DOI:
10.1039/C6RA27519B
(Paper)
RSC Adv., 2017,
7, 11510-11521
H3PW12O40 supported on functionalized polyoxometalate organic–inorganic hybrid nanoparticles as efficient catalysts for three-component Mannich-type reactions in water†
Received
29th November 2016
, Accepted 8th February 2017
First published on 14th February 2017
Abstract
Two new types of catalyst were synthesized by the immobilization of H3PW12O40 (HPW12) on the surface of organic–inorganic polyoxometalate nanoparticles of H6Cu2[PPDA]6[SiW9Cu3O37]·12H2O (HybPOM) (PPDA = p-phenylenediamine) and Go/Fe3O4/HybPOM nanoparticles. These catalysts were characterized by thermogravimetric analysis (TGA), Fourier transform infrared (FT-IR) spectroscopy, scanning electron microscopy (SEM), energy dispersive X-ray analysis (EDX) and alternating gradient force magnetometry (AGFM). The IR spectroscopy as well as XRPD reveal that HPW12 were immobilized on the support. The potentiometric titration with n-butylamine reveal that the catalysts can be classified as strong acids. The results show that the particles are mostly spherical in shape and have an average size in the range of 20–50 nm. The catalytic activities of the catalysts were probed through one-pot three-component Mannich-type reactions of aldehydes, amines and ketones in water at room temperature. The catalysts were re-used at least five times without any loss of their high catalytic activity.
1. Introduction
Polyoxometalates (POMs), discrete anionic metal-oxo clusters, can be linked together through cationic moieties to build materials with incredible structural diversity which exhibit a wide variety of compositions and structural versatility,1–3 as well as important optical,4 catalytic,5–9 and magnetic10–13 properties. POM clusters occupy a vast parameter space between the mononuclear metalate species and the bulk oxide.14 POMs can also self-assemble into ordered patterns at the nanometer scale, and thus have multilevel ordered structures.15,16 The great diversity in structure and composition of POMs make them attractive materials in many fields.17 One focus is to use them as both homogeneous and heterogeneous catalysts in oxidation and acid promoted reactions. Heteropoly acids (HPAs) such as H3PW12O40 have been extensively studied as acid catalysts for many reactions. Despite the above advantages, use of POMs is restricted owing to the fact that they are non-porous solids with low surface area (of less than 10 m2 g−1). Because of their ionic nature they dissolve well in polar solvents such as water, alcohols, ketones, acetonitrile and dimethylsulfoxide which makes them difficult to separate from the reaction products and their inevitable loss during recycling. Hence, efforts have also been made to study the support of HPAs on inert or weak acidic high surface area materials such as, silica,18 zeolite,19 and alumina.20 The application of supported HPAs increases the specific surface area of the catalysts and modifies their catalytic properties. Thus, immobilization of HPAs on supports show a greater number of surface acid sites than their bulk components. The use of nanoparticles (NPs) offers many advantages for supporting homogeneous catalysts due to their unique size and physical properties. NPs could have a higher catalyst loading capacity and a higher dispersion than many conventional support matrices. Recently, supported HPAs have been developed for a wide range of organic reactions in fundamental research.21,22
Easier recovery and recycling after carrying out reaction through simple separation processes are the advantages that supported HPAs have compared to homogeneous examples. In addition, heteropoly acids are more active catalysts for various reactions in solution than conventional inorganic and organic acids and they have many environmental benefits. They were tested as catalysts for several reactions with the final objective of industrial use,23–25 as alcohol dehydration,26 alkylation27 or esterification28 reactions. Among heteropoly acids, 12-tungstophosphoric acids are the most widely used catalysts owing to their high acid strength and thermal stability, and relatively low reducibility. Recently, the supported heteropoly acids as heterogeneous catalysts have attracted much attention. H3PW12O40 have been immobilized on Fe3O4 functionalized nanoparticles29–31 and successfully applied as active species in organic reaction catalysis.32,33 In this study, organic–inorganic nanohybride of HybPOM was used as a new kind of high surface area material for supporting homogeneous H3PW12O40. The functionalized organic–inorganic polyoxometalates of HybPOM was synthesized by a simple and high yield reaction.34 Ultimately, mixing a suspension of HybPOM with an ethanolic solution of HPW12 leads to the formation of HybPOM/HPW12 NPs which involves the reaction of H3PW12O40 with surface amine groups of HybPOM. Protonation of amine groups gives positively charged cations which bound electrostatically to the heteropolyanions.
Despite the high surface areas of HybPOM/HPW12, filtration or centrifugation is needed and the separation process is still a difficult and time consuming process. Magnetic nanoparticles are excellent supports for various catalysts35,36 and can easily be separated and recycled from the products by an external magnet. Surface functionalized Go/Fe3O4 nanoparticles are a kind of novel functional material which has been widely used in biotechnology and catalysis. The Go/Fe3O4/HybPOM magnetic nanoparticle was synthesized simply by addition of HybPOM to the ethanol disperse solution of Go/Fe3O4 nanoparticle. The GO because of its exceptionally high surface area (2630 m2 g−1) and abundant oxygenate groups such as epoxy, hydroxyl, and carboxyl groups on their surface, is as an ideal support for immobilizing and improving POMs catalytic activity. The GO/Fe3O4 nanoparticle, has key properties such as magnetic separation, the large surface area and ease of functionalizing with various chemical groups to increase their dependence toward target compounds. Through a nucleophilic attack by available NH2 groups on the surface HybPOM hybrid with functional groups such as epoxy, hydroxyl, and carboxyl groups on the surface of GO–Fe3O4 magnetic nanoparticle, the hybrid material is grafted via C–N bond formation.34 Once again mixing a suspension of the Go/Fe3O4/HybPOM with an ethanolic solution of HPW12 lead to the formation of Go/Fe3O4/HybPOM/HPW12 NPs.
The prepared Go/Fe3O4/HybPOM/HPW12 hybrid composite can be easily separated by magnetic separation from the medium after reaction. The Go/Fe3O4/HybPOM/HPW12 hybrid composite show the same catalytic efficiency compared to the HybPOM/HPW12 and can be easily manipulated by external magnetic field.
