DOI:
10.1039/C6RA27069G
(Paper)
RSC Adv., 2017,
7, 4872-4875
Synthesis of homochiral zeolitic metal–organic frameworks with amino acid and tetrazolates for chiral recognition†
Received
21st November 2016
, Accepted 3rd January 2017
First published on 17th January 2017
Abstract
By mixing amino acids and tetrazolate ligands, a series of homochiral zeolitic metal–organic frameworks (ZMOFs) with ABW topology have been synthesized, and these materials show permanent microporosity and potential enantioselective recognition ability.
Introduction
Within the last few years, inorganic zeolites and zeolitic metal–organic frameworks (ZMOFs) have received much attention due to their fascinating tetrahedral structures, high stability, and tailored functionalities.1–5 Among them, the combination of the tetrahedrally coordinated divalent cations (M2+ = Zn2+ or Co2+) and uninegative azolate ligands (especially imidazolate and tetrazolate derivatives) results in the successful development of zeolitic imidazolate frameworks (ZIFs) and tetrahedral tetrazolate frameworks (TTFs).4–7 TTFs7 possess uncoordinated N-heteroatom sites from the μ2-tetrazole ligands, and show promising potential applications in carbon dioxide capture and separation.
As enantioselective separation of racemates is especially important for the chemical and pharmaceutical industries, homochiral MOFs with such a function have attracted a great deal of attention in recent years.8,9 The most reliable approach to prepare homochiral MOFs is to select a enantiopure ligand as the primary linker to impart homochirality to the framework. The natural amino acids are ideal candidates because of their low cost and strong coordination ability. So far, although tremendous efforts have been devoted to the design and synthesis of novel homochiral MOFs based on optically pure amino acids and their derivatives,10,11 it remains highly challenging to construct homochiral MOFs with zeolitic topology. Recently, we have developed one kind of homochiral ZMOFs based on enantiopure amino acids ligand.11 By choosing a pair of enantiopure amino acids (L-Alanine and D-Alanine) as linkers and octahedral Ni(II) metal centers as 4-connected nodes, two homochiral MOFs with SOD topology are successfully synthesized. However, the short Ni⋯Ni distance made it a dense framework without pore.
In this work, we report a new approach to construct microporous homochiral ZMOFs based on enantiopure amino acids and tetrazolate derivatives. We select four enantiopure amino acids (L-lanine (L-Ala)), D-lanine (D-Ala), L-serine (L-Ser), and (L-valine (L-Val)) in combination with 5-methyltetrazole (5-Hmtz) ligand (Scheme 1) to realize four homochiral ZMOFs Zn4(5-mtz)6(L-Ala)2·2(DMF) (1L), Zn4(5-mtz)6(D-Ala)2·2(DMF) (1D), Zn4(5-mtz)6(L-Ser)2·2(DMF) (2L) and Zn4(5-mtz)6(L-Val)2·2(DMF) (3L) with ABW topology for the first time. Furthermore, these materials show permanent microporosity and enantioselective recognition ability.
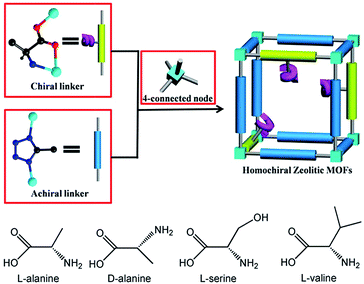 |
| Scheme 1 The synthesis strategy of the homochiral ZMOFs. | |
Experimental
Measurements
Elemental analyses of C, H and N were measured on a Vario MICRO E III elemental analyzer. The IR spectra (KBr pellets) were recorded on a Magna 750 FT-IR spectrophotometer. Powder X-ray diffraction data were recorded on a Rigaku MultiFlex diffractometer with a scan speed of 5° min−1. Thermal stability studies were carried out on a NETSCHZ STA-449C thermoanalyzer under N2 (room temperature-600 °C range) at a heating rate of 10 °C min−1. The gases adsorption isotherms were measured by using ASAP-2020 volumetric adsorption equipment. Circular dichroism (CD) experiments were recorded with a Bio-logic MOS-450 CD Spectrometer at room temperature.
