°, such coordination mode is in accordance with an octahedrally coordinated CdII center.
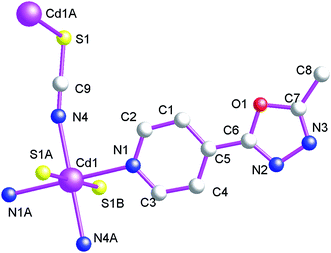 |
| Fig. 11 The fundamental structural unit of {[Cd(μ2-L2)2(μ2-NCS)2]}n (6). | |
As shown in Fig. 12, these bidentate bridging NCS− ligands connect neighboring CdII centers forming the {Cd–NCS–Cd} layers. The shortest distances of Cd⋯Cd within the {Cd–NCS–Cd} layers are 6.280(1) and 7.570(1) Å. On the other hand, as listed in Scheme 2(b), these L2 ligands in 6 adopt mono-dentate coordination mode. Therefore these {Cd–NCS–Cd} layers are further decorated via these mono-dentate L2 ligands. These L2 carbon atoms (C(1)) and nitrogen atoms (N(3)) generate non-classical hydrogen bonds (C(1)–H(1)⋯N(3), 3.528(4) Å), which further stabilize the 2D coordination polymer 6.
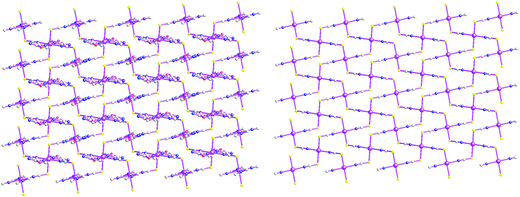 |
| Fig. 12 2D coordination polymer {[Cd(μ2-L2)2(μ2-NCS)2]}n (6) containing infinite {Cd–NCS–Cd} layers. | |
Structure of [Ag(μ2-L2)·(μ2-CF3SO3)]n (7)
As shown in Fig. 13, the asymmetric structural unit of [Ag(μ2-L2)·(μ2-CF3SO3)]n (7) contains one central AgI ions (Ag1), one μ2-bridging L2 ligand, one μ2-bridging CF3SO3− group. For 7, the coordination polymer crystallizes in monoclinic P21/n space group with one formula unit in the asymmetric unit. The central AgI atom (Ag1) is four-coordinated by two nitrogen atoms (N1 and N3A) from two L1 and two CF3SO3− oxygen atoms (O2A and O4) forming AgN2O2 donor set. Ag–N distances are 2.165(2)–2.184(2) Å, Ag–O distances are 2.623(2)–2.710(2) Å, all the N–Ag–N and N–Ag–O angles are in the range of 83.54(1)–94.19(1)°, such coordination mode is in accord with a tetrahedrally coordinated AgI center.
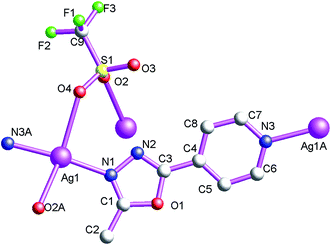 |
| Fig. 13 The fundamental structural unit of [Ag(μ2-L2)(μ2-CF3SO3)]n (7). | |
As shown in Fig. 14, these CF3SO3− anions adopt bi-dentate bridging coordination modes via its two oxygen atoms (O2 and O4). On the other hand, these L2 ligands also adopt bridging coordination mode as listed in Scheme 2(e). These bridging CF3SO3− anions and L2 ligands link these neighboring Ag(I) centers, which ultimately forming the 2D coordination polymer 7. These L2 carbon atoms (C(2) and C(6)) and CF3SO3− oxygen and fluoride atoms (O(4) and F(1)) generate these non-classical hydrogen bonds (C(2)–H(2C)⋯F(1), 3.338(5) Å; C(6)–H(6)⋯O(4), 3.308 Å), which further extend 7 into a 3D supramolecular architecture. Such weak intermolecular interactions are important for the stabilization of the 1D cluster-based AgI coordination polymer 7.
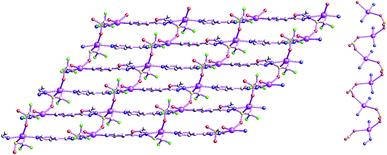 |
| Fig. 14 2D coordination polymer [Ag(μ2-L2)(μ2-CF3SO3)]n (7). | |
Structure of {[Ag(μ2-L2)]·BF4}n (8)
As shown in Fig. 15, the fundamental structural unit of {[Ag(μ2-L2)]·BF4}n (9) contains one central AgI ions (Ag1), one μ2-bridging L2 ligand, one free BF4− group. For 8, the coordination polymer crystallizes in monoclinic I2/a space group with one formula unit in the asymmetric unit. The central AgI atom (Ag1) is four-coordinated by four nitrogen atoms (N1, N3A, N1A and N3B) from four L2 forming AgN4 donor set. The Ag–N distances are 2.282(3)–2.349(3) Å, all the N–Ag–N angles are in the range of 94.65(17)–125.45(12)°, such coordination mode is in accord with a tetrahedrally coordinated AgI center.
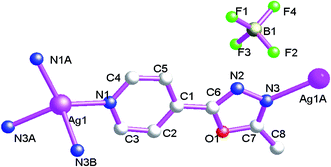 |
| Fig. 15 The fundamental structural unit of {[Ag(μ2-L2)]·BF4}n (8). | |
As shown in Fig. 16, these L2 ligands also adopt bridging coordination mode as listed in Scheme 2(e). These bridging L2 ligands link these neighboring Ag(I) centers, which ultimately forming the 2D grid-like cationic coordination polymer. These non-classical hydrogen bonds (C(4)–H(4)⋯F(2), 3.085(9) Å) also can be observed, which further extend 8 into a 3D supramolecular architecture. Such weak intermolecular interactions are important for the stabilization of the 2D AgI coordination polymer.