In this paper, we synthesized HybPOM/HPW12 and Go/Fe3O4/HybPOM/HPW12 nanoparticles by immobilization of Keggin type heteropoly acid H3PW12O40·xH2O on organic–inorganic nano hybrid of H6Cu2[PPDA]6[SiW9Cu3O37]·12H2O (HybPOM) and nanocomposite of Go/Fe3O4/HybPOM nanoparticles, respectively and assessed their catalytic activity in Mannich-type reactions in water. Indeed, the venerable Mannich reactions and its variants represent one of the more powerful constructs for alkaloid synthesis.37 To the best of our knowledge, this is the first report containing immobilization of a heteropolyacid on organic–inorganic nano hybrid. Synthesis, characterization and catalytic application of new nano catalysts have been investigated.
2. Experimental section
Materials and apparatus
All reagents and solvents were commercially obtained and used without further purification. The 12-tungstophosphoric acid (H3PW12O40·xH2O) was prepared according to a reported procedure.38 The GO–Fe3O4 was prepared according to the literature.39
Powder XRD was obtained by an X'PertPro Panalytical, Holland diffractometer in 40 kV and 30 mA with a CuKα radiation (λ = 1.5418 Å). Infrared spectra were recorded on a Bruker Vector 22 FT-IR using KBr plate. The morphology of nanocomposites was revealed by a scanning electron microscope (FESEM-TESCAN MIRA3). The elements in the nanocomposite samples were probed energy-dispersive X-ray (EDX) spectroscopy accessory to the FESEM-TESCAN MIRA3 scanning electron microscopy. The magnetic properties were investigated by a home-made alternative gradient force magnetometer (AGFM) in the magnetic field range of −5000 to 5000 Oe at room temperature. Thermo gravimetric analysis-differential thermal analysis (TGA-DTA) was carried out using a STA PT-1000 LINSEIS.
Synthesis of nanohybrides
Preparation of H6Cu2[PDA]6[SiW9Cu3O37]·12H2O nanohybrid (HybPOM). HybPOM was prepared by the following stated method.34 A 2.00 g (0.725 mmol) of Na9H[β-SiW9O34]·18H2O was dissolved in water (100 mL) at room temperature. Cu(C2H3O2)2·H2O (3.00 g, 15 mmol) was added to the solution while stirring, resulting in a blue solution. Then, 0.7 g (6.5 mmol) of PPDA was slowly added to this blue solution while stirring, producing a blue-brown precipitate. After stirring for 4 h, the precipitate color changed from blue-brown to black. Obtained black precipitate was centrifuged and washed several times with water and acetonitrile, then air-dried at room temperature.
Preparation of GO@Fe3O4@HybPOM nanohybride. GO@Fe3O4@HybPOM was prepared as per the following method.34 To disperse GO–Fe3O4 in 30 mL of absolute ethanol, an appropriate amount of HybPOM was added and the mixture was sonicated for 10 min to form a homogeneous dispersion. Then, the mixture was stirred under reflux condition for 24 h. The obtained solid was then magnetically collected from the solution and washed three times with water and ethanol, and dried at 50 °C.
Preparation of HybPOM/HPW12 nanohybride. A 0.500 g of HybPOM was dispersed in 25 mL of absolute ethanol by sonication for 20 minutes. An appropriate amount of dissolved H3PW12O40·xH2O in the absolute ethanol was added to the above solution while stirring. The resulting mixture was stirred under reflux condition for 48 h. The crude product was separated by centrifuges, washed several times with ethanol and dried at 50 °C under reduced pressure.
Preparation of Go/Fe3O4/HybPOM/HPW12 nanohybride. 0.500 g Go/Fe3O4/HybPOM was suspended in 25 mL of absolute ethanol and then an appropriate amount of H3PW12O40·xH2O dissolved in absolute ethanol was added to the suspension while stirring. The mixture was stirred under reflux condition for 24 hours. The obtained nanohybride were magnetically collected, washed three times with ethanol (3 × 5 mL), and dried at 50 °C under reduced pressure.
Titration of the catalysts acidity
The acidity of HybPOM/HPW12 and Go/Fe3O4/HybPOM/HPW12 was probed by potentiometric titration.40 HybPOM/HPW12 or Go/Fe3O4/HybPOM/HPW12 (0.05 g) was suspended in acetonitrile, and stirred for 3 h. Then, the suspension was titrated with 0.05 M n-butylamine in acetonitrile at 0.05 mL min−1. The electrode potential variation was measured with a WTW digital nanolab model using a double-junction electrode.
General procedure for the synthesis of β-amino ketones catalyzed by HybPOM/HPW12
Typically, the catalyst (0.005 g) was added to a mixture of aldehyde (1 mmol), amine (1 mmol), cyclohexanone (2 mmol) and H2O (1 mL). The reaction mixture was vigorously stirred at room temperature for an appropriate time. The progress of the reaction was monitored by TLC. After completion of the reaction, the catalyst was separated by centrifuge, washed with ethanol and water, vacuum dried and stored for subsequent runs. The reaction mixture was extracted with H2O and EtOAc. The organic layer was dried over MgSO4 and then evaporated under reduced pressure. This solution was concentrated at room temperature to yield the crude products. The crude products were purified either by crystallization from ethanol, giving of β-amino ketones in good yield. The reaction product was characterized by IR and mass spectroscopy.
General procedure for the synthesis of β-amino ketones catalyzed by Go/Fe3O4/HybPOM/HPW12
Typically, the catalyst (0.007 g) was added to a mixture of aldehyde (1 mmol), amine (1 mmol), cyclohexanone (2 mmol) and H2O (1 mL). The reaction mixture was vigorously stirred at room temperature for an appropriate time. The progress of the reaction was monitored by TLC. After completion of the reaction, the catalyst was separated by external magnet and washed with ethanol and water, vacuum dried and stored for subsequent runs. The reaction mixture was extracted with H2O and EtOAc. The organic layer was dried over MgSO4 and then evaporated under reduced pressure. The crude products were purified either by crystallization from ethanol, giving of β-amino ketones in good yield.
