DOI:
10.1039/C6RA28069B
(Paper)
RSC Adv., 2017,
7, 13281-13288
Broad red-emission of Sr3Y2Ge3O12:Eu2+ garnet phosphors under blue excitation for warm WLED applications
Received
12th December 2016
, Accepted 20th February 2017
First published on 27th February 2017
Abstract
Eu2+ ions activated Sr3Y2Ge3O12 (SYGO) phosphors were synthesized by an efficient pechini-type sol–gel method. X-ray diffractometry, Fourier transform infrared spectrometry, field-emission transmission electron microscopy, and spectrofluorometry measurements were performed to characterize the prepared phosphor samples after annealing under thermal CO reducing atmosphere. The phosphors showed nearly spherical-shaped morphology and their phase was identified to be cubic with the space group la
d (230). Under 468 nm blue excitation, the SYGO:Eu2+ phosphors exhibited a broadband red emission at 612 nm due to the 4f65d1 → 4f7 electronic transition of Eu2+ ions. Temperature-dependent photoluminescence (PL) emission properties, PL decay profile and quantum yield were measured for the optimized SYGO:0.01 mol Eu2+ (SYGO:0.01Eu2+) red phosphor. Furthermore, a white light-emitting diode (WLED) device was also fabricated by blending the SYGO:0.01Eu2+ red phosphor with a commercially available Y3Al5O12:Ce3+ (YAG:Ce3+) yellow phosphor on a 452 nm blue LED chip under 30 mA forward-bias current. The fabricated YAG:Ce3+/SYGO:0.01Eu2+-based WLED device showed Commission International de I'eclairage chromaticity coordinates in the natural white region (0.3300, 0.3439) with good color rendering index and correlated color temperature of 85.81 and 5483 K, respectively. These results imply that the SYGO:0.01Eu2+ is a potential red phosphor to obtain the warm white light under blue excitations.
Introduction
In recent years, white light-emitting diodes (WLEDs) which are a so-called next-generation solid-state lighting source have been extensively employed in backlights, automobile headlights, and flashlights.1–5 Currently, these devices are gradually replacing traditional incandescent and florescent lamps owing to their bright features of high energy efficiency, long lifetime, high durability, cost-effectiveness and environmental compatibility characteristics.3,6–9 It is well known that commercial WLEDs are fabricated by a fairly broad spectral power distribution of a yellow-emitting Ce3+-doped Y3Al5O12 (YAG) phosphor dispersed in an epoxy resin and coated on a blue-emitting InGaN LED (450–470 nm) chip.10,11 When the YAG:Ce3+ phosphor is combined with the blue LED chip, the part of blue light is converted into yellow light via the phosphor and the remaining part of light stays with the yellow light, thus resulting in a cool white light. Therefore, the commercial YAG:Ce3+-based WLEDs generate only cool white light because of their lack of a red component in the spectral region, which exhibits low color-rendering index (CRI) (i.e., CRI < 80) as well as high color temperature (CCT).4,12,13 Due to the lack of the red spectral component, the usage of these WLEDs is often limited in industrial and commercial fields. To overcome these limitations, an additional complementary blue light excitable red-emitting phosphor is essential to improve the CRI value (>85) for obtaining the warm or natural white light. In general, Eu2+ ions activated metal oxide phosphors show broad emission bands in the blue, green or red spectral region under ultraviolet (UV) or visible wavelength excitations. Enormous research efforts have been extensively devoted to improve the broadband red emission by Eu2+ ions activated sulfide- and nitride-based phosphors such as BaLa2Si2S8:Eu2+,14 (Ca, Sr)S:Eu2+,15 CaAlSiN3:Eu2+,16 Sr2Si5N8:Eu2+,17 and SrAlSi4N7:Eu2+ (ref. 18) due to the dipole-allowed 4f → 5d electronic transitions. Nevertheless, these sulfide-based phosphors are thermally unstable and moisture-sensible.19,20 On the other hand, the synthesis of nitride-based phosphors often requires high temperature, high pressure, and vigorous inert gas conditions.7,12,21 Consequently, it is important to investigate the novel broadband red-emitting phosphors with an excitation band in the blue spectral region.
Garnet-type structures having the general formula of A3B2X3O12 (A = Ca, Sr, B = Y, Ga, In, Sc, Al and X = Si, Ge) have been receiving much attention in crystal chemistry owing to their good structural, physical, chemical, and thermal properties.2,22–24 In the compound formula, A, B, and X atoms are located in dodecahedral, octahedral, and tetrahedral sites and are surrounded by 8, 6, and 4 oxygen atoms to make a polyhedron.24,25 The rare-earth (RE) ions activated garnet-type silicate phosphors in a long run have been proved as effective host materials for luminescent properties. Currently, germanate garnet-type phosphors, such as Ca3Ga2Ge3O12:Cr3+/Bi3+,26 Mg3Y2Ge3O12:Ce3+,27 and Ca3Ln2Ge3O12:Eu3+ (Ln = Pr3+, Nd3+, Sm3+, Gd3+, Dy3+), have also been studied.28 This is because the Si and Ge belong to the same fourth elemental group and have the same outer electronic distribution in the periodic table. As per going through the literature, until now, there exist no other reports on Eu2+ ions activated broadband red-emitting Sr3Y2Ge3O12 phosphors. Thus, we selected the Sr3Y2Ge3O12 as a host material which belongs to the garnet family and possesses the cubic structure with a space group 1a
d (230).
