DOI:
10.1039/C6RA27773J
(Paper)
RSC Adv., 2017,
7, 20591-20596
A diarylethene-based “on–off–on” fluorescence sensor for the sequential recognition of mercury and cysteine†
Received
5th December 2016
, Accepted 29th March 2017
First published on 10th April 2017
Abstract
A novel photochromic diarylethene with a quinoline unit was synthesized with multi-controllable fluorescence switching properties, which could be induced by light, mercury (Hg2+) and cysteine (Cys). Because the diarylethene displayed obvious fluorescence quenching with Hg2+ and the fluorescence was recovered to its original state evidently with Cys, it could sequentially recognize Hg2+ and Cys. Additionally, a logic circuit was constructed with the fluorescence intensity at 468 nm as output, and the combined stimuli of light and chemicals as inputs.
Introduction
As a hazardous chemical in the environment from a variety of natural and anthropogenic sources,1,2 the mercury ion (Hg2+) is a caustic and carcinogenic material with high cellular toxicity3 because it accumulates in the human body and a very small amount can cause serious diseases, such as cardiovascular disease, serious cognitive and motion disorders, Minamata disease and coronary heart disease.4–9 Therefore, monitoring Hg2+ in the environment has received increasing interest. Currently, various techniques and protocols, such as atomic absorption spectrometry, inductively coupled plasma, atomic emission spectroscopy, and capillary electrophoresis have been well developed and utilized to monitor Hg2+.10–13 However, most of these methods are still limited by complex instruments and complicated procedures with high cost, or low sensitivity and low selectivity. However, fluorescent sensors are very simple and sensitive in the detection of metal ions or harmful pollutants in the environment. Therefore, considerable efforts have been made to develop fluorescent molecular chemosensors for Hg2+.14–22
As a small molecular weight biothiol, cysteine (Cys) plays a prominent role in various critical biological systems, such as metabolic processes, biocatalysis and detoxifications of xenobiotics.23–27 However, Hg2+ ion can bond to Cys through Hg–S to cause many health problems,28–30 such as slowed growth in children, liver damage, loss of muscle and fat, skin lesions and weakness.31 On other hand, high levels of Cys in living systems also cause many human diseases, including developmental retardation, cardiovascular, osteoporosis, Alzheimer's disease, etc.32 Therefore, it is very important to detect Cys and Hg2+ in biological systems. Up to present, detection of Hg2+ and Cys was mainly based on photochromic materials with dual functionalities.33–36 Among these various photochromic compounds, diarylethenes are one of the most promising photo-switchable molecules with excellent photochemical reactivity, thermal stability, fatigue resistance37–40 and fluorescence.41–43 For example, Tian and his co-workers have reported two photochromic diarylethene-based chemosensors with excellent optical properties to distinguish Hg2+ in acetonitrile.44 We also synthesized a photochromic diarylethene with a formyl group. Because of the interaction between the formyl group of its ring-closed isomer with Cys, there was a notable change in its absorption spectrum with an evident color change from blue to pale yellow if Cys was added.45 However, all of these reports were focused on recognizing Hg2+ and Cys individually.
In this study, we designed and synthesized a new photochromic diarylethene with a quinoline unit, which could sequentially recognize Hg2+ and Cys efficiently. Compared with previously reported results, this method was more feasible, economic, convenient and significant. The fluorescence of the diarylethene was quenched in the presence of Hg2+ in tetrahydrofuran. When a certain amount of Cys was subsequently added, the fluorescence was enhanced and restored to almost the intensity before quenching.