Characterization of organic products
2-(Phenyl(phenylamino)methyl)cyclohexanone (Table 2, entry 1). White solid, mp 128–129 °C;53 FT-IR (KBr, cm−1) 1440–1594 (C
C, aromatic), 1701 (C
O, carbonyl), 3349 (NH, second amine), 2925, 2860 (C–H, aliphatic), 3015 (C–H, aromatic). 1H NMR (250 MHz, CDCl3) δ (ppm): 7.80 (s, 1H), 7.45–7.26 (m, 7H), 6.68–6.64 (m, 2H), 4.80 (d, 0.38Hsyn, J = 2.25 Hz), 4.63–4.60 (m, 0.36Hanti), 2.94–2.84 (m, 2H), 2.54–2.34 (m, 2H), 1.91–1.77 (m, 4H), 1.25 (s, 1H). (syn/anti: 50/50). EI-MS (70 eV): m/z (%) = 279 (M+˙, 11), 182 (M+˙–C6H9O, 48).
2-((2-Chlorophenyl)(phenylamino)methyl)cyclohexanone (Table 2, entry 2). White solid, mp 137–139 °C;54 FT-IR (KBr, cm−1) 1434–1600 (C
C, aromatic), 1704 (C
O, carbonyl), 3380 (NH, second amine), 2933, 2857 (C–H, aliphatic), 3045 (C–H, aromatic); 1H NMR (250 MHz, CDCl3) δ (ppm): 7.91 (s, 1H), 7.51–7.40 (m, 3H), 7.33–7.26 (m, 3H), 6.65–6.62 (m, 1H), 6.51 (d, 1H, J = 8 Hz), 5.33 (d, 0.30Hsyn, J = 3.25 Hz), 4.89 (d, 0.41Hanti, J = 4.5 Hz), 2.77–2.54 (m, 7H), 1.96–1.92 (m, 2H). (syn/anti: 42/58); EI-MS (70 eV): m/z (%) = 315 [(M+˙ + 2), 3], 313 (M+˙, 9), 216 (M+˙–C6H9O, 100).
2-((4-Bromophenyl)(phenylamino)methyl)cyclohexanone (Table 2, entry 3). White solid, mp 109–110 °C;55 FT-IR (KBr, cm−1) 1484–1610 (C
C, aromatic), 1709 (C
O, carbonyl), 3380 (NH, second amine), 2927, 2856 (C–H, aliphatic), 3029 (C–H, aromatic); 1H NMR (250 MHz, CDCl3) δ (ppm): 7.49–7.41 (m, 2H), 7.41–7.39 (m, 2H), 7.26–7.23 (m, 2H), 7.23–7.07 (m, 2H), 6.69–6.64 (m, 1H), 6.51 (d, 1H, J = 6 Hz), 4.72 (d, 0.41Hsyn, J = 4 Hz), 4.58 (d, 0.54Hanti, J = 6.25 Hz), 2.78–2.76 (m, 1H), 2.53–2.38 (m, 1H), 2.36–2.31 (m, 1H), 1.94–1.71 (m, 6H). (syn/anti: 43/57); EI-MS (70 eV): m/z (%) = 359 [(M+˙ + 2), 6], 357 (M+˙, 7), 260 (M+˙–C6H9O, 100).
2-((4-Chlorophenylamino)(phenyl)methyl)cyclohexanone (Table 2, entry 5). White solid, mp 137–138 °C;56 FT-IR (KBr, cm−1) 1490–1592 (C
C, aromatic), 1703 (C
O, carbonyl), 3369 (NH, second amine), 2940, 2850 (C–H, aliphatic), 3052 (C–H, aromatic); EI-MS (70 eV): m/z (%) = 315 [(M+˙ + 2), 3], 313 (M+˙, 9), 216 (M+˙–C6H9O, 85).
3. Results and discussion
Characterization of nanomaterials
The results of IR spectrum showed that all amine groups on the surface HybPOM were not linked to the GO–Fe3O4 magnetic nanoparticle. Therefore, H3PW12O40 might interact with the reminded free amine groups on the surface GO–Fe3O4 for the formation of Go/Fe3O4/HybPOM/HPW12 nanoparticles. The catalytic ability of the prepared HybPOM/HPW12 and Go/Fe3O4/HybPOM/HPW12 hybrid composite was evaluated in synthesis of various β-amino ketones via Mannich reactions in aqueous media. The schematic pathway for preparation Go/Fe3O4/HybPOM/HPW12 hybrid composite is presented in Scheme 1.
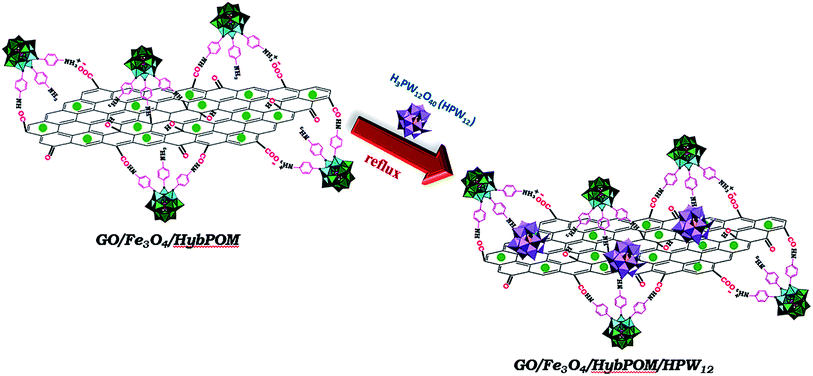 |
| Scheme 1 The schematic pathway for preparing Go/Fe3O4/HybPOM/HPW12 hybrid composite. | |
IR spectra of HybPOM, HybPOM/HPW12 and HPW12 samples are shown in Fig. 1. The PW12O403− Keggin ion structure is well known and shows typical bands for absorptions at 1081 (P–O), 985 (W
O), 897 and 803 (W–O–W) cm−1.41 As a result, the new peaks are observed at 1079 and 962 cm−1 in IR spectra of HybPOM/HPW12 indicating that HPW12 was anchored onto HybPOM successfully. IR spectra of Go/Fe3O4/HybPOM, Go/Fe3O4/HybPOM/HPW12 and HPW12 samples are shown in Fig. 2.