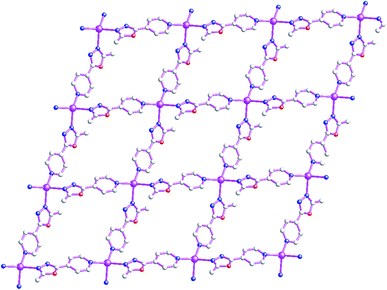 |
| Fig. 16 2D cationic AgI coordination polymer {[Ag(μ2-L2)]·BF4}n (8). | |
Magnetic properties of 3
Variable temperature dc magnetic susceptibility data were collected on a microcrystalline powder sample of 3 in a 1000 Oe field in the temperature range 1.8–300 K. The temperature-dependent magnetic susceptibility data of complexes 3 have been measured in the temperature range of 2–300 K. The diamagnetic correction was evaluated by using Pascal's constants. As shown in Fig. 17, The χmT values are ca. 0.416 cm3 kmol−1 (for 3) at room temperature, which are expected spin-only values for an isolated Cu(II) ion. When the temperature is lowered, χMT values remain constants and then smoothly decrease and drop rapidly below ca. 20 K reaching the minimum values at 2 K. Fitting the magnetic susceptibility data with the Curie–Weiss law χM = C/(T − θ), where C and θ represent the Curie constant and the Weiss temperature, it can give θ values of −1.05 K. These θ values reveal anti-ferromagnetic interactions between central CuII ions. The result of weak anti-ferromagnetic interactions can be attributed to can be attributed to very weak antiferromagnetic interactions as indicated by large metal–metal distances or the presence of zero-field splitting or both in 3.26
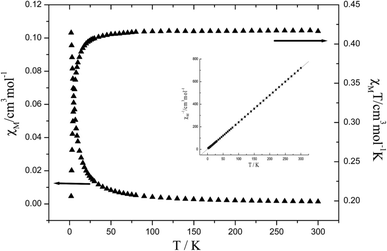 |
| Fig. 17 Variable-temperature magnetic susceptibility data for 3 in the 2–300 K temperature range. | |
Luminescence properties
Coordination polymers have been investigated for potential applications as luminescent materials, such as light-emitting diodes (LEDs).27 Owing to the ability of affecting the emission wavelength and strength of organic materials, syntheses of coordination polymers by judicious choice of conjugated organic spacers and transition metal centers can be an efficient method for obtaining new types of luminescent materials, especially for d10 or d10–d10 systems.28 In the present work, we have explored the luminescent properties of L1, L2 ligands and organic/inorganic coordination polymers 1, 2 and 4–8 based on the ligands in the solid state.
As shown in Fig. 18, at room temperature, free L1 and L2 ligands in the solid state are luminescent, which show the broad emission maximum centered at 360 nm (λex = 280 nm) and 361 nm (λex = 280 nm), respectively. For the free L1 and L2 ligands, the chromophores are aromatic pyridine and oxadiazol rings, the observed emission is due to π–π* transition. In comparison with that of free ligand, upon excitation of solid samples of 1, 2, 6 and 8 show emission bands with maximum at ca. 360 nm (for 1), ca. 361 nm (for 2), ca. 363 nm (for 6) and ca. 362 nm (for 8) respectively. The emissions of 1, 2, 6 and 8 should be assigned to intraligand π–π* transitions.29 The slight shifts of the emission bands relative to free L1 and L2 ligands can be attributed to the coordination action of ligand to central metal ions.30
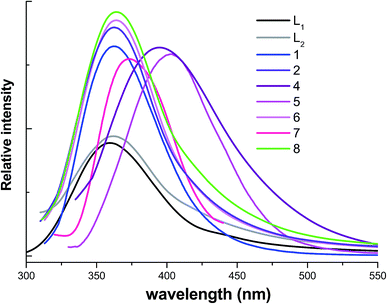 |
| Fig. 18 Solid-state fluorescence spectra of 1–2, 4–8 and L1, L2 ligand molecules. | |
On the other hand, for these 1D or 2D cluster-based AgI coordination polymers 4, 5 and 7, it is noted that red-shift of luminescent emissions can be observed (emission bands with maximum centered at 395 nm for 4 (λex = 300 nm), 403 nm for 5 (λex = 300 nm) and 372 nm for 7 (λex = 300 nm)). The photoluminescence origin of the emission bands should be attributed to metal-to-ligand charge transfer (MLCT) as discussed in the previous literature.1 Because 5 and 6 contain similar 1D structural motifs, different band shapes in the luminescent emissions of 5 and 6 can be ascribed to different guest PF6−/CF3SO3− anions, which can greatly affect luminescence emission bands.31 These cluster-based AgI coordination polymers increase the ligand conformational rigidity, thereby reducing the non-radiative decay of the intraligand (π–π*) excited state. The syntheses of new Cd(II) and Ag(I) complexes with these isomeric oxadiazol-pyridine ligands can be an efficient method for obtaining new types of luminescent materials.32,33
Luminescent sensing properties of Cr2O72− anion pollutants for 8
In general, water pollutions are a global environmental issue, and considerable research attention has been paid to the removal of pollutants from waste water. With the development of modern industry, Cr2O72− anions have been widely used in the field of chromium plating, metallurgy, pigment manufacturing, leather tanning, and wood preservation. These anion pollutants have been a focus of concern because they cause serious damage to human health and the environment. Therefore, the exploration of new materials for the efficient detection of these anion pollutants is highly important.34
In this work, the product of 8 as the luminescent probes was carefully explored for sensing diverse ions, owing to its strong visible blue light when excited by ultraviolet light. The photoluminescence emission bands of 8 in aqueous solutions are centered at around 360 nm. These emission bands should be more likely to be due to intra-ligand transitions because similar photo-luminescent bands can be observed for free L2 ligands in aqueous solutions. On the other hand, water stability of 8 was also investigated, the solid state samples were suspended in aqueous solutions for 12 h, then water solutions were filtrated and solid state samples were dried by the vacuum oven. Then solid state samples were characterized by PXRD patterns. As shown in Fig. S1,† PXRD patterns of solid state samples suspended in the water solutions and these calculated patterns deduced from single-crystal X-ray data are in good agreement. The result indicates that the coordination polymer structure is unchangeable and can retain stable in aqueous solutions.