Experimental
General methods
NMR spectra were recorded on Bruker AV400 (400 MHz) spectrometer with CDCl3 as the solvent and tetramethylsilane (TMS) as the internal standard. Mass spectra were measured on a Bruker AmaZon SL Ion Trap Mass spectrometer. IR spectra were recorded on a Bruker Vertex-70 spectrometer. Melting point was measured on a WRS-1B melting point apparatus. The absorption spectra were measured on an Agilent 8453 UV/Vis spectrophotometer. Photoirradiation was carried out with an SHG-200 UV lamp, CX-21 ultraviolet fluorescence analysis cabinet and a BMH-250 visible lamp. Light of appropriate wavelength was isolated through different light filters. Fluorescence spectra were recorded on a Hitachi F-4600 fluorescence spectrophotometer. The fluorescence quantum yield was measured on an Absolute PL Quantum Yield Spectrometer QY C11347-11. All inorganic salts (Hg(ClO4)2·6H2O, Cd(NO3)2·4H2O, Zn(NO3)2·6H2O, Mg(NO3)2·6H2O, Ca(NO3)2·4H2O, Ba(NO3)2, Cr(NO3)3·9H2O, Al(NO3)3·9H2O, Ni(NO3)2·6H2O, MnCl2·4H2O, Co(NO3)2·6H2O, Cu(NO3)2·3H2O, Sr(NO3)2, Pb(NO3)2, Fe(NO3)3·9H2O and KCl) and amino-acids (cysteine (Cys), glycine (Gly), alanine (Ala), valine (Val), leucine (Leu), isoleucine (Ile), phenylalanine (Phe), proline (Pro), tryptophan (Try), serine (Ser), tyrosine (Tyr), glutamine (Glu), threonine (Thr), glutamic acid (Glu), lysine (Lys), arginine (Arg), histidine (His)) were purchased and used without further purification. All metal ions and amino-acids were dissolved (0.1 mol L−1) in distilled water. All other solvents used were of spectro-grade and purified through distillation prior to use.
Synthesis of 1O
The synthetic route of 1-(2-methyl-3-benzothiophenyl)-2-{2-methyl-5-(4-pheny)-(2-benzoylquinolines-8-benzothiazole)-3-thienyl}-perfluorocyclopentene (1O) was shown in Fig. 1. The intermediate 2 was synthesized according to literature.46 Synthesis of 1-(2-methyl-3-benzothiophenyl)-2-{2-methyl-5-phenyl-4-(2-formylquinoline-8-methoxyl)-3-thienyl} perfluorocyclopentene (3).
 |
| Fig. 1 Synthetic route for 1O. | |
To a compound 2 (0.58 g, 1.00 mmol) solution in anhydrous acetonitrile (30 mL), 8-hydroxyquinoline-2-carboxaldehyde (0.16 g, 0.90 mmol) and K2CO3 (0.33 g, 2.00 mmol) were added with continuously stirring. After 6 hours of refluxing, the mixture was cooled to room temperature and concentrated under vacuum. The crude product was purified through column chromatography on silica gel with petroleum ether/ethyl acetate (v/v = 6/1) as the eluent to afford compound 3 as a pale pink solid. The yield was 70%. Mp 340–342 K. 1H NMR (CDCl3, 400 MHz), δ (ppm) 1.93 (s, 3H), 2.30 (s, 3H), 5.47 (s, 2H), 7.15 (t, 2H, J = 8.0 Hz), 7.30–7.36 (m, 2H), 7.44–7.49 (m, 3H), 7.52–7.57 (m, 4H), 7.73 (d, 1H, J = 8.0 Hz), 8.08 (d, 1H, J = 8.4 Hz), 8.29 (d, 1H, J = 7.8 Hz), 10.33 (s, 1H).
Synthesis of 1-(2-methyl-3-benzothiophenyl)-2-{2-methyl-5-phenyl-4-(2-formylquinoline-8-benzothiazole)-3-thienyl} perfluorocyclopentene (1O).
Compound 3 (0.34 g, 0.5 mmol) and 2-aminothiophenol (0.06 g, 0.5 mmol) were dissolved in 5 mL of ethanol. After the reaction mixture was refluxed for 6 hours, a light purple powder crude product appeared. Then, the reaction mixture was cooled to room temperature, washed with cold ethanol and dried in air. The yield was (0.31 g) 80%. Mp 438–440 K. 1H NMR (CDCl3, 400 MHz), δ (ppm) 1.93 (s, 3H), 2.31 (s, 3H), 5.46 (s, 2H), 7.13–7.21 (m, 1H), 7.24 (s, 1H), 7.30–7.40 (m, 3H), 7.47–7.51 (m, 2H), 7.53–7.59 (m, 4H), 7.67 (d, 2H, J = 8.0 Hz), 7.75 (d, 1H, J = 7.7 Hz),8.02 (d, 1H, J = 7.7 Hz), 8.14 (d, 1H, J = 8.1 Hz), 8.30 (d, 1H, J = 8.5 Hz),8.53 (d, 1H, J = 8.6 Hz). 13C NMR (CDCl3, 100 MHz), δ (ppm) 14.82, 14.89, 71.06, 112.08, 118.73, 120.35, 120.49, 122.06, 122.09, 122.13, 122.18, 122.57, 123.77, 124.51, 124.95, 125.19, 125.58, 125.73, 125.84, 126.27, 127.70, 127.92, 130.36, 132.78, 136.60, 136.97, 137.04, 138.22, 140.45, 141.58, 141.69, 142.49, 150.21, 154.46. 19F NMR (DMSO, 376 MHz), δ (ppm) −130.15 (1F), −128.96 (1F), −108.29 (1F), −106.88 (1F), −106.36 (2F). (Fig. S1†) IR (KBr, ν, cm−1): 522, 724, 839, 1052, 1190, 1272, 1379, 1447, 1561. (Fig. S2†) LRMS: m/z 807.0 [M + Na]+.