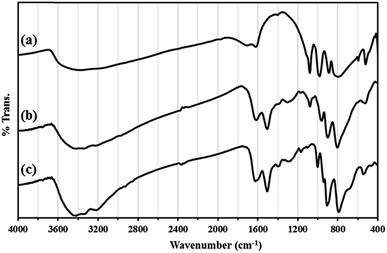 |
| Fig. 1 IR spectra of HPW12 (a), HybPOM/HPW12 (b) and HybPOM (c). | |
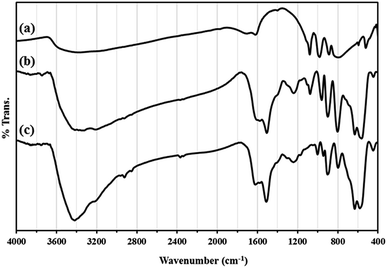 |
| Fig. 2 IR spectra of HPW12 (a) Go/Fe3O4/HybPOM/HPW12 (b) and Go/Fe3O4/HybPOM (c). | |
In Fig. 2, peaks at 573–630 cm−1 in the Go/Fe3O4/HybPOM/HPW12 composite are attributed to Fe–O stretching vibration and the peak at 1080 cm−1 is attributed to the asymmetric stretching vibration P–O.
The XRD diffraction patterns of Go/Fe3O4/HybPOM (a) HybPOM/HPW12 (b) and Go/Fe3O4/HybPOM/HPW12 (c) are shown in Fig. 3. X-ray diffractions pattern of HybPOM, as a typical amorphous state material is observed in the X-ray spectra of HybPOM/HPW12 and Go/Fe3O4/HybPOM/HPW12. In Fig. 3, peaks corresponding to the Fe3O4 are observed at 2θ = 30.52°, 35.85°, 43.58°, 53.96°, 57.43°, 63.16° and 74.65° which match well with the standard Fe3O4 sample (JCPDS file no. 19-0629).40
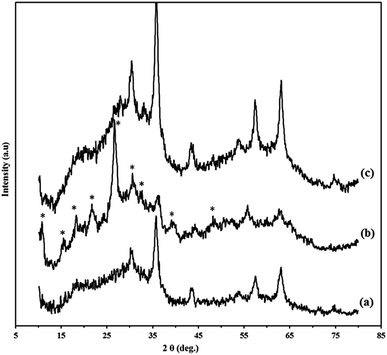 |
| Fig. 3 The XRD diffraction patterns of Go/Fe3O4/HybPOM (a) HybPOM/HPW12 (b) and Go/Fe3O4/HybPOM/HPW12 (c). | |
The peaks corresponding to HPW12 at 2θ = 30.52°, 35.85°, 43.58°, 53.96°, are observed in X-ray powder diffractions of HybPOM/HPW12 and Go/Fe3O4/HybPOM/HPW12 which match well with the X-ray powder diffraction pattern of H3PW12O40·6H2O.30 The X-ray powder diffractions thus confirm that the Keggin structure of the HPW12 compound was preserved with its immobilization on the supports.
Numerous methods have been employed to describe the acidity of POMs in the solid state both qualitatively and quantitatively. Titration with Hammett indicators, temperature programmed desorption of adsorbed molecules such as ammonia or pyridine, adsorption microcalorimetry, NMR spectroscopy and catalytic probe reactions42 are the most common methods for characterizing solid acids. The nature of the support apparently affecting the acidity of the supported HPAs by means of potentiometric titration with n-butylamine allows the estimation of the number of acid sites and their distribution.22
The titration curves obtained for HybPOM and HybPOM/HPW12 are illustrated in Fig. 4A and those of Go/Fe3O4/HybPOM and Go/Fe3O4/HybPOM/HPW12 are shown in Fig. 4B. It is believed that the initial electrode potential (Ei) indicates the maximum strength of the acid sites and the value from which the plateau is reached (meq. n-butylamine/total amount of meq. H+) indicates the total number of acid sites that are present in the titrated solid. On the other hand, the acid strength of these sites may be classified according to the following scale: Ei > 100 mV (very strong sites), 0 < Ei < 100 mV (strong sites), −100 < Ei < 0 (weak sites) and Ei < −100 mV (very weak sites).43 The HybPOM (Fig. 4A(a)) and Go/Fe3O4/HybPOM (Fig. 4B(a)) showed very weak and weak acid sites (Ei = −165.9 and −73.8 mV), respectively. Difference in the acid strength of HybPOM and Go/Fe3O4/HybPOM is attributed to the total number of free NH2 groups on them. For Go/Fe3O4/HybPOM, some of the NH2 groups are involved in the bonding with Go/Fe3O4 nanoparticles.
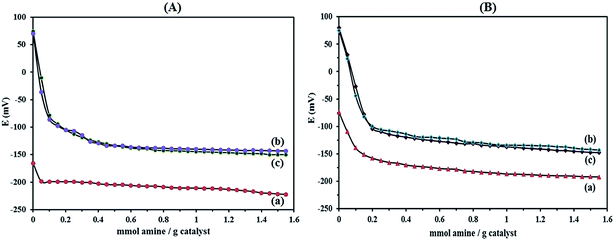 |
| Fig. 4 The potentiometric titration with n-butylamine curves of (A) HybPOM (a) HybPOM/HPW12 (b) HybPOM/HPW12 after five consecutive runs (c); and (B) Go/Fe3O4/HybPOM (a) Go/Fe3O4/HybPOM/HPW12 (b) and Go/Fe3O4/HybPOM/HPW12 after five consecutive runs (c). | |
As expected, the strength of the acid sites of the HPW12 decreases with its addition to the HybPOM and Go/Fe3O4/HybPOM surfaces. This effect depends on the extent of the interaction of HPW12 with the support. According to potentiometric titration curves, HPW12 presented very strong acidic sites (Ei = 572.2 mV).44 Potentiometric titration results of HybPOM/HPW12 (Fig. 4A(b)) and Go/Fe3O4/HybPOM/HPW12 (Fig. 4B(b)) samples showed the lower Ei value of 74.1 and 80 mV, respectively compared to the HPW12 which might be a result of a strong interaction with the HybPOM and Go/Fe3O4/HybPOM support. Here, differences in the acid strength of HybPOM/HPW12 and Go/Fe3O4/HybPOM/HPW12 samples are attributed to the total number of free NH2 groups on HybPOM and Go/Fe3O4/HybPOM support.