Classically, RE ions activated metal oxide phosphors have been prepared by a conventional solid-state reaction method. This method needs high temperature over a long time and shows micrometer-sized particles with many impurities during the mechanical grinding process, which results in the lower luminescence intensity. However, to solve these problems and reduce the particle size, several finest wet-chemical methods such as hydrothermal,29 solvothermal,30 combustion,31 spray pyrolysis,32 sol–gel,33 co-precipitation,34 and pechini-type sol–gel35 were employed. Among them, the pechini-type sol–gel method is one of the best attractive and useful methods for the preparation of phosphor materials with definite morphology and is favorable to enhance the luminescent intensity. Pechini synthesis process consists of stable metal complex formation via the citric acid or salicylic acid and further proceeds the polyesterification with organic polymerizing agents such as ethylene glycol or polyethylene glycol, forming polymeric resins. The metal complexes can be tied up with the rigid polymeric network and decrease the segregation in between the metal ions, which assures the compositional homogeneity of all the reactants. Therefore, in this work, we reported the synthesis of Sr3Y2Ge3O12 phosphor samples with different Eu2+ ion concentrations by the pechini-type sol–gel method. The structural and morphological properties were examined by X-ray diffraction (XRD) patterns and field-emission transmission electron microscope (FE-TEM) images, respectively. A systematic study on the photoluminescence (PL) properties of Sr3Y2Ge3O12:Eu2+ phosphors was carried out in detail. Additionally, a WLED was implemented using a blue LED chip coated with the blend of the commercial YAG:Ce3+ phosphor and the Sr3Y2Ge3O12:Eu2+ red-emitting phosphor. It is found that the fabricated WLED exhibits higher CRI value for solid-state lighting and back-lighting applications.
Experimental procedure
A series of Sr3−3xEu3xY2Ge3O12 (x = 0.005, to 0.04 mol) phosphor samples were prepared by the pechini-type sol–gel method. The high-purity raw materials (Sigma-Aldrich Co.) such as strontium nitrate (Sr(NO3)2), yttrium nitrate hexahydrate (Y(NO3)3·6H2O), germanium oxide (GeO2), europium nitrate pentahydrate (Eu(NO3)3·5H2O), citric acid (HCO(COOH)(CH2COOH)2), and polyethylene glycol (PEG) ((C2H4O)n·H2O) were used according to the stoichiometric ratios. In the typical synthesis process, 3 mol of germanium oxide was mixed with 10 mL of concentrated nitric acid in a small beaker under magnetic stirring and it is diluted with 20 mL of de-ionized (DI) water to attain germanium nitrate solution, labeled as solution I. Secondly, solution II was prepared by adding 2 mol of yttrium nitrate, 3 − 3x mol of strontium nitrate, and 3x mol of europium nitrate in 180 mL of DI water. The solution I and solution II were stirred separately for 40 min. After that, the solution I was added slowly to the solution II and maintained the stirring for 30 min to attain the homogenous mixture. Later, 16 mol of citric acid (2
:
1 molar ratio of citric acid and total metal ions in the composition) was added as a chelating agent and stirred for 10 min. Finally, 1 g of PEG was prepended as a cross linking agent and the stirring continued for 1 h to get a complete homogeneous solution. Then, the beaker was covered with a polyethylene cap and heated on a hot plate at 85 °C for about 2 h under the stirring. Later on, the polyethylene cap and the magnetic bar were separated from the solution mixture and the solution was left at the same temperature for 8 h. The solution evaporated slowly, producing the homogenous brownish wet gel. After drying the wet gel in an oven at 120 °C for 24 h, a white colored porous solid matrix called as xerogel was formed. The xerogel was ground, heated at 1200 °C for 8 h in the air atmosphere, and again annealed at 1000 °C (rising temperature of 2 °C per min) for 4 h under thermal CO reducing atmosphere. The obtained phosphor samples were employed to assess the further characterization.
Sample characterization
The XRD pattern and Fourier transform infrared (FTIR) spectrum of the Sr3Y2Ge3O12:Eu2+ (hereafter referred as SYGO:Eu2+) phosphor samples were recorded on a Mac Science M18XHF-SRA X-ray powder diffractometer and a Thermo Nicolet-5700 FTIR spectrophotometer with the KBr pellet method, respectively. The morphological properties were measured by using a FE-TEM (JEOL JEM-2100F). The PL spectra were studied by using a fluorescence spectrometer (FluoroMate FS-2, Scinco, South Korea) and the lifetime was evaluated on a Photon Technology International (PTI, USA) phosphorimeter with a Xe flash lamp of 25 W power. Thermal PL properties were recorded on the fluorescence spectrometer (FluoroMate FS-2, Scinco, South Korea) equipped with a thermocouple in the temperature range of 30–210 °C (NOVA ST540). In addition, the quantum yield measurement was performed by using a Hamamatsu Photonics C9920-02 system with an integrating sphere.