Synthesis
Colorless crystals of 1L were solvothermally synthesized by the self-assembly of Zn(NO3)2·6H2O, 5-Hmtz and L-alanine in DMF solvent. 1D, 2L and 3L were obtained by using D-alanine, L-serine, and L-valine respectively to replace the L-alanine under the similar condition. The details of the synthesis of 1L, 1D, 2L and 3L were described in the ESI.†
Crystallography
Crystallographic data of 1L, 1D and 2L were collected on a Mercury single crystal diffractometer equipped with graphite-monochromatic Mo Kα radiation (λ = 0.71073 Å) at room temperature. Crystallographic data of 3L was collected on a SuperNova (Cu) single crystal diffractometer equipped with graphite-monochromatic Cu Kα radiation (λ = 1.54184 Å) at room temperature. The structures were solved by direct methods and refined by full-matrix least-squares on F2. All the structures were solved by using the program package SHELX-2014/7.12 Non-hydrogen atoms were refined anisotropically, and all hydrogen atoms bond C were generated geometrically. Crystallographic data and structural refinement details for 1L, 1D, 2L and 3L are listed in Table 1. The starting amino acids are not optically pure, resulting in the high Flack values.13 CCDC 1511625 (1D), 1511626 (2L), 1511627 (1L) and 1511628 (3L) contain the ESI† crystallographic data for this paper.
Table 1 Crystallographic data and structural refinement details for 1L, 1D, 2L and 3L
|
1L |
1D |
2L |
3L |
Formula |
C24H46N28O7Zn4 |
C24H46N28O7Zn4 |
C24H44N28O8Zn4 |
C28H52N28O6Zn4 |
Formula weight |
1100.45 |
1100.45 |
1114.43 |
1138.54 |
T/K |
293(2) |
293(2) |
293(2) |
293(2) |
Crystal system |
Monoclinic |
Monoclinic |
Monoclinic |
Monoclinic |
Space group |
P21 |
P21 |
P21 |
P21 |
a/Å |
10.185(4) |
10.175(4) |
10.110(5) |
10.2303(3) |
b/Å |
21.488(9) |
21.481(10) |
21.362(10) |
21.4402(6) |
c/Å |
10.501(5) |
10.493(5) |
10.475(5) |
11.1573(4) |
α/° |
90 |
90 |
90 |
90 |
β/° |
93.174(8) |
93.144(9) |
93.970(6) |
91.259(3) |
γ/° |
90 |
90 |
90 |
90 |
V/Å3 |
2294.7(17) |
2290.0(18) |
2256.8(19) |
2446.65(13) |
Z |
2 |
2 |
2 |
2 |
Dcalc/g cm−3 |
1.593 |
1.596 |
1.640 |
1.546 |
μ/mm−1 |
2.137 |
2.142 |
2.176 |
2.810 |
F(000) |
1124.0 |
1124.0 |
1136.0 |
1168.0 |
R1 |
0.0643 |
0.0685 |
0.0733 |
0.0446 |
wR(F2) |
0.1538 |
0.1590 |
0.1810 |
0.1304 |
GOF |
0.965 |
1.046 |
1.019 |
1.017 |
Flack |
0.24(5) |
0.33(5) |
0.21(5) |
0.17(7) |
Results and discussion
Structural description
Single crystal X-ray analysis revealed that four compounds are isostructural. Here, only the structure of 1L is described in detail. 1L crystallizes in the monoclinic system with chiral space group P21. The asymmetric unit of 1L consists of four Zn(II) ions, six 5-mtz, two L-Ala anions and two ordered DMF molecules. There are two kinds of Zn centers (Fig. 1a). One is tetrahedrally coordinated by three N atoms from three 5-mtz and one carboxylate oxygen atom from L-Ala. The other one shows a distorted trigonal bipyramidal configuration with five-coordination, in which two N atoms from two 5-mtz and one amino N atom from L-Ala occupy the three equatorial, and two axial positions are occupied by one N atom from one 5-mtz and one carboxylate oxygen atom from L-Ala. The Zn–O bond