In order to study the luminescent responses to different anions, the products of 8 were ground into powder and suspended in the water solution containing different salt solutions of the same concentration (10−4 M) of SiF62−, CO32−, HCO32−, BF4−, NO3−, SCN−, Ac−, SO42−, Br−, Cl−, F−, I−, BrO3−, IO3−, CrO42−, HPO42− and PO43− and Cr2O72−. Aqueous solutions of SiF62−, CO32−, HCO32−, BF4−, NO3−, SCN−, Ac−, SO42−, Br−, Cl−, F−, I−, BrO3−, IO3−, CrO42−, HPO42− and PO43− and Cr2O72− were prepared from these corresponding potassium salts, respectively. As shown in Fig. 19(a), Cr2O72− gave significant fluorescence quenching effect, while there was only a negligible effect on the luminescence intensity for other anions. PXRD pattern of 8-Cr2O72− was measured and remains well consistent with the simulated one of 8, indicating that 8 still remains stable. The result indicates the high selectivity of 8 for the detection and specific recognition of Cr2O72− anions in aqueous solutions. Therefore 8 may be chosen as a candidate for the selective sensing of Cr2O72−.
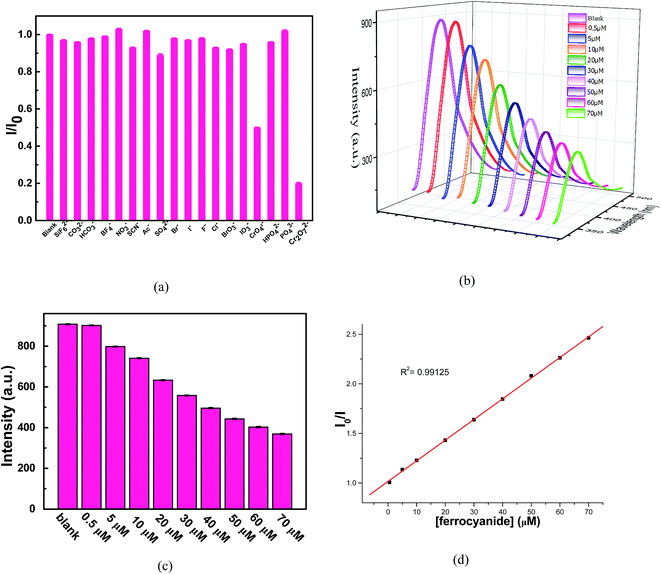 |
| Fig. 19 (a) Comparison of the luminescence intensity of 8 at 360 nm incorporating aqua solutions (10−4 M) upon the addition of various anions; (b) liquid luminescence spectra of 8 under different concentrations of Cr2O72− aqueous solution; (c) comparison of the luminescence intensity of 8 under different concentrations of Cr2O72− anion pollutants aqueous solution. (d) The linear luminescence intensity vs. Cr2O72− anion pollutants concentration plot. | |
Generally, waste water contains more than one type of pollutant anions, and therefore, it is essential to investigate the influence of mixed anions on the luminescence of 8. The detailed experiments are as follows: 0.4 mL of Na2Cr2O7 (10−4 M) and 0.4 mL of other anion solutions (10−4 M) were slowly dropped into a 3.2 mL suspension of 8, respectively. The luminescence measurements of resultant solutions, containing Cr2O72− and other anions, were carried out at once. It is also noted that there was only a negligible effect on the luminescence intensity for Cr2O72− anions. This selective detection of Cr2O72− anions has no interfering effects from other anions such as SiF62−, CO32−, HCO32−, BF4−, NO3−, SCN−, Ac−, SO42−, Br−, Cl−, F−, I−, BrO3−, IO3−, CrO42−, HPO42− and PO43−, suggesting that 8 can selectively detect Cr2O72− anions among the above anions.
Moreover, to explore the detection limit of 8 as a luminescent probe for detecting Cr2O72−, as shown in Fig. 19(b), a series suspension of 8-Cr2O72− (5 μM to 100 μM) were prepared by dropping different concentrations of Cr2O72− solutions into the suspension of 8. The luminescence intensity of 8 gradually decreases with increasing the concentration of Cr2O72−. As shown in Fig. 19(c) and (d), the luminescence intensity linearly decreases with the concentration of Cr2O72− ranging from 0.5 μM to 70 μM. The detection limit of 8 as a luminescent probe for detecting Cr2O72− is supported by the calculated results based on the equation: detection limit = 3σ/k (σ is the standard deviation of blank measurement; k is the slope between the luminescence intensity vs. log[Cr2O72−]). To further explore the relationship between the quenching effect and Cr2O72− concentration, the linear luminescence intensity vs. Cr2O72− concentration plot was made, which can be fitted into I0/I = 1 + Ksv[Cr2O72−] (I0 and I represent the luminescence intensity of 8 before and after adding Cr2O72−, respectively; [Cr2O72−] represents the concentration of Cr2O72−, and Ksv represents the quenching rate constant). The Ksv value is calculated to be 2.08 × 104 L mol−1, indicating the high quenching efficiency of Cr2O72− in the emission of 8.35–37 The high quenching efficiency and low detection limit 0.19 μM (S/N = 3) also reveal that 8 can act as luminescent probes for discrimination and detection of Cr2O72−.