Results and discussion
XRD, FE-TEM and FTIR analyses of SYGO:0.01Eu2+ phosphor
Fig. 1(a) shows the XRD patterns of the SYGO host lattice, SYGO:0.01Eu3+ phosphor and SYGO:0.01Eu2+ phosphor after annealing in air and thermal CO reducing atmosphere, respectively. It can be observed that all the diffraction peaks of the three phosphor samples were well consistent with the available standard JCPDS # 85-2410 card. This is attributed to the pure cubic crystal phase of garnet with a space group la
d (230). The pure phase form indicates that the Eu2+ ions are completely dissolved into the Sr2+ sites of the SYGO host lattice and did not exhibit any new significant phase in the present synthesis process. The full width at half maximum (FWHM) of the strongest XRD peak is utilized to determine the crystallite/grain sizes of the SYGO:0.01Eu2+ phosphor by the well-known Scherrer formula of Dhkl = kλ/β
cos
θ, where D is the average crystallite size, k (0.9) is a shape factor, λ is the X-ray wavelength which is 1.5406 Å, β is the FWHM, and θ is the corresponding Bragg's diffraction angle of the observed peaks. Thus, the calculated average crystallite size of the SYGO:0.01Eu2+ phosphor is 97 nm. According to the data in the JCPDS # 85-2410 card, the SYGO garnet crystal structure belongs to the cubic system with a space group la
d (230), showing the body-centered cubic symmetry in a unit cell lattice. The SYGO garnet is iso-structural to the Ca3Y2Ge3O12 garnet phase and the ideal unit cell crystal structure was drawn using a Diamond software as shown in Fig. 1(b). From the crystal structure, the Sr2+, Y3+, and Ge4+ ions were surrounded by 8, 6, and 4 oxygen anions, leading to the dodecahedron, octahedron, and tetrahedron, respectively. On account of the ionic radii or valence state, the Eu2+ ions are expected to occupy the Sr2+ sites in the SYGO crystal structure.
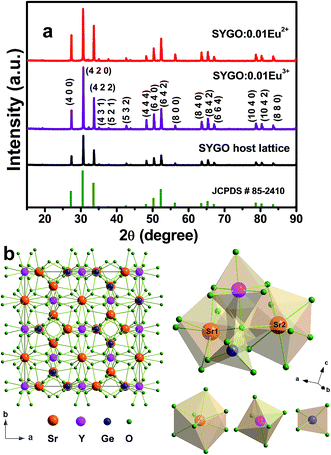 |
| Fig. 1 (a) XRD patterns of the SYGO host lattice, SYGO:0.01Eu3+ phosphor, and SYGO:0.01Eu2+ phosphor after annealing in air at 1200 °C and thermal CO reducing atmosphere at 1000 °C, respectively and (b) crystal structure drawn using the Diamond software. | |
Fig. 2(a) shows the FE-TEM image of the SYGO:0.01Eu2+ phosphor after annealing under the CO reducing atmosphere. The FE-TEM image revealed the inhomogeneous spherical-shaped morphology with non-identical particle sizes. The spherical-shaped morphology would be favorable to produce more stable efficient white light for solid-state lighting applications.36,37 It is observed that the particle size (>200 nm) is different from the calculated crystallite/grain size from the XRD (97 nm) because a particle may be composed of one or more crystallites.38 Fig. 2(b) and (c) represents the high-resolution TEM (HRTEM) image and the corresponding selected area electron diffraction (SAED) pattern of the single particle of SYGO:0.01Eu2+ phosphor. The HRTEM image showed a d-spacing of 3.4 Å, corresponding to the (321) plane of the phosphor sample, and it well coincided with the cubic phase of the garnet in the XRD. From the SAED pattern, it is clear that the diffraction spots were in doted pattern which confirms the single crystalline nature of the SYGO:0.01Eu2+ phosphor. Fig. 2(d) shows the FTIR spectrum of the as-synthesized SYGO:0.01Eu2+ phosphor sample. This spectrum exhibited absorption peaks at 3306 and 1616 cm−1 which can be attributed to the stretching and bending vibrations of the O–H bonds due to absorption of water on the surface of the phosphor sample.38,39 The sharp band at 1405 cm−1 can be attributed to the bending mode of O–H bonds.40,41 It can be also observed that strong absorption bands at 934, 792, and 711 cm−1 are related to the asymmetric vibration of Ge–O bonds.42 The sharp peaks at 562 and 482 cm−1 represent the characteristic vibration modes of Ge–O–Ge bonds.40,42
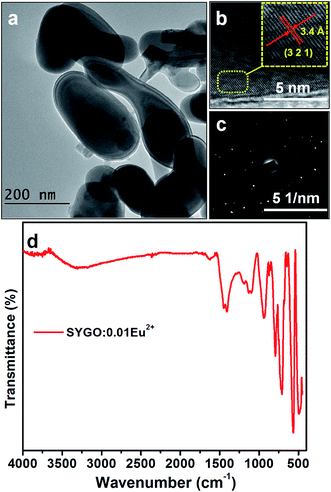 |
| Fig. 2 (a) FE-TEM image, (b) HRTEM image, (c) SAED pattern, and (d) FTIR spectrum of the SYGO:0.01Eu2+ phosphor. | |
PL properties and quantum yield of SYGO:Eu2+ phosphors