Results and discussion
Photochromism and fluorescence of 1O
The photochromic and fluorescent behaviors of 1O were studied in THF solution (2.0 × 10−5 mol L−1) at room temperature, its absorption spectral, fluorescence spectral and color changes were induced by alternating irradiation with 297 nm UV light and visible light (λ > 500 nm) (Fig. 2). As shown in Fig. 2A, the absorption maximum of 1O was at 298 nm due to its π–π* transition.47,48 Upon the irradiation with 297 nm UV light, a new absorption band centered at 548 nm emerged due to the formation of the closed-ring isomer 1C of 1O with larger π-electron delocalization in the molecule, which was accompanied with a color change from colorless to purple. After 5 minutes of irradiation with 297 nm UV light, the photocyclization reaction reached a photostationary state (PSS), and the photoconversion ratio from the openring isomer 1O to the closed-ring isomer 1C was 84% based on the HPLC analysis. (Fig. S3†) The cyclization and cycloreversion quantum yield of the diarylethene were 0.34 and 0.027, respectively, with 1,2-bis(2-methyl-5-phenyl-3-thienyl)-perfluorocyclopentene as the reference.49 Reversely, the absorption spectrum of 1C with purple solution could be completely bleached to the initial state of 1O upon the visible light irradiation (λ > 500 nm). Fig. 2B showed the emission spectral changes of 1O upon photoirradiation when excited at 354 nm. Upon irradiation with 297 nm UV light, the emission peak of 1O was decreased significantly due to the formation of non-fluorescent closed-ring isomer 1C, which was accompanied by an obvious fluorescence intensity change from light blue to dark. In the photostationary state, the emission intensity of 1O was quenched to ca. 97%, and the fluorescence quantum yield of 1O to 1C was determined to be 0.459 to 0.008. Similarly, the fluorescence intensity of 1 could also be recovered with the irradiation of appropriate visible light (λ > 500 nm).
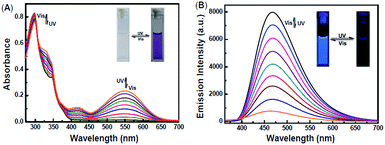 |
| Fig. 2 Changes in the absorption and fluorescence of 1O upon alternating irradiation with UV and visible light in THF: (A) absorption spectra change (2.0 × 10−5 mol L−1); (B) fluorescence change (2.0 × 10−5 mol L−1), excited at 354 nm. | |
Fluorescent turn-off detection of Hg2+
The fluorescence response of 1O toward 16 different metal ions (Hg2+, Cd2+, Zn2+, Mg2+, Ca2+, Ba2+, Cr3+, Al3+, Ni2+, Mn2+, Co2+, Cu2+, Sr2+, Pb2+, Fe3+, K+) was measured in THF (2.0 × 10−5 mol L−1) at room temperature. As shown in Fig. 3A, diarylethene 1O displayed a strong fluorescence with an emission of 468 nm. The addition of 5.0 equiv. metal ion (Cd2+, Zn2+, Mg2+, Ca2+, Ba2+, Cr3+, Al3+, Ni2+, Mn2+, Co2+, Cu2+, Sr2+, Pb2+ or K+) to the solution, 1O showed no significant changes in its fluorescence, while the same amount of Fe3+ caused the fluorescence intensity to decrease by almost 30%. The addition of Hg2+ into 1O resulted in the complete quench of its fluorescence intensity. The Fig. 3B shows the fluorescence color changes of 1O when the various metal ions added. These results indicated that 1O could easily discriminate Hg2+ from others metal ions.