8 is a cationic coordination polymer, in which free BF4− anions are located between these 2D cationic layers, while in other AgI coordination polymers these anions are tightly linked to main frameworks through coordination bonds. As illustrated in the previous literature, these cationic coordination polymers can effectively capture Cr2O72− anions through anion exchange process,38 which may effectively lead to the fluorescent quenching of 8. On the other hand, in comparison with other anions, only Cr2O72− exhibits two wide absorption bands from 230 to 413 nm.39 The bands almost cover the whole ranges of absorption bands that arise from 8. Thus, the luminescence quenching mechanism also further causes by the competition of excitation energy between Cr2O72− anions and 8. Upon illumination, the adsorption of Cr2O72− ions for excitation energy hinders the UV/vis absorption of the target coordination polymer, thus resulting in a decrease, or even full quenching, of the luminescence intensities. This luminescent sensing mechanism for Cr2O72− is consistent with those previously proposed by previous literature.40,41 To the best of our knowledge, work on these metal–organic polymers as luminescent probes for discrimination and detection of Cr2O72− is important and still limited.42 8 also represents the first report of coordination polymers based on oxadiazol-pyridine derivatives as luminescent response to Cr2O72− in the water solutions.
Conclusions and perspectives
In conclusion, in this work two position-isomeric oxadiazol-pyridine ligands 3-(5-methyl-1,3,4-oxadiazol-2-yl)pyridine (L1) and 4-(5-methyl-1,3,4-oxadiazol-2-yl)pyridine (L2) have been designed and synthesized. A series of novel coordination polymers, namely [Cd2(μ2-L1)2(μ2-NCS)4]n (1), {[Cd(L1)(μ2-dca)2(H2O)]·H2O}n (2), {[Cu(μ2-L1)2(NCS)2]·0.5H2O}n (3), {[Ag2(μ2-L1)(μ3-L1)2]·2PF6}n (4), {[Ag3(μ2-L1)4(μ2-CF3SO3)(CF3SO3)]·CF3SO3} (5), {[Cd(L2)2(μ2-NCS)2]}n (6), [Ag(μ2-L2)(μ2-CF3SO3)]n (7) and {[Ag(μ2-L2)]·BF4}n (8) have been isolated. Both 1 and 2 are 2D CdII coordination polymers containing infinite {Cd–NCS–Cd} chains (for 1) or infinite {Cd–dca–Cd} layers (for 2). 3 is a 2D CuII coordination polymer, in which central metal ions are bridged via bidentate bridging L1 ligand. While when different AgI salts were introduced into the reaction system, 1D cluster-based AgI coordination polymers 4 and 5 with diverse coordination modes can be isolated. Further when the isomeric oxadiazol-pyridine L2 is used to replace L1 in the reaction system, 6–8 can be isolated. 6 is a 2D CdII coordination polymer containing 2D {Cd–NCS–Cd} layers. 7 is a 2D neutral AgI coordination polymer while 8 is a 2D cationic AgI coordination polymer. Variable temperature magnetic susceptibility measurements (2–300 K) reveal anti-ferromagnetic interactions between central copper(II) ions for 3. Solid-state luminescent properties of 1, 2 and 4–8 have been investigated indicating strong fluorescent emissions. Additionally, luminescent measurements illustrate that complex 8 also exhibits highly sensitive luminescence sensing for Cr2O72− anion pollutants in aqueous solutions with high quenching efficiency Ksv = 2.08 × 104 L mol−1 and low detection limit (0.19 μM (S/N = 3)). 8 also represents the first report of coordination polymers based on oxadiazol-pyridine derivatives as luminescent response to Cr2O72− anion pollutants in the water solutions. On the basis of this work, further syntheses, structures and properties studies of these coordination polymers using these isomeric oxadiazol-pyridine derivatives as basic building blocks are also under way in our laboratory.
Acknowledgements
This work was supported financially by the Open Project of Key Lab Adv Energy Mat Chem (Nankai Univ), Tianjin Educational Committee (20120508), Natural Science Foundation of Tianjin (Grant no. 14JCQNJC05900), Young Scientist Fund (Grant no. 21301128), National Natural Science Foundation of China (Grant No. 21375095) and the Program for Innovative Research Team in University of Tianjin (TD12-5038).
References
-
(a) D. Venkataraman, S. Lee, J. S. Moore, P. Zhang, K. A. Hirsch, G. B. Gardner, A. C. Covey and C. L. Prentice, Chem. Mater., 1996, 8, 2030 CrossRef CAS;
(b) O. M. Yaghi, T. J. Pinnavaia and M. F. Thorpe, in Access in Nanoporous Materials, ed. Plenum, New York, 1995, p. 111 Search PubMed;
(c) A. Stein, S. W. Keller and T. E. Mallouk, Science, 1993, 259, 1558 CAS;
(d) P. J. Fagan and M. D. Ward, Sci. Am., 1992, 267, 48 CrossRef CAS;
(e) Inclusion Compounds, ed. T. Iwamoto, J. L. Atwood, J. E. D. Davies and D. D. MacNicol, Oxford, New York, 1991, vol. 5, p. 177 Search PubMed;
(f) B. F. Hoskins and R. Robson, J. Am. Chem. Soc., 1990, 112, 1546 CrossRef CAS;
(g) J. Lu, W. T. A. Harrison and A. J. Jacobson, Angew. Chem., Int. Ed., 1995, 34, 2557 CrossRef CAS.