Fig. 3 shows the PL excitation (PLE) and PL emission spectra of the SYGO:0.01Eu2+ phosphor sample. The PLE spectrum consists of two broad bands which cover the wavelength from UV to visible regions (200 to 600 nm) when it was monitored at 612 nm emission wavelength. The first broadband ranging from 220 to 320 nm occurred with the band maxima at 270 nm due to the electronic transition between the valance and conduction bands of the host lattice. Another broadband was located in the wavelength range of 375 to 600 nm with the band maxima at 468 nm and it also consists of several sub-bands centered at 440, 452, 484, and 493 nm which is ascribed to the 4f7 → 4f65d1 electronic transition of Eu2+ ions. Under 468 nm blue excitation, the PL emission of the SYGO:0.01Eu2+ phosphor exhibited a single and symmetric broad red emission band localized between 540 to 750 nm with the band maxima at 612 nm corresponding to the 4f65d1 → 4f7 transition of Eu2+ ions. The broad red emission band also consisted of Eu3+ emission peak in the region of 650 to 670 nm with the band maxima at 660 nm due to the 5D0 → 7F3 electronic transition. Under 270 nm excitation, a similar red emission band occurred significantly with lower intensity. The broad red emission band of the SYGO:0.01Eu2+ phosphor showed higher emission intensity at 468 nm excitation wavelength with a FWHM value of 80 nm. Consequently, the prepared SYGO:0.01Eu2+ phosphor revealed broadband red emission under UV and visible wavelength excitations.
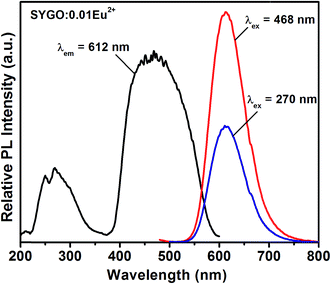 |
| Fig. 3 PLE (λem = 612 nm) and PL emission (λex = 270 and 468 nm) spectra of the SYGO:0.01Eu2+ phosphor. | |
In order to find an optimum concentration, a series of SYGO:Eu2+ phosphor samples with different concentrations of Eu2+ ions were prepared. The PL emission spectra taken at an excitation wavelength of 468 nm are shown in Fig. 4(a). The broadband red emission intensity was increased with increasing the Eu2+ ion concentration up to 0.01 mol. The emission intensity decreased with further increasing the Eu2+ ion concentration (0.02, 0.03 and 0.04 mol) due to the concentration quenching phenomena and it is ascribed to the non-radiative energy transfer between the adjacent Eu2+ ions. The non-radiative energy process exists due to the radiation reabsorption, electric multipolar interactions or exchange interactions. It is obvious that the energy transfer between the Eu2+ ions of SYGO phosphors should not be dominated by the exchange interaction due to the allowed 4f7 → 4f65d1 electronic transition. The radiative reabsorption occurs due to the spectral overlap of PLE and PL emission spectra, which is not fully accountable for non-radiative energy transfer between the Eu2+ ions in the present study. Thus, the energy transfer between the Eu2+ ions should be controlled by electric multipolar interactions. Based on Dexter theory,43,44 the concentration quenching mechanism of interaction between the two adjacent Eu2+ ions is calculated by the following expression:
|
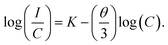 | (1) |
Here,
I and
K are the luminescence intensity and activator constant,
C is the optimum substituted mole fraction of the Eu
2+ ions (0.01 mol) and
θ is the electric multipolar parameter. The
θ = 3, 6, 8, and 10 correspond to the exchange coupling, dipole–dipole, dipole–quadrupole, and quadrupole–quadrupole interactions, respectively.
Fig. 4(b) shows the linear relationship between the log(
C)
versus log(
I/
C) of Eu
2+ ions. This curve was well fitted by a straight line with a slope of −1.35. The calculated value of
θ is 9.6, which is close to 10. Therefore, the quadrupole–quadrupole interactions are responsible for non-radiative energy transfer mechanism between the Eu
2+ ions.
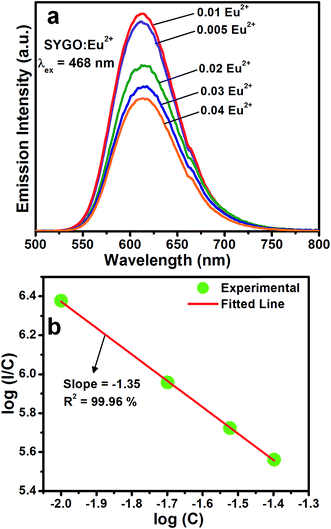 |
| Fig. 4 (a) PL emission spectra of SYGO:Eu2+ phosphors as a function of Eu2+ ion concentration at 468 nm excitation wavelength. (b) Relationship of log(C) versus log(I/C) for SYGO:Eu2+ phosphors. | |
To estimate the lifetime of the optimized SYGO:0.01Eu2+ phosphor sample, the luminescence decay curve was measured under 612 nm emission and 468 nm excitation wavelengths as shown in Fig. 5(a). The decay profile was well fitted to a second-order exponential function as follows:45
|
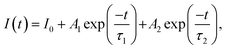 | (2) |
where
I0 and
I(
t) are the luminescence intensities at initial time
t = 0 and time
t, respectively.