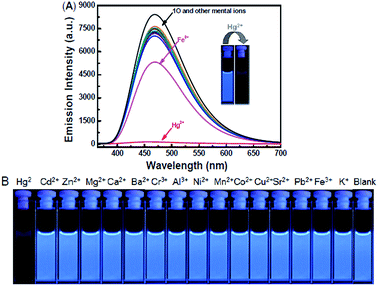 |
| Fig. 3 Competitive changes in the fluorescence of 1O in THF (2.0 × 10−5 mol L−1) with the addition of various metal ions (5.0 equiv.): (A) emission spectral changes, (B) image of the fluorescence color changes. | |
As shown in Fig. 4, when the 1O was titrated with Hg2+, the fluorescence intensity was significantly decreased until 3.2 equiv. of Hg2+ were added. With further addition of Hg2+, the fluorescence intensity was then decreased slowly. When 5.0 equiv. of Hg2+ were added, the fluorescence intensity of 1O was almost quenched completely. The quantum yields of 1O′ (the complex of 1O added the Hg2+) from 0.459 to 0.11 were determined. Meanwhile, based on the linear Benesi–Hildebrand expression and the Stern–Volmer plot, linear relationships between 1O′ fluorescence at 468 nm and 1O/[Hg2+] were obtained. The association constant of Hg2+ binding to diarylethene 1O was found as 1.11 × 104 L mol−1 (R = 0.99858). The limit of 1O as a fluorescent sensor to detect Hg2+ was 4.76 × 10−8 mol L−1, which was determined from a plot of intensity as a function of the concentration of Hg2+ (Fig. S4†).50
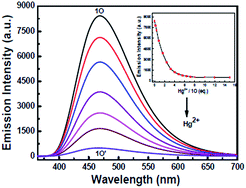 |
| Fig. 4 Changes in the fluorescence of 1O induced by Hg2+ in THF (2.0 × 10−5 mol L−1) and emission intensity changes of 1O induced by the addition of different equiv. of Hg2+. | |
Comparative, Job's plots and the ESI-MS experiments were conducted to elucidate the binding mode of 1O with Hg2+. The naphthol-benzothiazole moiety (NBTZ), quinolinol-benzoxazole moiety (QBOZ) and quinolinol-benzothiazole (QBTZ) moiety were synthesized to the comparative experiments and all the 1H NMR, 13C NMR datas were conducted. (Fig. S5†) Then, the detection to Hg2+ in THF (2.0 × 10−5 mol L−1) of the precursor 3, NBTZ, QBOZ and QBTZ were tested. The precursor 3 and the probe NBTZ have no effect on Hg2+, the probe QBOZ and QBTZ could all be quenched by Hg2+. (Fig. S6†) These phenomenon indicated that the Hg2+ could combine the atom “N” from the quinoline, the other bridging site could not be determined. While, the same structure that contains quinolinol-benzimidazole in our earlier published paper that could not recognize Hg2+.51 So, we think the other possible bridging site was the atom “S” from the benzothiazole. According to its ESI-MS spectrometry of 1O′ (Fig. S7†), it had a 1
:
1 complexation stoichiometry in 1O − Hg2+ complex with a peak at m/z 985.3 due to [1O + Hg2+]+, another peak at m/z 1209.9 due to [1O + Hg2+ + 2ClO4− + Na+]+. As shown in Fig. 5 of Job's plots, the maximum value was achieved when the molar fraction of [Hg2+]/([Hg2+] + [1O]) was about 0.5, which further demonstrated the 1
:
1 stoichiometry between 1O with Hg2+.
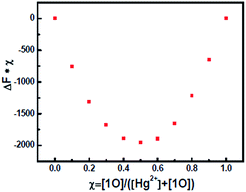 |
| Fig. 5 Job's plot of 1O with Hg2+. | |
In the competitive experiment, the fluorescence quenching of diarylethene 1O caused by 5.0 equiv. of Hg2+ was retained in the presence of the same concentration of other metal ions, including Cd2+, Zn2+, Mg2+, Ca2+, Ba2+, Cr3+, Al3+, Ni2+, Mn2+, Co2+, Cu2+, Sr2+, Pb2+, Fe3+ and K+. These results indicated that 1O had an excellent selectivity to Hg2+ over other metal ions (Fig. 6).