-
(a) B. Moulton and M.-J. Zaworotko, Chem. Rev., 2001, 101, 1629 CrossRef CAS PubMed;
(b) O. M. Yaghi, M. O'Keeffe, N. W. Ockwig, H. K. Chae, M. Eddaoudi and J. Kim, Nature, 2003, 423, 706 CrossRef PubMed;
(c) S. Kitagawa, R. Kitaura and S. I. Noro, Angew. Chem., Int. Ed., 2004, 43, 2334 CrossRef CAS PubMed;
(d) G. Kubas, Chem. Rev., 2007, 107, 4152 CrossRef CAS PubMed.
-
(a) M. Eddaoudi, J. Kim, N. Rosi, D. Vodak, J. Wachter, M. O'Keeffe and O. Yaghi, Science, 2002, 295, 469 CrossRef CAS PubMed;
(b) S. Bourrelly, P. Llewellyn, C. Serre, F. Millange, T. Loiseau and G. Férey, J. Am. Chem. Soc., 2005, 127, 13519 CrossRef CAS PubMed;
(c) B. Panella, M. Hirscher, H. Puetter and U. Mueller, Adv. Funct. Mater., 2006, 16, 520 CrossRef CAS;
(d) Y. Liu, J. F. Eubank, A. J. Cairns, J. Eckert, V. C. Kravtsov, R. Luebke and M. Eddaoudi, Angew. Chem., Int. Ed., 2007, 46, 3278 CrossRef CAS PubMed;
(e) A. Phan, C. J. Doonan, F. J. Uribe-Romo, C. B. Knobler, M. O'Keeffe and O. M. Yaghi, Acc. Chem. Res., 2010, 43, 58 CrossRef CAS PubMed.
-
(a) R.-B. Getman, Y. S. Bae, C.-E. Wilmer and R.-Q. Snurr, Chem. Rev., 2011, 112, 703 CrossRef PubMed;
(b) P. Horcajada, R. Gref, T. Baati, P.-K. Allan, G. Maurin, P. Couvreur, G. Férey, R.-E. Morris and C. Serre, Chem. Rev., 2011, 112, 1232 CrossRef PubMed;
(c) L.-E. Kreno, K. Leong, O.-K. Farha, M. Allendorf, R.-P. Van Duyne and J.-T. Hupp, Chem. Rev., 2011, 112, 1105 CrossRef PubMed;
(d) J.-R. Li, J. Sculley and H.-C. Zhou, Chem. Rev., 2011, 112, 869 CrossRef PubMed.
-
(a) J. R. Li, R. J. Kuppler and H. C. Zhou, Chem. Soc. Rev., 2009, 38, 1477 RSC;
(b) R. Vaidhyanathan, S. S. Iremonger, G. K. H. Shimizu, P. G. Boyd, S. Alavi and T. K. Woo, Science, 2010, 330, 650 CrossRef CAS PubMed;
(c) P. Nugent, Y. Belmabkhout, S.
D. Burd, A. J. Cairns, R. Luebke, K. Forrest, T. Pham, S. Ma, B. Space, L. Wojtas, M. Eddaoudi and M. J. Zaworotko, Nature, 2013, 495, 80 CrossRef CAS PubMed;
(d) H. Yin, J. Wang, Z. Xie, J. Yang, J. Bai, J. Lu, Y. Zhang, D. Yin and J. Y. S. Lin, Chem. Commun., 2014, 50, 3699 RSC.
-
(a) R. Kitaura, K. Fujimoto, S. Noro, M. Kondo and S. Kitagawa, Angew. Chem., Int. Ed., 2002, 41, 133 CrossRef CAS;
(b) R. Kitaura, G. Onoyama, H. Sakamoto, R. Matsuda, S. Noro and S. Kitagawa, Angew. Chem., Int. Ed., 2004, 43, 2684 CrossRef CAS;
(c) B. Chen, F. R. Fronczek and A. W. Maverick, Inorg. Chem., 2004, 43, 8209 CrossRef CAS PubMed;
(d) P. A. Maggard, B. Yan and J. Luo, Angew. Chem., Int. Ed., 2005, 44, 2 CrossRef PubMed;
(e) R. Custelcean and M. G. Gorbunova, J. Am. Chem. Soc., 2005, 127, 16362 CrossRef CAS PubMed;
(f) Y. Yoshida, K. Inoue and M. Kurmoo, Inorg. Chem., 2009, 48, 267 CrossRef CAS PubMed;
(g) Y. Inokuma, T. Arai and M. Fujita, Nat. Chem., 2010, 2, 780 CrossRef CAS PubMed;
(h) M. J. Manos, E. J. Kyprianidou, G. S. Papaefstathiou and A. J. Tasiopoulos, Inorg. Chem., 2012, 51, 6308 CrossRef CAS PubMed.
-
(a) M. Kurmoo, Chem. Soc. Rev., 2009, 38, 1353 RSC;
(b) P. Mahata, S. Natarajan, P. Panissod and M. Drillon, J. Am. Chem. Soc., 2009, 131, 10140 CrossRef CAS PubMed;
(c) G. Rogez, N. Virat and M. Drillon, Angew. Chem., Int. Ed., 2010, 49, 1921 CrossRef CAS PubMed;
(d) D. D. Sante, A. Stroppa, P. Jain and S. Picozzi, J. Am. Chem. Soc., 2013, 135, 18126 CrossRef PubMed;
(e) A. Stroppa, P. Barone, P. Jain, J. M. Perez-Mato and S. Picozzi, Adv. Mater., 2013, 25, 2284 CrossRef CAS PubMed;
(f) G. Beobide, W. Wang, O. Castillo, A. Luque, P. Román, G. Tagliabue, S. Galli and J. A. R. Navarro, Inorg. Chem., 2008, 5267 CrossRef CAS PubMed.