A1 and
A2 are constants and
τ1 and
τ2 are the slow decay times for exponential functions. Thus, the average lifetime can be determined by the following expression:
|
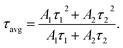 | (3) |
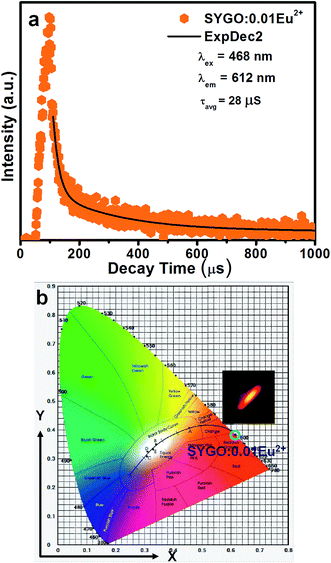 |
| Fig. 5 (a) Decay curve and (b) CIE chromaticity diagram with the corresponding luminescent photograph of the optimized SYGO:0.01Eu2+ phosphor sample. | |
Accordingly, the average lifetime value was found to be around 28 μs. The double exponential curve fitting is due to the existence of the two Sr luminescent centers as explored in the SYGO crystal structure. The quantum efficiency (η) was measured by the integrated sphere method for the optimized SYGO:0.01Eu2+ phosphor sample. Under the present synthesis condition, the obtained η value for the SYGO:0.01Eu2+ phosphor sample at 612 nm emission and 468 nm excitation wavelengths was ∼28.3%. The Commission International de I'eclairage (CIE) chromaticity coordinate values were calculated by taking the emission wavelength range from 500 to 800 nm of the SYGO:0.01Eu2+ phosphor as shown in Fig. 5(b). The obtained CIE values (0.6146, 0.3837) were found in the reddish-orange region and the matching luminescent photograph of the phosphor is shown in the inset of Fig. 5(b).
Thermal stability and warm WLED fabrication process of SYGO:0.01Eu2+ phosphor
Thermal stability is one of the scientific properties of the synthesized phosphor materials due to their considerable impact on the light output, chromaticity, and CRI in practical applications of high-power WLEDs. Fig. 6(a) represents the temperature-dependent PL emission spectra of the SYGO:0.01Eu2+ phosphor sample excited at 468 nm in the temperature range of 30 to 210 °C at an interval of 20 °C. From the PL emission spectra, it is clear that the PL emission intensity of the SYGO:0.01Eu2+ phosphor gradually decreased with increasing the temperature. The PL emission intensity of the sample was maintained to approximately 52% at 130 °C with respect to the initial intensity at 30 °C. The loss of the reaming 48% emission intensity is due to the thermal quenching phenomena in the SYGO:0.01Eu2+ phosphor. In this manner, the impressive thermal stability of the phosphor strongly gives aid to the high-power WLED applications. The thermal quenching behavior occurs due to the non-radiative transition from the 4f65d1 excited state to the 4f7 ground state of Eu2+ ions.46 Furthermore, the red emission spectrum of the SYGO:0.01Eu2+ phosphor was blue-shifted above 15 nm with increasing the temperature as shown in Fig. 6(a). The obtained blue-shift is due to the thermally active phonon-assisted excitation from a lower energy emission band to a high-energy emission band of Eu2+ ions in the configuration coordinate diagram.46,47 The activation energy (Ea) for the thermal quenching of the SYGO:0.01Eu2+ phosphor was calculated by the following Arrhenius equation:48,49 |
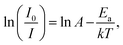 | (4) |
where I0 and I are the emission intensities of the phosphor at room temperature and applied temperature, respectively. A is an Arrhenius constant, T is the temperature, and k is a Boltzmann's constant (8.617 × 10−5 eV K−1). Fig. 6(b) shows the plots of ln(I0/I − 1) versus 1/kT. The experimental data were well fitted by a linear fit with a slope of −0.175, indicating the thermal quenching phenomena accompanied with the Arrhenius activation model. From the Fig. 6(b), the calculated Ea value can be inferred to be 0.175 eV.