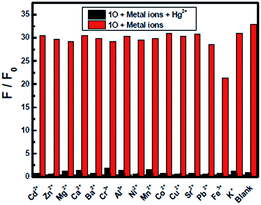 |
| Fig. 6 Competitively fluorescent response tests of 1O′ to various metal ions in THF (2.0 × 10−5 mol L−1). Red bars represent the addition of 5.0 equiv. of various metal ions to the solution of 1O. Black bars represent the addition of Hg2+ (5.0 equiv.) to the above solution, respectively. | |
Fluorescent turn-on response of 1O′ toward Cys
As shown in Fig. 7, studies on 1O′ fluorescent spectra with 17 different amino-acids, such as Cys, Gly, Ala, Val, Leu, Ile, Phe, Pro, Try, Ser, Tyr, Glu, Thr, Glu, Lys and Arg were conducted in THF. Becasue Hg2+ has high affinity towards thiol-based amino-acids,52–54 the fluorescence intensity of 1O′ almost recovered to the original of 1O only when Cys was added into the solution, and the quantum yields of 1O′ + Cys was measured as 0.426, while other amino-acids did not change the emission spectra. The fluorescence recovery might be due to release of 1O from the 1O′ through the interaction of thiol-containing Cys with Hg2+. Then, others organic thiol compounds like ethylene mercaptan, 2-aminothiophenol and 2-aminoethanethiol were tested and the fluorescence could also recovery. (Fig. S8†) These results showed that 1O′ could be successfully utilized to sensor thiol-containing compounds with turn-on fluorescence.
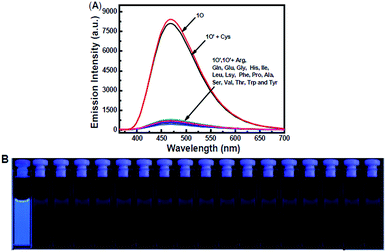 |
| Fig. 7 Changes in fluorescence of 1O′ induced by Cys in THF (2.0 × 10−5 mol L−1): (A) emission spectral changes; (B) image demonstrating changes in fluorescence. | |
As shown in Fig. 8, when Cys was added to the solution of 1O′, the fluorescence of the solution was enhanced rapidly. When 5.0 equiv. of Cys was added, the fluorescence recovered to the original of 1O, indicating that Cys took the Hg2+ away from 1O′. According to the Job's plots (Fig. 9) and ESI-MS spectrometry (Fig. S9†), the complex 1O′ − Cys had a 1
:
2 coordination stoichiometry. In the negative-ion mass spectrum, the peak at m/z 441.4 was assignable to [2Cys + Hg2+ + H]− and the other peak at m/z 785 was assignable to 1O. Similarly, according to Benesi–Hildebrand, the binding constant between 1O′ and Cys was 7.86 × 103 L mol−1 (R = 0.99351) and the detection limit of 1O′ was 7.06 × 10−8 mol L−1 (Fig. S10†).
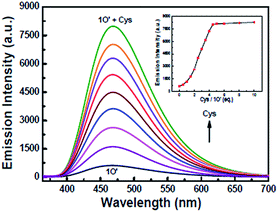 |
| Fig. 8 Fluorescence changes of 1O′ induced by Cys in THF (2.0 × 10−5 mol L−1) and emission intensity changes of 1O′ induced by different equiv. of Cys. | |
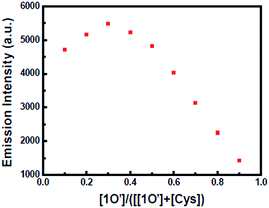 |
| Fig. 9 Job's plot of 1O′ with Cys. | |
As shown in Fig. 10, when 1O′ was treated with 5.0 equiv. of Cys in the presence of the same concentration of other amino-acids, the emission enhancement caused by Cys was retained with Gly, Ala, Val, Leu, Ile, Phe, Pro, Try, Ser, Tyr, Glu, Thr, Glu, Lys, Arg and His, which indicated that any other amino acids could not interference the detection of Cys by 1O′ in THF.
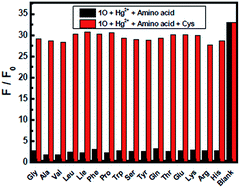 |
| Fig. 10 Competitive tests on the fluorescent responses of 1O′ + Cys to various amino acids in THF (2.0 × 10−5 mol L−1). Black bars represent the addition of 5.0 equiv. of various amino acids to the 1O′ solution. Red bars represent the addition of Cys (5.0 equiv.) to the above solution, respectively. | |
Application of 1O in logic circuits
Since the fluorescence intensity of 1O could be controlled by the stimulation of UV/Vis light, Hg2+ and Cys, a combinational logic circuit could be constructed with four inputs (In1: 297 nm UV light, In2: λ > 500 nm visible light, In3: Hg2+ and In4: Cys) and one output signal (the change of fluorescence intensity at 468 nm). The emission intensity of 1O at 468 nm was regarded as the initial value.