-
(a) Y. Takashima, V. M. Martinez, S. Furukawa, M. Kondo, S. Shimomura, H. Uehara, M. Nakahama, K. Sugimoto and S. Kitagawa, Nat. Commun., 2011, 2, 168 CrossRef PubMed;
(b) D. N. Dybtsev, H. Chun and K. Kim, Angew. Chem., Int. Ed., 2004, 43, 5033 CrossRef CAS PubMed.
-
(a) E. Y. Lee and M. P. Suh, Angew. Chem., Int. Ed., 2004, 43, 2798 CrossRef CAS PubMed;
(b) C. L. Chen, A. M. Goforth, M. D. Smith, C. Y. Su and H. C. zur Loye, Angew. Chem., Int. Ed., 2005, 44, 6673 CrossRef CAS PubMed;
(c) Q. Chu, D. C. Swenson and L. R. MacGillivray, Angew. Chem., Int. Ed., 2005, 44, 3569 CrossRef CAS PubMed;
(d) J. P. Ma, Y. B. Dong, R. Q. Huang, M. D. Smith and C. Y. Su, Inorg. Chem., 2005, 44, 6143 CrossRef CAS PubMed;
(e) J. Y. Lee, S. Y. Lee, W. Sim, K. M. Park, J. Kim and S. S. Lee, J. Am. Chem. Soc., 2008, 130, 6902 CrossRef CAS PubMed;
(f) Z. Wang and S. M. Cohen, J. Am. Chem. Soc., 2007, 129, 12368 CrossRef CAS PubMed.
-
(a) Y. Han, X. Li, L. Li, C. Ma, Z. Shen, Y. Song and X. You, Inorg. Chem., 2010, 49, 10781 CrossRef CAS PubMed;
(b) S. Dang, J.-H. Zhang, Z.-M. Sun and H. Zhang, Chem. Commun., 2012, 48, 11139 RSC;
(c) S. Dang, J.-H. Zhang and Z.-M. Sun, J. Mater. Chem., 2012, 22, 8868 RSC;
(d) W.-H. Zhu, Z.-M. Wang and S. Gao, Inorg. Chem., 2007, 46, 1337 CrossRef CAS PubMed.
-
(a) J. Rocha, L. D. Carlos, F. A. A. Paz and D. Ananias, Chem. Soc. Rev., 2011, 40, 926 RSC;
(b) D. Banerjee, Z. Hu and J. Li, Dalton Trans., 2014, 43, 10668 RSC;
(c) L. Zhang, Z. Kang, X. Xin and D. Sun, CrystEngComm, 2016, 18, 193 RSC;
(d) D. Zhao, Y. Cui, Y. Yang and G. Qian, CrystEngComm, 2016, 18, 3746 RSC.
-
(a) D. J. Tranchemontagne, J. L. Mendoza-Cortés, M. O'Keeffe and O. M. Yaghi, Chem. Soc. Rev., 2009, 38, 1257 RSC;
(b) L. Ma, C. Abney and W. Lin, Chem. Soc. Rev., 2009, 38, 1248 RSC;
(c) J. Lee, O. K. Farha, J. Roberts, K. A. Scheidt, S. T. Nguyen and J. T. Hupp, Chem. Soc. Rev., 2009, 38, 1450 RSC;
(d) M. D. Allendorf, C. A. Bauer, R. K. Bhakta and R. J. T. Houk, Chem. Soc. Rev., 2009, 38, 1330 RSC.
-
(a) H. Kajiro, A. Kondo, K. Kaneko and H. Kanoh, Int. J. Mol. Sci., 2010, 11, 3803 CrossRef CAS PubMed;
(b) L. Carlucci, G. Ciani, D. M. Proserpio, T. G. Mitina and V. A. Blatov, Chem. Rev., 2014, 114, 7557 CrossRef CAS PubMed;
(c) A. Schneemann, V. Bon, I. Schwedler, I. Senkovska, S. Kaskel and R. A. Fischer, Chem. Soc. Rev., 2014, 43, 6062 RSC.
-
(a) R. Kitaura, K. Seki, G. Akiyama and S. Kitagawa, Angew. Chem., Int. Ed., 2003, 42, 428 CrossRef CAS PubMed;
(b) A. Kondo, H. Noguchi, S. Ohnishi, H. Kajiro, A. Tohdoh, Y. Hattori, W. C. Xu, H. Tanaka, H. Kanoh and K. Kaneko, Nano Lett., 2006, 6, 2581 CrossRef CAS PubMed;
(c) A. Kondo, T. Nakagawa, H. Kajiro, A. Chinen, Y. Hattori, F. Okino, T. Ohba, K. Kaneko and H. Kanoh, Inorg. Chem., 2010, 49, 9247 CrossRef CAS PubMed;
(d) D. Xiao, H. Chen, D. Sun, J. He, S. Yan, J. Yang, X. Wang, R. Yuan and E. Wang, CrystEngComm, 2012, 14, 2849 RSC;
(e) A. Kondo, N. Kojima, H. Kajiro, H. Noguchi, Y. Hattori, F. Okino, K. Maeda, T. Ohba, K. Kaneko and H. Kanoh, J. Phys. Chem. C, 2012, 116, 4157 CrossRef CAS;
(f) Y. Jiang, J. Huang, B. Kasumaj, G. Jeschke, M. Hunger, T. Mallat and A. Baiker, J. Am. Chem. Soc., 2009, 131, 2058 CrossRef CAS PubMed.
-
(a) Y. B. Dong, Q. Zhang, L. L. Liu, J.-P. Ma, B. Tang and R. Q. Huang, J. Am. Chem. Soc., 2007, 129, 1514 CrossRef CAS PubMed;
(b) Y. F. Han, W. G. Jia, Y. J. Lin and G. X. Jin, Angew. Chem., Int. Ed., 2009, 34, 6234 CrossRef PubMed.