The magnetization of GO–Fe3O4, Go/Fe3O4/HybPOM and Go/Fe3O4/HybPOM/HPW12 samples was measured at room temperature as shown in Fig. 5. The specific saturation magnetization of GO–Fe3O4, is 29.2 emu g−1 value is smaller than the reported value of bulk Fe3O4 of 92 emu g−1.45 The specific saturation magnetization, of Go/Fe3O4/HybPOM and Go/Fe3O4/HybPOM/HPW12 are 22.47 and 16.64 emu g−1, respectively. A decrease in saturation magnetization observed for Go/Fe3O4/HybPOM and Go/Fe3O4/HybPOM/HPW12 could be attributed to the increased mass and the loading of the HybPOM and HPW12. Even with this reduction in the saturation magnetization, complete magnetic separation of Go/Fe3O4/HybPOM and Go/Fe3O4/HybPOM/HPW12 was achieved in <10 s by placing a magnet near the vessels containing the aqueous dispersion of the nanoparticles.
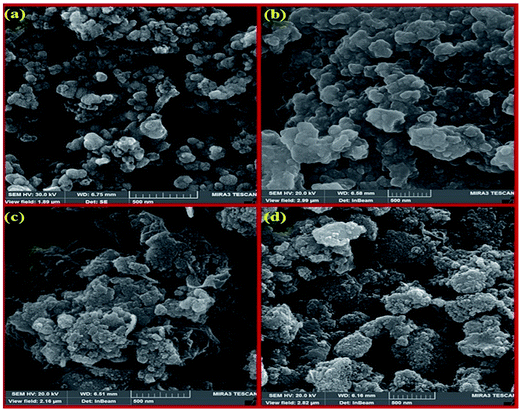 |
| Fig. 5 The SEM images of HybPOM (a), HybPOM/HPW12 (b), Go/Fe3O4/HybPOM (c) and Go/Fe3O4/HybPOM/HPW12 (d). | |
Fig. 6 shows the SEM images of HybPOM (a), HybPOM/HPW12 (b), Go/Fe3O4/HybPOM (c) and Go/Fe3O4/HybPOM/HPW12 (d). The images indicate the nano compounds after immobilization of HPW12 preserved the relatively uniform spherical nanometer particles of diameter in the range 20–50 nm. EDX analysis (Fig. 7) of HybPOM/HPW12 (a) and Go/Fe3O4/HybPOM/HPW12 (b) nanoparticles show peaks of W, O, Si, C, N, Cu, P and C, O, Fe, W, Si, N, Cu, P respectively, the obtained element percentages of which are fairly consistent with the TGA results.
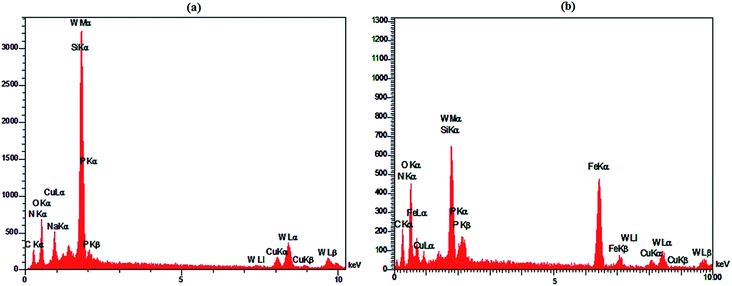 |
| Fig. 6 EDX analysis of HybPOM/HPW12 (a) and Go/Fe3O4/HybPOM/HPW12 nanoparticles (b). | |
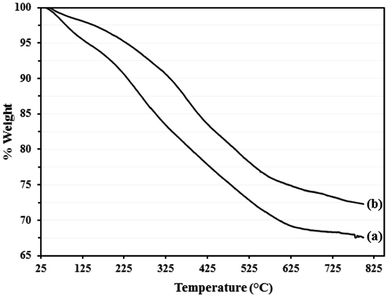 |
| Fig. 7 TGA plot of Go/Fe3O4/HybPOM (a) and Go/Fe3O4/HybPOM/HPW12 (b). | |
TGA was conducted in the range of 30–800 °C and the TGA plot of Go/Fe3O4/HybPOM (a) and Go/Fe3O4/HybPOM/HPW12 (b) are depicted in Fig. 7. Although, the TGA profile of Go/Fe3O4/HybPOM/HPW12 shows continuous weight loss in the range of 30–800 °C, only three weight loss steps cab be observed. The first weight loss (ca. 2.5%) that occurred below 150 °C is related to the loss of physisorbed water at the hybrid material surface. A main weight loss (14.00%) in the range of 150–425 °C is assigned to the decomposition of the Go/Fe3O4/HybPOM nanocomposite support.34 The third weight loss (ca. 8.28%) in the range of 425–600 °C is due to partial decomposition of H3PW12O40 (ref. 29) and residual Go/Fe3O4/HybPOM nanocomposite. The TGA analysis indicates that the prepared Go/Fe3O4/HybPOM/HPW12 is thermally stable at temperatures lower than 150 °C. The total weight loss of Go/Fe3O4/HybPOM/HPW12 (27.7 wt%) was less than that of Go/Fe3O4/HybPOM (32.4 wt%) because of additional residual from partial decomposition of H3PW12O40.30
Recycling experiments for catalysts were performed by the Mannich condensation of benzaldehyde, aniline and cyclohexanone in water. The model reaction was carried out by using 0.01 g of the catalysts. Fig. 8 shows the yield of five consecutive cycles for the preparation of 2-(phenyl(phenylamino)methyl)cyclohexanone in the presence of HybPOM/HPW12 (a) and Go/Fe3O4/HybPOM/HPW12 (b). When the reaction was completed, catalysts are separated and water was removed from the mixture to leave a residue. Then, the product was dissolved in ethyl acetate and the catalyst easily separated from the product by centrifuge or with the aid of an external magnet, followed by decantation of the product solution. The remaining catalysts were washed with ethanol and water to remove the residual product, dried under vacuum and re-used in a subsequent reaction. The catalysts have been observed to be re-usable for at least five times without a detectable catalytic leaching or an appreciable change in activity. High catalytic activity and significantly low leaching of catalysts (during catalytic reactions) were observed by titration of acid content. The potentiometric titration curve of the re-used catalysts showed that all of their acidic sites were preserved during five cycles and HPW12 leaching was negligible (Fig. 4A(c) and B(c)). The FT-IR spectra showed no significant structural changes for catalysts after five consecutive runs (Fig. 9).