All the four inputs could be either “on” or “off” state with different Boolean values. When the emission intensity was quenched by over 50% of the initial value, the output signal could be regarded as ‘off’ state with a Boolean value of ‘0’. Otherwise, it was regarded as ‘on’ state with a Boolean value of ‘1’. The diarylethene 1O showed an on–off–on fluorescence switching behavior under the stimuli of different inputs. For example, when the string is ‘0, 0, 1, and 0’ the corresponding input signals of In1, In2, In3, and In4 are ‘off, off, on, off’. It means that 1O was stimulated by Hg2+ and its emission intensity quenched dramatically. And the output signal was ‘off’ with a digit of ‘0’. All possible strings of the three inputs were listed in Table 1 and the logic circuit corresponding to the truth table was shown in Fig. 11.
Table 1 Truth table for all possible strings of the four binary-input data and the corresponding output digit
Input |
Outputa λem = 468 nm |
In1 (UV) |
In2 (Vis) |
In3 (Hg2+) |
In4 (Cys) |
The emission intensity of 1O at 468 nm was regarded as the initial value and defined as 1, otherwise defined as 0. |
0 |
0 |
0 |
0 |
1 |
1 |
0 |
0 |
0 |
0 |
0 |
1 |
0 |
0 |
1 |
0 |
0 |
1 |
0 |
0 |
0 |
0 |
0 |
1 |
1 |
1 |
1 |
0 |
0 |
1 |
1 |
0 |
1 |
0 |
0 |
1 |
0 |
0 |
1 |
0 |
0 |
1 |
1 |
0 |
0 |
0 |
1 |
0 |
1 |
1 |
0 |
0 |
1 |
1 |
1 |
1 |
1 |
1 |
0 |
0 |
1 |
1 |
0 |
1 |
1 |
1 |
0 |
1 |
1 |
0 |
0 |
1 |
1 |
1 |
1 |
1 |
1 |
1 |
1 |
1 |
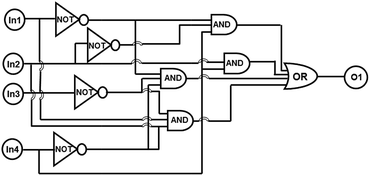 |
| Fig. 11 Combinational logic circuits equivalent to the truth table given in Table 1: In1 (UV), In2 (Vis), In3 (Hg2+), In4 (Cys). | |
Conclusions
In summary, a new diarylethene-based “on–off–on” fluorescence sensor for the sequential recognition of Hg2+ and Cys with very high selectivity and sensitivity was designed and synthesized successfully. When Hg2+ was added into the solution of 1O, fluorescence of the 1O solution was quenched because 1O bond to Hg2+ into [1O − Hg2+] (1O′) 1
:
1 stoichiometry. When Cys was added into 1O′ solution, the fluorescence was enhanced significantly due to the reaction between 1O′ and Cys in 1
:
2 stoichiometry. Based on the fact that the fluorescence of the diarylethene could be effectively modulated with the stimulation of light and chemical species, a logic circuit was also constructed successfully with the fluorescence intensity as the output signal, and UV/Vis, Hg2+ and Cys as the inputs, which should have great potentials in future fluorescent sensors for some special species.
Acknowledgements
The authors are grateful for the financial support from the National Natural Science Foundation of China (21662015, 21363009, 21362013, 51373072), the Project of Jiangxi Academic and Technological leader (20142BCB22010), and the Project of the Science Funds of Jiangxi Education Office (KJLD13069). YC2015-S392.
Notes and references
- H. N. Kim, W. X. Ren, J. S Kim and J. Yoon, Chem. Soc. Rev., 2012, 41, 3210–3244 RSC.
- M. Li, X. J. Zhou, W. Q. Ding, S. W. Guo and N. Q. Wu, Biosens. Bioelectron., 2013, 79, 889–893 CrossRef PubMed.
- N. Zheng, Q. C. Wang, X. W. Zhang, D. M. Zheng, Z. S. Zhang and S. Q. Zhang, Sci. Total Environ., 2007, 387, 96–104 CrossRef CAS PubMed.