-
(a) J.-G. Haasnoot, Coord. Chem. Rev., 2000, 200–202, 131 CrossRef CAS;
(b) J. P. Zhang, S. Horike and S. Kitagawa, Angew. Chem., Int. Ed., 2007, 46, 889 CrossRef CAS PubMed.
-
(a) C. H. Lee, H. Y. Huang, Y. H. Liu, T. T. Luo, G. H. Lee, S. M. Peng, J. C. Jiang, I. Chao and K. L. Lu, Inorg. Chem., 2013, 52, 3962 CrossRef CAS PubMed;
(b) G.-M. Sun, F. Luo, Y.-M. Song, X.-Z. Tian, H.-X. Huang, Y. Zhu, Z.-J. Yuan, X.-F. Feng, M.-B. Luo, S.-J. Liu and W.-Y. Xu, Dalton Trans., 2012, 41, 11559 RSC.
- B.-C. Tzeng and T.-Y. Chang, Cryst. Growth Des., 2009, 9, 5343 CAS.
- Y. B. Dong, T. Sun, J. P. Ma, X. X. Zhao and R. Q. Huang, Inorg. Chem., 2006, 45, 10613 CrossRef CAS PubMed.
- J.-P. Ma, C.-W. Zhao, S.-Q. Wang, J.-P. Zhang, X. Niu and Y.-B. Dong, Chem. Commun., 2015, 51, 14586 RSC.
-
(a) B. Ding, L. Yi, Y. Wang, P. Cheng, D.-Z. Liao, S.-P. Yan and Z.-H. Jiang, Dalton Trans., 2006, 665 RSC;
(b) B. Ding, L. Yi, P. Cheng, D. Z. Liao and S. P. Yan, Inorg. Chem., 2006, 45, 5799 CrossRef CAS PubMed;
(c) B. Ding, Y.-Y. Liu, Y. Q. Huang, W. Shi, P. Cheng, D.-Z. Liao and S.-P. Yan, Cryst. Growth Des., 2009, 9, 593 CrossRef CAS;
(d) E.-C. Yang, H.-K. Zhao, B. Ding, X.-G. Wang and X.-J. Zhao, Cryst. Growth Des., 2007, 7, 2009 CrossRef CAS;
(e) B. Ding, P. Yang, Y.-Y. Liu, Y. Wang and G.-X. Du, CrystEngComm, 2013, 15, 2490 RSC;
(f) X.-X. Wu, Y.-Y. Wang, P. Yang, Y.-Y. Xu, J.-Z. Huo, B. Ding, Y. Wang and X.-G. Wang, Cryst. Growth Des., 2014, 14, 477 CrossRef CAS;
(g) J. Y. Liu, Q. Wang, L. J. Zhang, B. Yuan, Y. Y. Xu, X. Zhang, C. Y. Zhao, D. Wang, Y. Yuan, Y. Wang, B. Ding, X. J. Zhao and M. M. Yue, Inorg. Chem., 2014, 53, 5972 CrossRef CAS PubMed;
(h) Y. Wang, B. Yuan, Y. Y. Xu, X. G. Wang, B. Ding and X. J. Zhao, Chem.–Eur. J., 2015, 21, 2107 CrossRef CAS PubMed.
- G. Navarrete-Vázqueza, G. M. Molina-Salinasb, Z. V. Duarte-Fajardoa, J. Vargas-Villarrealb, S. Estrada-Sotoa, F. González-Salazarb, E. Hernández-Núñeza and S. Said-Fernándezb, Bioorg. Med. Chem., 2009, 46, 309 Search PubMed.
- G. M. Sheldrick, SHELXS-97, Program for X-ray Crystal Structure Solution, Göttingen University, Göttingen, Germany, 1997 Search PubMed.
- G. M. Sheldrick, SHELXL-97, Program for X-ray Crystal Structure Refinement, Göttingen University, Göttingen, Germany, 1997 Search PubMed.
-
(a) B. Salignac, S. Riedel, A. Dolbecq, F. Sécheresse and E. Cadot, J. Am. Chem. Soc., 2000, 122, 10381 CrossRef CAS;
(b) A. Hijazi, J. C. Kemmegne-Mbouguen, S. Floquet, J. Marrot, J. Fize, V. Artero and E. Cadot, Dalton Trans., 2013, 42, 4848 RSC.
-
(a) A. Barba-Bon, A. M. Costero, S. Gil, M. Parra, J. Soto, R. Martinez-Manezac and F. Sancenon, Chem. Commun., 2012, 48, 3000 RSC;
(b) G. S. Yang, M. N. Li, S. L. Li, Y. Q. Lan, W. W. He, X. L. Wang, J. S. Qin and Z. M. Su, J. Mater. Chem., 2012, 22, 17947 RSC.
- Z. G. Gu, Y. F. Xu, X. H. Zhou, J. L. Zuo and X. Z. You, Cryst. Growth Des., 2008, 8, 1306 CAS.
-
(a) Z. Wang, K. Hu, S. Gao and H. Kobayashi, Adv. Mater., 2010, 22, 1526 CrossRef CAS PubMed;
(b) G. Rogez, N. Viart and M. Drillon, Angew. Chem., Int. Ed., 2010, 49, 1921 CrossRef CAS PubMed;
(c) D. Di Sante, A. Stroppa, P. Jain and S. Picozzi, J. Am. Chem. Soc., 2013, 135, 18126 CrossRef CAS PubMed;
(d) M. Mączka, A. Ciupa, A. Gągor, A. Sieradzki, A. Pikul, B. Macalik and M. Drozd, Inorg. Chem., 2014, 53, 5260 CrossRef PubMed.