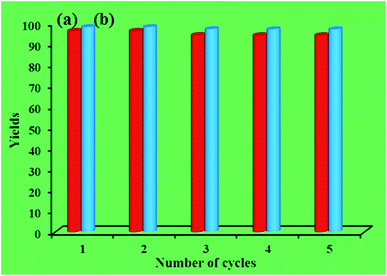 |
| Fig. 8 The yield (%) of five consecutive cycles for the preparation of 2-(phenyl(phenylamino)methyl)cyclohexanone of HybPOM/HPW12 (a) and of Go/Fe3O4/HybPOM/HPW12 (b). | |
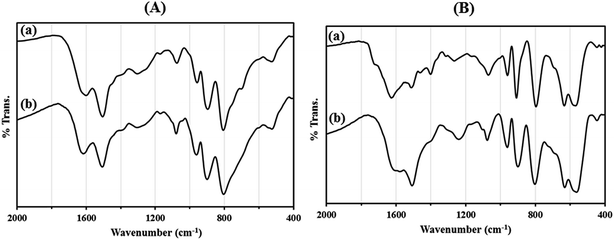 |
| Fig. 9 The FT-IR spectra of (A) HybPOM/HPW12 (a) and HybPOM/HPW12 after five consecutive run (b); (B) Go/Fe3O4/HybPOM/HPW12 (a) and Go/Fe3O4/HybPOM/HPW12 after five consecutive run (b). | |
Catalytic studies
The Mannich-type reactions are very important carbon–carbon bond-forming reactions in organic synthesis and one of the most widely utilized chemical transformations for constructing β-amino ketones and other β-amino carbonyl compounds.46,47 Recently, direct Mannich reactions of aldehydes, ketones and aryl amines have been realized via Lewis acids, lanthanides, transition metal salt catalysis and organocatalytic approaches.47–50 Most of these methods suffer from severe drawbacks including the use of a large amount of catalysts, expensive reagents or catalysts, sometimes long reaction times and low yield. The use of environmentally benign solvents in organic reactions have attracted much attention and another key research area of green chemistry, with great advances being seen in aqueous catalysis. Use of water in addition to its being an environmentally benign solvent show unique selectivity and reactivity. In this study, the catalytic activity of HybPOM/HPW12 and Go/Fe3O4/HybPOM/HPW12 nanoparticles were investigated through one-pot three-component Mannich-type reactions of aldehydes, amines and cyclohexanone in water as a novel, efficient and heterogeneous catalyst under mild conditions. First, in order to optimize the Mannich-type reaction conditions, the reaction was performed with benzaldehyde, aniline and cyclohexanone as a model reaction (Table 1). As shown in Table 1, 0.005 and 0.007 g of catalysts were found to be ideal for the Mannich-type reactions and the best results were obtained using 1 mL water as solvent. The control experiments carried out using homogenous H3PW12O40·xH2O and Go/Fe3O4/HybPOM showed that the mixed Mannich product and imine were formed. When using H3PW12O40·xH2O as a homogenous catalyst, approximately 80% and 15% of the Mannich product and imine were formed, respectively. However, when Go/Fe3O4/HybPOM was used as a catalyst, approximately 65% of the Mannich product was formed. The reaction was extended to a series of aldehydes, cyclohexanone and amines to explore the generality of this catalytic system (Table 2).
Table 1 Optimization of different parameters for the synthesis of 2-(phenyl(phenylamino)methyl)cyclohexanone catalyzed by HybPOM/HPW12 (1) and Go/Fe3O4/HybPOM/HPW12 (2)a
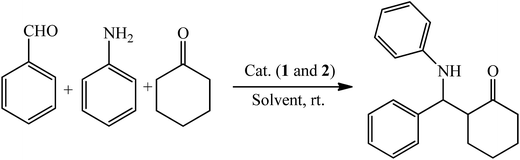
|
Entry |
Cat. (g) |
Solvent |
Time (min) Cat. 1(2) |
Yieldb (%) Cat. 1(2) |
Reaction conditions: aldehyde (1 mmol), amine (1 mmol), ketone (2 mmol). Isolated yield. |
1 |
0 |
H2O |
250(250) |
Trace(trace) |
2 |
0.003 |
H2O |
90(110) |
80(75) |
3 |
0.005 |
H2O |
60(90) |
96(88) |
4 |
0.007 |
H2O |
60(55) |
97(98) |
5 |
0.007 |
EtOH |
120(115) |
89(90) |
6 |
0.007 |
PEG |
70(80) |
70(65) |
7 |
0.007 |
EtOAc |
160(180) |
60(88) |
8 |
0.007 |
CH2Cl2 |
210(200) |
75(85) |
Table 2 One-pot Mannich reactions for the synthesis of β-amino carbonyl compounds catalyzed by HybPOM/HPW12 (1) and Go/Fe3O4/HybPOM/HPW12 (2)
The formed anti and syn isomers in the Mannich-type reactions were identified by the coupling constants (J) of the vicinal protons adjacent to C
O and NH in their 1H NMR spectra. The coupling constant (J) for the anti-isomer is higher than that of the syn. The anti/syn ratio was determined by 1H NMR judged by the intensity of the H1 (Scheme 2).51
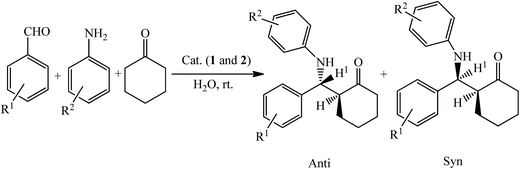 |
| Scheme 2 Mannich reactions of aromatic aldehydes, anilines, and cyclohexanone. | |
A possible mechanism of the Mannich-type reaction in the presence of HybPOM/HPW12 and Go/Fe3O4/HybPOM/HPW12 as Bronsted acid catalysts is proposed (Scheme 3). The reaction proceeded typically through the imine formation of the aldehyde and amine, protonation of the imine, and the attack of the enol derived from the ketone to the protonated imine, leading to the formation of Mannich products.