- J. K. Virtanen, T. H. Rissanen, S. Voutilainen and T. P. Tuomainen, J. Nutr. Biochem., 2007, 18, 75–85 CrossRef CAS PubMed.
- T. W. Clarkson, L. Magos and G. J. Myers, N. Engl. J. Med., 2003, 349, 1731–1737 CrossRef CAS PubMed.
- J. Gutknecht, J. Membr. Biol., 1981, 61, 61–66 CrossRef CAS.
- E. M. Nolan and S. J. Lippard, Chem. Rev., 2008, 108, 3443–3480 CrossRef CAS PubMed.
- Y. W. Chen, C. F. Huang, K. S. Tsai, R. S. Yang, C. C. Yen, C. Y. Yang, S. Y. Lin-Shiau and S. H. Liu, Chem. Res. Toxicol., 2006, 19, 1080–1085 CrossRef CAS PubMed.
- P. Holmes, K. A. F. James and L. S. Levy, Sci. Total Environ., 2009, 408, 171–182 CrossRef CAS PubMed.
- M. Ghaedi, M. R. Fathi, A. Shokrollahi and F. Shajarat, Anal. Lett., 2006, 39, 1171–1185 CrossRef CAS.
- T. Matousek, A. Z. Hernández, M. Svoboda, L. Langrová, B. M. Adair, Z. Drobná, D. J. Thomas, M. Styblo and J. Dedina, Spectrochim. Acta, Part B, 2008, 63, 396–406 CrossRef PubMed.
- X. Y. Jia, Y. Han, X. L. Liu, T. C. Duan and H. T. Chen, Spectrochim. Acta, Part B, 2011, 66, 88–92 CrossRef.
- Y. L. Feng, H. W. Chen and H. Y. Chen, J. Anal. Chem., 1998, 361, 155–157 CAS.
- B. Han, J. Yuan and E. Wang, Anal. Chem., 2009, 81, 5569–5573 CrossRef CAS PubMed.
- B. Cao, C. Yuan, B. Liu, C. Jiang, G. Guan and M. Y. Han, Anal. Chim. Acta, 2013, 786, 146–152 CrossRef CAS PubMed.
- C. Wang, D. Zhang, X. Huang, P. Ding, Z. Wang, Y. Zhao and Y. Ye, Sens. Actuators, B, 2014, 198, 33–40 CrossRef CAS.
- D. H. Kim, J. Seong, H. Lee and K. H. Lee, Sens. Actuators, B, 2014, 196, 421–428 CrossRef CAS.
- M. Li, Q. Wang, X. Shi, L. A. Hornak and N. Wu, Anal. Chem., 2011, 83, 7061–7065 CrossRef CAS PubMed.
- R. M. Kong, X. B. Zhang, L. L. Zhang, X. Y. Jin, S. Y. Huan, G. L. Shen and R. Q. Yu, Chem. Commun., 2009, 5633–5635 RSC.
- M. Hollenstein, C. Hipolito, C. Lam, D. Dietrich and D. M. Perrin, Angew. Chem., Int. Ed., 2008, 47, 4346–4350 CrossRef CAS PubMed.
- H. Urata, E. Yamaguchi, T. Funai, Y. Matsumura and S. I. Wada, Angew. Chem., 2010, 122, 6666–6669 CrossRef.
- Y. Zhao and Z. Zhong, J. Am. Chem. Soc., 2006, 128, 9988–9989 CrossRef CAS PubMed.
- S. Seshadri, A. Beiser, J. P. Selhub, F. I. Jacques, H. Rosenberg, R. B. D. Agostino, P. W. Wilson and P. A. N. Wolf, N. Engl. J. Med., 2002, 346, 476–483 CrossRef CAS PubMed.
- S. A. Lee, J. J. Lee, J. W. Shin, K. S. Min and C. Kim, Dyes Pigm., 2015, 116, 131–138 CrossRef CAS.
- Y. Yang, F. J. Huo, C. Yin, J. Chao and Y. Zhang, Dyes Pigm., 2015, 114, 105–109 CrossRef CAS.
- C. Hwang, A. J. Sinskey and H. F. Lodish, Science, 1992, 257, 1496–1502 CAS.
- L. Wang, Q. Zhou, B. Zhu, L. Yan, Z. Ma, B. Du and X. Zhang, Dyes Pigm., 2012, 95, 275–279 CrossRef CAS.