lengths range from 1.947 to 2.305 Å. The Zn–N bond lengths range from 1.972 to 2.118 Å, respectively. The L-Ala ligand is coordinated to two Zn centers. All the 5-mtz ligands adopt similar coordination mode of 2-methylimidazole, using two N atoms to coordinate two Zn atoms. The Zn⋯Zn distances linked by 5-mtz ligands range from 6.036 to 6.227 Å. In comparison, short Zn⋯Zn distances linked by L-Ala ligands ranging from 5.189 to 5.199 Å are observed. All the Zn atoms were linked by 5-mtz ligands to form a layer (Fig. 2c), which can be simplified as a 3-connected (63) net. The L-Ala ligands further link adjacent layers to form a 3D framework (Fig. 1b). Notably, a remarkably structural feature in 1L is the presence of three-dimensional 4-connected ABW network. As mentioned above, both of the L-Ala and 5-mtz act as μ2-bridging ligands. Each Zn center can be successfully reduced as a 4-connected node. As a result, the whole framework can be topologically represented as a uninodal 4-connected zeotype ABW topology by considering L-Ala and 5-mtz as simple linkers (Fig. 2a and d). Two kinds of channels along the a-axis are observed in the structure of 1L. The small channel is from the typical double zigzag chain (DZC) which is well known in zeolite structures (Fig. 2b). The large channel with dimension of ca. 5.5 × 11.2 Å are filled by the structurally ordered DMF molecules. Strong N–H⋯O hydrogen bonding interactions (dN–O = 2.753 Å and 2.945 Å) are observed between the amino ligand and the DMF guest (Fig. 1b). The solvent-accessible volume of ca. 32.1% was calculated by the PLATON program.14 The solvent-accessible volume for 2L and 3L were ∼30.7% and ∼30.1%, respectively.
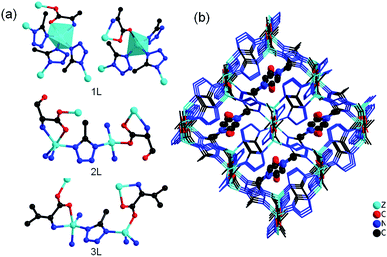 |
| Fig. 1 (a) The coordination environment of metal centers in 1L, 2L and 3L; (b) the 3D framework of 1L with guest molecule DMF (ball and stick) in the pores. | |
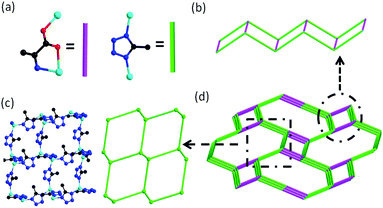 |
| Fig. 2 (a) The simplified L-alanine and 5-methyltetrazole; (b) the DZC chain in 1L; (c) 3-connected net in 1L; (d) the ABW topology of 1L. | |
X-ray powder diffraction and thermal analysis
The powder X-ray diffraction patterns confirm the phase purity of the bulk products and also demonstrate that activated frameworks of 1L, 1D, 2L, 3L remain unchanged after gas sorption measurements (Fig. S1†). Thermal gravimetric analysis (TGA) shows that 1L, 1D, 2L, 3L can be stable up to ca. 230 °C under the N2 atmosphere (Fig. S2†).
Gas absorption measurement
Gas-adsorption measurements of 1L to 3L were performed on a Micromeritics ASAP 2020 surface-area and pore-size analyzer. The samples were activated by solvent exchange with ethanol followed by evacuation at 60 °C for 5 hours. The permanent porosity of 1L to 3L was confirmed by the reversible CO2 sorption measurements at 195 K (Fig. S3†). The Langmuir and BET surface areas of 1L were 302 m2 g−1 and 294 m2 g−1, respectively. The Langmuir and BET surface areas for 2L were 377 m2 g−1 and 364 m2 g−1, respectively. 3L has the largest Langmuir and BET surface areas (672 m2 g−1 and 658 m2 g−1, respectively), which may be assigned to the isopropyl group connected to α-C of amino acid enlarge the channel (Fig. 1).