-
(a) X. Li, X. W. Wang and Y. H. Zhang, Inorg. Chem. Commun., 2008, 11, 832 CrossRef CAS;
(b) C. A. Bauer, T. V. Timofeeva, T. B. Settersten, B. D. Patterson, V. H. Liu, B. A. Simmons and M. D. Allendorf, J. Am. Chem. Soc., 2007, 129, 7136 CrossRef CAS PubMed.
-
(a) S. H. Rahaman, R. Ghosh, G. Mostafa and B. K. Ghosh, Inorg. Chem. Commun., 2005, 8, 1137 CrossRef CAS;
(b) Y. Lui and R. T. Tiekink, CrystEngComm, 2005, 7, 20 RSC;
(c) G. Singha, S. Girdhara and M. Garga, Synth. React. Inorg., Met.-Org., Nano-Met. Chem., 2013, 43, 1107–1111 CrossRef.
-
(a) J. M. Hao, B. Y. Yu, K. V. Hecke and G. H. Cui, CrystEngComm, 2015, 17, 2279 RSC;
(b) G. H. Cui, C. H. He, C. H. Jiao, J. C. Geng and V. A. Blatov, CrystEngComm, 2012, 14, 4210 RSC;
(c) X. X. Wang, B. Y. Yu, K. V. Hecke and G. H. Cui, RSC Adv., 2014, 4, 61281 RSC;
(d) L. Qin, J. Zheng, S. L. Xiao, X. H. Zheng and G. H. Cui, Inorg. Chem. Commun., 2013, 34, 71 CrossRef CAS;
(e) X. X. Wang, M. X. Zhang, B. Y. Yu, K. V. Hecke and G. H. Cui, Spectrochim. Acta, Part A, 2015, 139, 442 CrossRef CAS PubMed.
-
(a) C. M. Che, C. W. Wan, K. Y. Ho and Z. Y. Zhou, New J. Chem., 2001, 25, 63 RSC;
(b) J. H. Luo, M. C. Hong, R. H. Wang, R. Cao, L. Han and Z. Z. Lin, Eur. J. Inorg. Chem., 2003, 2705 CrossRef CAS;
(c) X. L. Wang, C. Qin, E. B. Wang and Z. M. Su, Chem.–Eur. J., 2006, 12, 2680 CrossRef CAS PubMed.
-
(a) C. X. Chen, Q. K. Liu, J. P. Ma and Y. B. Dong, J. Mater. Chem., 2012, 22, 9027 RSC;
(b) C. Fang, Q. K. Liu, J. P. Ma and Y. B. Dong, Inorg. Chem., 2012, 51, 3923 CrossRef CAS PubMed.
-
(a) Q. G. Zhai, X. Y. Wu, S. M. Chen, C. Z. Lu and W. B. Yang, Cryst. Growth Des., 2006, 6, 2126 CrossRef CAS;
(b) C. Ren, Y. N. Zhang, W. J. Shi, B. Liu, Y. Y. Wang and Q. Z. Shi, CrystEngComm, 2011, 13, 5179 RSC;
(c) J. Y. Gao, N. Wang, X. H. Xiong, C. J. Chen, W. P. Xie, X. R. Ran, Y. Long, S. T. Yue and Y. L. Liu, CrystEngComm, 2013, 15, 3261 RSC.
- K. L. Wong, G. L. Law, Y. Y. Yang and W. T. Wong, Adv. Mater., 2006, 18, 1051 CrossRef CAS.
-
(a) C. X. Chen, Q. K. Liu, J. P. Ma and Y. B. Dong, J. Mater. Chem., 2012, 22, 9027 RSC;
(b) C. Fang, Q. K. Liu, J. P. Ma and Y. B. Dong, Inorg. Chem., 2012, 51, 3923 CrossRef CAS PubMed.
-
(a) Q. G. Zhai, X. Y. Wu, S. M. Chen, C. Z. Lu and W. B. Yang, Cryst. Growth Des., 2006, 6, 2126 CrossRef CAS;
(b) C. Ren, Y. N. Zhang, W. J. Shi, B. Liu, Y. Y. Wang and Q. Z. Shi, CrystEngComm, 2011, 13, 5179 RSC;
(c) J. Y. Gao, N. Wang, X. H. Xiong, C. J. Chen, W. P. Xie, X. R. Ran, Y. Long, S. T. Yue and Y. L. Liu, CrystEngComm, 2013, 15, 3261 RSC.
- P. F. Shi, B. Zhao, G. Xiong, Y. L. Hou and P. Cheng, Chem. Commun., 2012, 48, 8231 RSC.
- K. L. Wong, G. L. Law, Y. Y. Yang and W. T. Wong, Adv. Mater., 2006, 18, 1051 CrossRef CAS.
- Z. Xie, L. Ma, K. E. deKrafft, A. Jin and W. Lin, J. Am. Chem. Soc., 2010, 132, 922 CrossRef CAS PubMed.
-
(a) W. S. Liu, T. Q. Jiao, Y. Z. Li, Q. Z. Liu, M. Y. Tan, H. Wang and L. F. Wang, J. Am. Chem. Soc., 2004, 126, 2280 CrossRef CAS PubMed;
(b) X. Yang, Z. Huang, J. Dang, C. L. Ho, G. Zhou and W. Y. Wong, Chem. Commun., 2013, 49, 4406 RSC;
(c) H. X. Zhao, L. Q. Liu, Z. D. Liu, Y. Wang, X. J. Zhao and C. Z. Huang, Chem. Commun., 2011, 47, 2604 RSC.
-
(a) Z. C. Hu, B. J. Deibert and J. Li, Chem. Soc. Rev., 2014, 43, 5815 RSC;
(b) H. Xu, C. S. Cao, X. M. Kang and B. Zhao, Dalton Trans., 2016, 45, 18003 RSC.
|
This journal is © The Royal Society of Chemistry 2017 |
Click here to see how this site uses Cookies. View our privacy policy here.