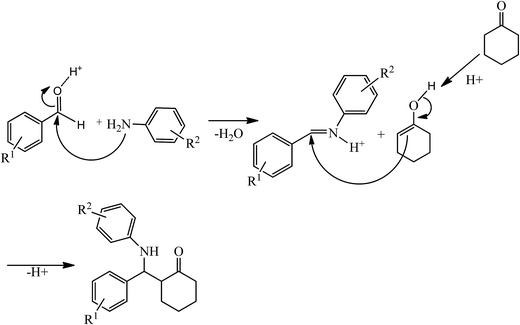 |
| Scheme 3 Proposed mechanism of the Mannich-type reaction in the presence of HybPOM/HPW12 and Go/Fe3O4/HybPOM/HPW12. | |
As shown in Scheme 4, an intermediate are formed via hydrogen bonds formation among Go/Fe3O4/HybPOM/HPW12, the imine and the enol form of cyclohexanone. In the intermediate the aryl groups of aldimine and the methylene groups of cyclohexanone would be anti to each other and there would be less steric repulsion. Therefore, the most stable transition state would produce the anti isomer.52
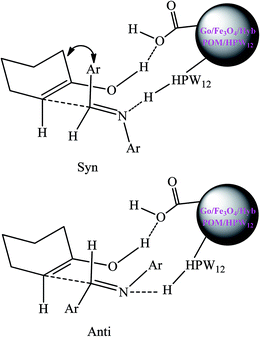 |
| Scheme 4 Proposed syn- and anti-intermediates for the Mannich-type reaction in the presence of Go/Fe3O4/HybPOM/HPW12. | |
4. Conclusion
The new nanoparticle catalysts were synthesized by the immobilization of H3PW12O40 on the surface of H6Cu2[PPDA]6[SiW9Cu3O37]·12H2O (HybPOM) (PPDA = p-phenylenediamine) organic–inorganic hybrid polyoxometalates and Go/Fe3O4/HybPOM nanocomposite. The IR spectroscopy as well as X-ray powder diffraction confirmed immobilization of H3PW12O40 on the supports. Potentiometric titration results show that the new nanocatalysts possessing strong and sufficient acidic sites is responsible for excellent conversion values of products. The nanocatalysts catalyzed one-pot three-component Mannich-type reactions of aldehydes, amines and ketones in water at room temperature and the catalysts were re-used at least five times without any loss of their high catalytic activity.
Acknowledgements
We gratefully acknowledge the Research Council of the University of Kurdistan for supporting this work.
References
- D. L. Long, E. Burkholder and L. Cronin, Chem. Soc. Rev., 2007, 36 Search PubMed.
- M. T. Pope and A. Müller, Angew. Chem., Int. Ed. Engl., 1991, 30, 34 CrossRef.
- P. Kögerler and L. Cronin, Angew. Chem., Int. Ed., 2005, 44, 844 CrossRef.
- T. Yamase, Chem. Rev., 1998, 98, 307 CrossRef CAS PubMed.
- J. T. Rhule, W. A. Neiwert, K. I. Hardcastle, B. T. Do and C. L. Hill, J. Am. Chem. Soc., 2001, 123, 12101 CrossRef CAS PubMed.
- M. V. Vasylyev and R. Neumann, J. Am. Chem. Soc., 2004, 126, 884 CrossRef CAS PubMed.
- N. Mizuno, K. Yamaguchi and K. Kamata, Coord. Chem. Rev., 2005, 249, 1944 CrossRef CAS.
- N. Mizuno and M. Misono, Chem. Rev., 1998, 98, 199 CrossRef CAS PubMed.
- M. Sadakane and E. Steckhan, Chem. Rev., 1998, 98, 219 CrossRef CAS PubMed.
- A. Müller, P. Kögerler and A. W. M. Dress, Coord. Chem. Rev., 2001, 222, 193 CrossRef.
- T. Yamase, K. Fukaya, H. Nojiri and Y. Ohshima, Inorg. Chem., 2006, 45, 7698 CrossRef CAS PubMed.
- J. M. Clemente-Juan and E. Coronado, Coord. Chem. Rev., 1999, 193–195, 361 CrossRef CAS.
- D. L. Long, P. Kogerler, L. J. Farrugia and L. Cronin, Dalton Trans., 2005, 1372 RSC.
- D. L. Long, R. Tsunashima and L. Cronin, Angew. Chem., Int. Ed., 2010, 49, 1736 CrossRef CAS PubMed.
- D. Volkmer, A. Du Chesne, D. G. Kurth, H. Schnablegger, P. Lehmann, M. J. Koop and A. Müller, J. Am. Chem. Soc., 2000, 122, 1995 CrossRef CAS.
- D. W. Fan, X. F. Jia, P. Q. Tang, J. C. Hao and T. B. Liu, Angew. Chem., Int. Ed., 2007, 46, 3342 CrossRef CAS PubMed.
- R. Neumann, Prog. Inorg. Chem., 1998, 47, 317 CrossRef CAS.
- X. M. Yan, J. H. Lei, D. Liu, Y. C. Wu and W. Liu, Mater. Res. Bull., 2007, 42, 1905 CrossRef CAS.
- N. Dubey, S. S. Rayalu, N. K. Labhsetwar and S. Devotta, Int. J. Hydrogen Energy, 2008, 33, 5958 CrossRef CAS.
- M. A. Alibeik, Z. Zaghaghi and I. M. Baltork, J. Chin. Chem. Soc., 2008, 55, 1 CrossRef.
-
(a) T. Okuhara, N. Mizuno and M. Misono, Appl. Catal., A, 2001, 222, 63 CrossRef CAS;
(b) E. Rafiee, S. Eavani, S. Rashidzadeh and M. Joshaghani, Inorg. Chim. Acta, 2009, 362, 3555 CrossRef CAS.
- E. Rafiee, M. Joshaghani, S. Eavani and S. Rashidzadeh, Green Chem., 2008, 10, 982 RSC.
- Perspectives in Catalysis, ed. Y. Ono, J. M. Thomas and K. I. Zamaraev, Blackwell, London, 1992, p. 341 Search PubMed.
- I. V. Kozhevnikov and K. I. Matveev, Appl. Catal., 1983, 5, 135 CrossRef CAS.