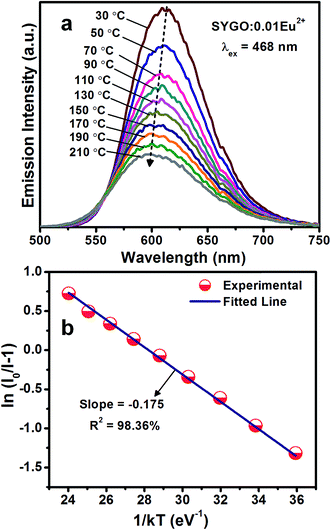 |
| Fig. 6 (a) Temperature-dependent PL emission spectra of the SYGO:0.01Eu2+ phosphor at 468 nm excitation wavelength and (b) the corresponding plot of ln(I0/I − 1) versus 1/kT. | |
Fig. 7 shows the comparative PLE and PL emission spectra of the as-synthesized SYGO:0.01Eu2+ red-emitting phosphor with the commercially available YAG:Ce3+ phosphor under their corresponding emission and excitation wavelengths, respectively. From the spectra, it is clear that the excitation and emission bands of the SYGO:0.01Eu2+ phosphor were strongly overlapped with the emission band of YAG:Ce3+ phosphor and, in particular, the excitation peaks at 452 and 468 nm of the both phosphors were well matched with each other. Thus, the prepared red-emitting phosphors with the excitation wavelengths of 445–475 nm strongly serve as the excitation source to compensate the red emission deficiency of the YAG:Ce3+ phosphor and the excitation wavelength region also perfectly matched with the InGaN blue LED excitation. Hence, by mixing the appropriative amounts of the SYGO:0.01Eu2+ and YAG:Ce3+ phosphors with the blue LED chip, a natural white light was produced for indoor illumination and back-lighting sources.
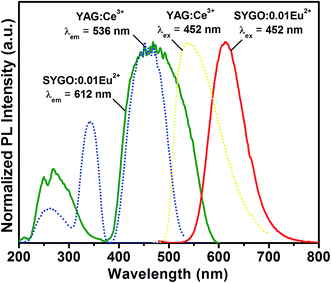 |
| Fig. 7 Comparative PLE and PL emission spectra of the SYGO:0.01Eu2+ and commercial YAG:Ce3+ phosphors. | |
In order to explore the potentiality of the prepared SYGO:0.01Eu2+ phosphor, a WLED package was done by adding this to the YAG:Ce3+ phosphor on a 452 nm InGaN blue LED chip. Fig. 8(a) represents the electroluminescence (EL) spectrum of the WLED made with the commercial YAG:Ce3+ phosphor under a forward-bias current of 30 mA. The EL spectrum exhibited a broadband emission with the band maxima at about 534 nm due to the corresponding 2D3/2 → 2F5/2 and 2F7/2 electronic transitions of Ce3+ ions. The CRI and CCT values of the fabricated device were 75.5 and 9314 K, respectively. The CIE values were found to be (0.2771, 0.3101). Thus, the fabricated YAG:Ce3+-based WLED emitted a cool white light due to the absence of red-emitting phosphors as shown in the inset of Fig. 8(a). To obtain warm white light, a WLED device was fabricated using the similar 452 nm blue LED chip with a appropriative blend of the YAG:Ce3+ phosphor and the SYGO:0.01Eu2+ red-emitting phosphor, driven by a 30 mA forward-bias current as shown in Fig. 8(b). The fabricated WLED exhibited the lower CCT (5483 K) and higher CRI (85.81) values compared with the fabricated YAG:Ce3+-based WLED. The obtained CRI and CCT values are excellent prerequisite specifications for illuminating the natural white light. Thus, an obvious emission in the red wavelength region was enhanced by the incorporation of the red-emitting phosphor and the variation in the EL spectrum was clearly observed for the fabricated YAG:Ce3+ and YAG:Ce3+/SYGO:0.01Eu2+ WLED devices. The inset of Fig. 8(b) shows the warm white-light digital photograph of the fabricated WLED device under the same forward-bias current. It is further noticed that the CIE chromaticity coordinates of the YAG:Ce3+-based WLED in the cool white region (0.2771, 0.3101) were well tuned to the natural white region (0.3300, 0.3439) when mixing the red-emitting phosphor as shown in Fig. 8(c). The inset of Fig. 8(c) represents the schematic diagram of the packaged WLED devices with the InGaN blue LED chips using the YAG:Ce3+ phosphor and the mixed YAG:Ce3+ and red-emitting phosphors. Consequently, the obtained results demonstrate that the SYGO:0.01Eu2+ is a potential red-emitting phosphor to get the warm white light in solid-state lighting and optical display applications.