- F. Jalilehvand, B. O. Leung, M. Izadifard and E. Damian, Inorg. Chem., 2006, 45, 66–73 CrossRef CAS PubMed.
- J. S. Wu, R. L. Sheng, W. M. Liu, P. F. Wang, J. J. Ma, H. Y. Zhang and X. Q. Zhuang, Inorg. Chem., 2011, 50, 6543–6551 CrossRef CAS PubMed.
- G. Mcdonnell and D. A. Russell, Clin. Microbiol. Rev., 1999, 12, 147–157 CAS.
- S. Shahrokhian, Anal. Chem., 2001, 73, 5972–5978 CrossRef CAS PubMed.
- C. Jacob, G. I. Giles, N. M. Giles and H. Sies, Angew. Chem., Int. Ed., 2003, 42, 4742–4758 CrossRef CAS PubMed.
- Z. Li, Y. Wang, Y. N. Ni and S. Kokot, Sens. Actuators, B, 2015, 207, 490–497 CrossRef CAS.
- B. L. Feringa, Molecular Switches, 2001 Search PubMed.
- V. Balzani, M. Venturi and A. Cred, Molecular Devices and Machines, 2003 Search PubMed.
- I. Willner, Acc. Chem. Res., 1997, 30, 347–356 CrossRef CAS.
- K. Higashiguchi, K. Matsuda, N. Tanifuji and M. Irie, J. Am. Chem. Soc., 2005, 127, 8922–8923 CrossRef CAS PubMed.
- B. M. Neilson and C. W. Bielawski, J. Am. Chem. Soc., 2012, 134, 12693–12699 CrossRef CAS PubMed.
- S. Kawata and Y. Kawata, Chem. Rev., 2000, 100, 1777–1788 CrossRef CAS PubMed.
- Y. Nakayama, K. Hayashi and M. Irie, J. Org. Chem., 1990, 55, 2592–2596 CrossRef CAS.
- F. Duan, G. Liu, P. Liu, C. B. Fan and S. Z. Pu, Tetrahedron, 2016, 72, 3213–3220 CrossRef CAS.
- S. J. Xia, G. Liu and S. Z. Pu, J. Mater. Chem. C, 2015, 3, 4023 RSC.
- S. Q. Cui, Z. Y. Tian, S. Z. Pu and Y. F. Dai, RSC Adv., 2016, 6, 19957 RSC.
- Q. Zou, J. Y. Jin, B. Xu, L. Ding and H. Tian, Tetrahedron, 2011, 67, 915–921 CrossRef CAS.
- C. H. Zheng, S. Z. Pu, G. Liu, B. Chen and Y. F. Dai, Dyes Pigm., 2013, 98, 280–285 CrossRef CAS.
- S. Z. Pu, L. L. Ma, G. Liu, H. C. Ding and B. Chen, Dyes Pigm., 2015, 113, 70–77 CrossRef CAS.
- Z. X. Li, L. Y. Liao, W. Sun, C. H. Xu, C. Zhang, C. J. Fang and C. H. Yan, J. Mater. Chem. C, 2008, 112, 5190–5196 CAS.
- L. Yuan, W. Y. Lin, Z. M. Cao, J. L. Wang and B. Chen, Chem.–Eur. J., 2012, 18, 1247–1255 CrossRef CAS PubMed.
- M. Irie, T. Lifka, S. Kobatake and N. Kato, J. Am. Chem. Soc., 2000, 122, 4871–4876 CrossRef CAS.
- S. Y. Tao, Y. Wei, C. Wang and B. J. Ding, RSC Adv., 2014, 4, 46955–46961 RSC.
- G. Li, G. Liu, D. B. Zhang and S. Z. Pu, Tetrahedron, 2016, 72, 6390–6396 CrossRef CAS.
- Y. Y. Fu, H. X. Li, W. P. Hu and D. B. Zhu, Chem. Commun., 2005, 3189–3191 RSC.
- N. Shao, J. Y. Jin, S. M. Cheung and R. H. Yang, Angew. Chem., Int. Ed., 2006, 45, 4944–4948 CrossRef CAS PubMed.
- Z. Q, Guo, S. W. Nam, S. S. Park and J. Y. Yoon, Chem. Sci., 2012, 3, 2760–2765 RSC.
Footnote |
† Electronic supplementary information (ESI) available. See DOI: 10.1039/c6ra27773j |
|
This journal is © The Royal Society of Chemistry 2017 |
Click here to see how this site uses Cookies. View our privacy policy here.