- M. Misono and N. Noriji, Appl. Catal., 1990, 64, 1 CrossRef CAS.
- Y. Izumi, R. Hasebe and K. Urabe, J. Catal., 1983, 84, 402 CrossRef CAS.
- H. Soeda, T. Okuhara and M. Misono, Chem. Lett., 1994, 909 CrossRef CAS.
- M. A. Schwegler, H. van Bekkum and N. Munck, Appl. Catal., 1991, 74, 191 CAS.
- M. A. Wahab, I. I. Kim and C.-S. Ha, Microporous Mesoporous Mater., 2004, 69, 19 CrossRef CAS.
- J. B. Mioc, R. Z. Dimitrijevi, M. Davidovic, Z. P. Nedic, M. M. Mitrovic and P. H. Colomban, J. Mater. Sci., 1994, 29, 3705 CrossRef.
- M. Masteri-Farahania, J. Movassagh, F. Taghavi, P. Eghbali and F. Salimi, Chem. Eng. J., 2012, 184, 342 CrossRef.
- R. Tayebee, M. M. Amini, H. Rostamian and A. Aliakbari, Dalton Trans., 2014, 43, 1550 RSC.
- T. A. Zillillah and Z. Li Ngu, Green Chem., 2014, 16, 1202 RSC.
- R. Khoshnavazi, L. Bahrami and F. Havasi, RSC Adv., 2016, 6, 100962 RSC.
- S. Shyles, V. Schünemann and W. R. Thiel, Angew. Chem., Int. Ed., 2010, 49, 3428 CrossRef PubMed.
- M. B. Gawande, A. K. Rathi, P. S. Branco and R. S. Varma, Appl. Sci., 2013, 3, 656 CrossRef CAS.
- A. L. Simplício, J. M. Clancy and J. F. Gilmer, Int. J. Pharm., 2007, 336, 208 CrossRef PubMed.
- I. V. Kozhevnikov, in Catalysis for Fine Chemical Synthesis, Catalysis by Polyoxometalates 2, ed. E. Derouane, Wiley, New York, 2002 Search PubMed.
- J. Li, S. Zhang, C. Chen, G. Zhao, X. Yang, J. Li and X. Wang, ACS Appl. Mater. Interfaces, 2012, 4, 4991 CAS.
- B. Shen, W. Zhai, M. Tao, J. Ling and W. Zheng, ACS Appl. Mater. Interfaces, 2013, 5, 11383 CAS.
- F. Shahbazi and K. Amani, Catal. Commun., 2014, 55, 57 CrossRef CAS.
- W. E. Farneth and R. J. Gorte, Chem. Rev., 1995, 95, 615 CrossRef CAS.
- R. Cid and G. Pecci, Appl. Catal., 1985, 14, 15 CrossRef CAS.
- L. R. Pizzio and M. N. Blanco, Appl. Catal., A, 2003, 255, 265 CrossRef CAS.
- X. Yang, X. Zhang, Y. Ma, Y. Huang, Y. Wang and Y. Chen, J. Mater. Chem., 2009, 19, 2710 RSC.
- G. Pandey, R. P. Singh, A. Garg and V. K. Singh, Tetrahedron Lett., 2005, 46, 2137 CrossRef CAS.
- L. Wang, J. Han, J. Sheng, H. Tian and Z. Fan, Catal. Commun., 2005, 6, 201 CrossRef CAS.
- H. Ishitani, M. Ueno and S. Kobayashi, J. Am. Chem. Soc., 1997, 119, 7153 CrossRef.
- B. List, P. Pojarliev, W. T. Biller and H. J. Martin, J. Am. Chem. Soc., 2002, 124, 12964 CrossRef.
- M. A. Bigdeli, M. M. Heravi, F. Nemati and G. H. Mahdavinia, ARKIVOC, 2008, 243–248 CAS.
-
(a) T. P. Loh, S. B. K. W. Liung, K. L. Tan and L. L. Wei, Tetrahedron, 2000, 56, 3227 CrossRef CAS;
(b) C. Gennari, I. Venturini, F. Gislon and G. Schimperma, Tetrahedron Lett., 1987, 28, 227 CrossRef CAS;
(c) G. Guanti, E. Narisano and L. Ban, Tetrahedron Lett., 1987, 28, 4331 CrossRef CAS;
(d) T. Ollevier and E. J. Nadeau, J. Org. Chem., 2004, 69, 9292 CrossRef CAS PubMed.
- H. Wu, Y. Shen, Y. Fan, P. Zheng, C. Chen and W. Wang, Tetrahedron, 2007, 63, 2404 CrossRef CAS.
- H. Wu, X. M. Chen, Y. Wan, L. Ye, H. Q. Xin, H. H. Xu, C. H. Yue, L. I. Pang, R. Ma and D. Shi, Tetrahedron Lett., 2009, 50, 1062 CrossRef CAS.
- G. Zhang, Z. Huang and J. Zou, Chin. J. Chem., 2009, 27, 1967 CrossRef CAS.
- M. B. Gawande, P. S. Branco, A. Velhinho, I. D. Nogueira, C. A. A. Ghumman and O. M. N. D. Teodorod, RSC Adv., 2012, 2, 6144 RSC.
- E. Rafiee, S. Eavani, F. Khajooei Nejad and M. Joshaghani, Tetrahedron, 2010, 66, 6858 CrossRef CAS.
- Y. Y. Yang, W. G. Shou and Y. G. Wang, Tetrahedron, 2006, 62, 10079 CrossRef CAS.
- A. R. Massah, R. J. Kalbasi and N. Samah, Bull. Korean Chem. Soc., 2011, 32, 1703 CrossRef CAS.
- Z. Li, X. Ma, J. Liu, X. Fang, G. Tian and A. Zhu, J. Mol. Catal. A: Chem., 2007, 272, 132 CrossRef CAS.
- P. Vadivel, C. S. Maheswari and A. Lalitha, Journal of Innovative Technology and Exploring Engineering, 2013, 2, 267 Search PubMed.
Footnote |
† Electronic supplementary information (ESI) available. See DOI: 10.1039/c6ra27519b |
|
This journal is © The Royal Society of Chemistry 2017 |