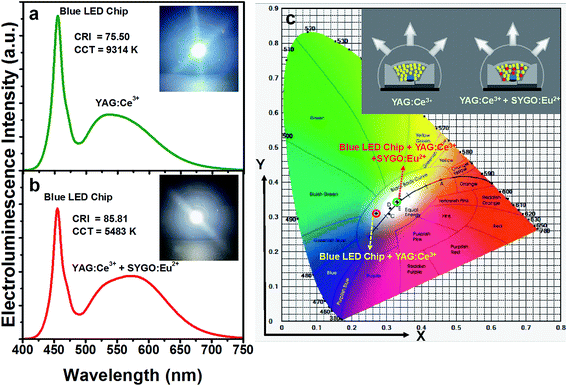 |
| Fig. 8 EL spectra of (a) the commercial YAG:Ce3+ phosphor, (b) the mixed YAG:Ce3+ and SYGO:0.01Eu2+ red-emitting phosphors coated on the 452 nm blue LED chips under a forward-bias current of 30 mA (insets show the corresponding digital photograph images of the packaged WLEDs), and (c) CIE chromaticity coordinates of the YAG:Ce3+- and YAG:Ce3+/SYGO:0.01Eu2+-based WLEDs (inset shows the schematic diagram of the packaged WLEDs). | |
Conclusions
In summary, we have successfully synthesized the SYGO:Eu2+ red-emitting phosphor samples by the pechini-type sol–gel method. The XRD patterns confirmed the cubic phase form of the garnet phosphor with a space group la
d (230). The FE-TEM image revealed the spherical-shaped morphology after annealing under the CO reduced atmosphere. The PL emission spectra of the SYGO:Eu2+ phosphors showed broad symmetric red band in the wavelength range of 540 to 750 nm with the band maxima at 612 nm due to the allowed 4f65d1 → 4f7 electronic transition of Eu2+ ions at 468 nm excitation wavelength. The optimum Eu2+ ion concentration in SYGO phosphors was found to be 0.01 mol and the quadrupole–quadrupole interactions were involved in the concentration quenching mechanism of Eu2+ ions. The temperature-dependent PL emission spectra were explored for the optimized SYGO:0.01Eu2+ phosphor, exhibiting good thermal stability with the activation energy of 0.175 eV. The η value of SYGO:0.01Eu2+ phosphor at 612 nm emission and 468 nm excitation wavelengths was about 28.3%. Moreover, for the purpose of comparison, the individual commercial YAG:Ce3+-based WLED and the mixed YAG:Ce3+ + red-emitting SYGO:0.01Eu2+ phosphors-based WLED were fabricated under a forward-bias current of 30 mA. The CRI and CCT values of the YAG:Ce3+/SYGO:0.01Eu2+-based WLED are very much improved and close to a warm white light. Therefore, from all these results, the broadband SYGO:0.01Eu2+ red-emitting phosphors are expected to be a promising candidate for the applications of solid-state lighting and back-lighting systems.
Acknowledgements
This work was supported by the National Research Foundation of Korea (NRF) Grant funded by the Korea government (MSIP) (No. 2015R1A5A1037656).
References
- J. Zhong, W. Zhuang, X. Xing, R. Liu, Y. Li, Y. Liu and Y. Hu, J. Phys. Chem. C, 2015, 119, 5562–5569 CAS.
- Y. F. Wu, Y. H. Chan, Y. T. Nien and I. G. Chen, J. Am. Ceram. Soc., 2013, 96, 234–240 CrossRef CAS.
- Z. Xu, Z. Xia, B. Lei and Q. Liu, J. Mater. Chem. C, 2016, 4, 9711–9716 RSC.
- Y. Jin, M.-H. Fang, M. Grinberg, S. Mahlik, T. Lesniewski, M. G. Brik, G.-Y. Luo, J. G. Lin and R.-S. Liu, ACS Appl. Mater. Interfaces, 2016, 8, 11194–11203 CAS.
- H. L. Li, R. J. Xie, N. Hirosaki, T. Takeda and G. H. Zhou, Int. J. Appl. Ceram. Technol., 2009, 6, 459–464 CrossRef CAS.
- A. Kitai, Lumin. Mater. Appl., John Wiley & Sons, 2008 Search PubMed.
- D. Deng, H. Yu, Y. Li, Y. Hua, G. Jia, S. Zhao, H. Wang, L. Huang, Y. Li, C. Li and S. Xu, J. Mater. Chem. C, 2013, 1, 3194–3199 RSC.
- W. B. Im, Y.-I. Kim, N. N. Fellows, H. Masui, G. Hirata, S. P. DenBaars and R. Seshadri, Appl. Phys. Lett., 2008, 93, 1905 CrossRef.
- Z. Jiang and Y. Wang, Electrochem. Solid-State Lett., 2010, 13, J68–J70 CrossRef CAS.
- W.-j. Ding, M. Zhang, R.-j. Yu, J. wang and Q. su, Acta Sci. Nat. Univ. Sunyatseni, 2008, 4, 014 Search PubMed.
- X. Ding, Q. Wang and Y. Wang, Phys. Chem. Chem. Phys., 2016, 18, 8088–8097 RSC.
- J. Ruan, R. J. Xie, N. Hirosaki and T. Takeda, J. Am. Ceram. Soc., 2011, 94, 536–542 CrossRef CAS.
- T. Hussain, L. Zhong, M. Danesh, H. Ye, Z. Liang, D. Xiao, C.-W. Qiu, C. Lou, L. Chi and L. Jiang, Nanoscale, 2015, 7, 10350–10356 RSC.
- S.-P. Lee, T.-S. Chan and T.-M. Chen, ACS Appl. Mater. Interfaces, 2014, 7, 40–44 Search PubMed.
- Y. Hu, W. Zhuang, H. Ye, S. Zhang, Y. Fang and X. Huang, J. Lumin., 2005, 111, 139–145 CrossRef CAS.
- K. Uheda, N. Hirosaki, Y. Yamamoto, A. Naito, T. Nakajima and H. Yamamoto, Electrochem. Solid-State Lett., 2006, 9, H22–H25 CrossRef CAS.
- R.-J. Xie, N. Hirosaki, T. Suehiro, F.-F. Xu and M. Mitomo, Chem. Mater., 2006, 18, 5578–5583 CrossRef CAS.
- C. Hecht, F. Stadler, P. Schmidt and W. Schnick, Z. Anorg. Allg. Chem., 2008, 634, 2044 CrossRef.
- S. S. Manoharan, S. Goyal, M. L. Rao, M. S. Nair and A. Pradhan, Mater. Res. Bull., 2001, 36, 1039–1047 CrossRef CAS.
- B. L. Abrams, W. Roos, P. H. Holloway and H. C. Swart, Surf. Sci., 2000, 451, 174–181 CrossRef CAS.
- X. Piao, T. Horikawa, H. Hanzawa and K.-i. Machida, Appl. Phys. Lett., 2006, 88, 161908 CrossRef.
- O. Lipina, L. Surat, M. Melkozerova, A. Tyutyunnik, I. Leonidov and V. Zubkov, Opt. Spectrosc., 2014, 116, 695–699 CrossRef CAS.
- Y. Liu, J. Hao, W. Zhuang and Y. Hu, J. Phys. D: Appl. Phys., 2009, 42, 245102 CrossRef.
- D. Chen, Y. Chen, H. Lu and Z. Ji, Inorg. Chem., 2014, 53, 8638–8645 CrossRef CAS PubMed.
- D. Pasiński, E. Zych and J. Sokolnicki, J. Alloys Compd., 2015, 653, 636–642 CrossRef.
- C. Liu, Z. Xia, M. S. Molokeev and Q. Liu, J. Am. Ceram. Soc., 2015, 98, 1870–1876 CrossRef CAS.
- Z. Jiang, Y. Wang and L. Wang, J. Electrochem. Soc., 2010, 157, J155–J158 CrossRef CAS.
- F. Piccinelli, A. Lausi and M. Bettinelli, J. Solid State Chem., 2013, 205, 190–196 CrossRef CAS.
- B. Yan and J.-H. Wu, Mater. Chem. Phys., 2009, 116, 67–71 CrossRef CAS.
- X. Li, H. Liu, J. Wang, H. Cui, S. Yang and I. Boughton, J. Phys. Chem. Solids, 2005, 66, 201–205 CrossRef CAS.
- T. Peng, H. Yang, X. Pu, B. Hu, Z. Jiang and C. Yan, Mater. Lett., 2004, 58, 352–356 CrossRef CAS.
- Y. Shimomura and N. Kijima, J. Electrochem. Soc., 2004, 151, H192–H197 CrossRef CAS.
- I.-C. Chen and T.-M. Chen, J. Mater. Res., 2001, 16, 644–651 CrossRef CAS.
- J. H. Zeng, J. Su, Z. H. Li, R. X. Yan and Y. D. Li, Adv. Mater., 2005, 17, 2119–2123 CrossRef CAS.
- M. Pang, X. Liu and J. Lin, J. Mater. Res., 2005, 20, 2676–2681 CrossRef CAS.
- G. S. R. Raju, H. C. Jung, J. Y. Park, C. Kanamadi, B. K. Moon, J. H. Jeong, S.-M. Son and J. H. Kim, J. Alloys Compd., 2009, 481, 730–734 CrossRef CAS.
- D. Geng, G. Li, M. Shang, C. Peng, Y. Zhang, Z. Cheng and J. Lin, Dalton Trans., 2012, 41, 3078–3086 RSC.
- S. K. Hussain and J. S. Yu, J. Lumin., 2016, 175, 100–105 CrossRef CAS.
- M. Runowski, T. Grzyb, A. Zep, P. Krzyczkowska, E. Gorecka, M. Giersig and S. Lis, RSC Adv., 2014, 4, 46305–46312 RSC.
- Y. Cao, G. Zhu and Y. Wang, RSC Adv., 2015, 5, 65710–65718 RSC.
- L. K. Bharat and J. S. Yu, J. Nanosci. Nanotechnol., 2013, 13, 8239–8244 CrossRef CAS PubMed.
- V. Zubkov, I. Leonidov, A. Tyutyunnik, N. Tarakina, I. Baklanova, L. Perelyaeva and L. Surat, Phys. Solid State, 2008, 50, 1699–1706 CrossRef CAS.
- D. L. Dexter, J. Chem. Phys., 1953, 21, 836–850 CrossRef CAS.
- B. Wang, H. Lin, J. Xu, H. Chen and Y. Wang, ACS Appl. Mater. Interfaces, 2014, 6, 22905–22913 CAS.
- M. P. Saradhi and U. Varadaraju, Chem. Mater., 2006, 18, 5267–5272 CrossRef CAS.
- L. Chen, C.-C. Lin, C.-W. Yeh and R.-S. Liu, Materials, 2010, 3, 2172–2195 CrossRef CAS.
- C. C. Lin and R.-S. Liu, J. Phys. Chem. Lett., 2011, 2, 1268–1277 CrossRef CAS PubMed.
- N. Zhang, C. Guo, J. Zheng, X. Su and J. Zhao, J. Mater. Chem. C, 2014, 2, 3988–3994 RSC.
- J. Chen, N. Zhang, C. Guo, F. Pan, X. Zhou, H. Suo, X. Zhao and E. M. Goldys, ACS Appl. Mater. Interfaces, 2016, 8, 20856–20864 CAS.
|
This journal is © The Royal Society of Chemistry 2017 |