Chiral recognition of carvone
Since 1L, 1D, 2L and 3L are homochiral frameworks, we are interested in their potential properties on chiral recognition of carvone. A solution of D-carvone or L-carvone in ethanol with the same concentration (10−2 mol L−1) and amount (2 mL) were placed in the cells. Then the same amounts of the as-synthesized samples of 1L, 1D, 2L and 3L were added respectively, and the circular dichroism (CD) signal was recorded. For 1L, 2L and 3L (Fig. 3a, c and d), the CD intensities of solutions decreased with the increased samples, however, the intensity of D-carvone decreased more drastically than that of L-carvone. The result means that the interactions between the samples based on L-amino and D-carvone are stronger than the interactions between the samples based on L-amino and L-carvone. For 1D (Fig. 3b), the intensity of L-carvone decreased more drastically than that of D-carvone, which indicates the stronger interactions between 1D and L-carvone. The samples (about 250 mg) based on L-amino acids exhibit separation ee values of 1L, 2L and 3L are 18.6%, 43.2% and 25.8%, respectively. The largest separation ability of 2L may be attributed to the –OH groups, which promote the interaction between 2L and carvone.
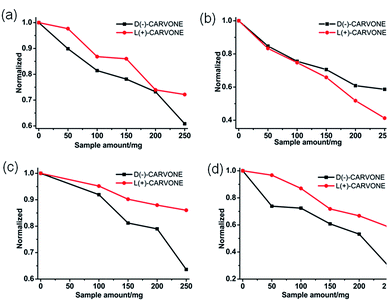 |
| Fig. 3 Enantioselective recognition of carvone in ethanol solutions, (a) 1L; (b) 1D; (c) 2L; (d) 3L. | |
Conclusions
In summary, we first constructed a series of homochiral ZMOFs with ABW topology by employing enantiopure amino acids with tetrazolate ligands. These materials show permanent microporosity and potential enantioselective recognition ability. This work reveals the versatile building strategy of zeolite analogs and opens a new approach toward the synthesis of homochiral ZMOFs by employing natural amino acids.
Acknowledgements
We thank the support for this work from NSFC (21425102, 21521061 and 21573236).
Notes and references
-
(a) M. Eddaoudi, D. F. Sava, J. F. Eubank, K. Adil and V. Guillerm, Chem. Soc. Rev., 2015, 44, 228 RSC;
(b) L. Sun, H. Xing, Z. Liang, J. Yu and R. Xu, Chem. Commun., 2013, 49, 11155 RSC.
-
(a) T. Wu, X. Bu, J. Zhang and P. Feng, Chem. Mater., 2008, 20, 7377 CrossRef CAS;
(b) J.-P. Zhang, Y.-B. Zhang, J.-B. Lin and X.-M. Chen, Chem. Rev., 2012, 112, 1001 CrossRef CAS PubMed;
(c) Y. Liu, V. C. Kravtsov, R. Larsen and M. Eddaoudi, Chem. Commun., 2006, 1488 RSC.
-
(a) H.-X. Zhang, M. Liu, T. Wen and J. Zhang, Coord. Chem. Rev., 2016, 307, 255 CrossRef CAS;
(b) X.-C. Huang, Y.-Y. Lin, J. P. Zhang and X.-M. Chen, Angew. Chem., Int. Ed., 2006, 45, 1557 CrossRef CAS PubMed.
-
(a) J. Zhang, T. Wu, C. Zhou, S. Chen, P. Feng and X. Bu, Angew. Chem., Int. Ed., 2009, 48, 2542 CrossRef CAS PubMed;
(b) J.-P. Zhang, A.-X. Zhu, R.-B. Lin, X.-L. Qi and X.-M. Chen, Adv. Mater., 2011, 23, 1268 CrossRef CAS PubMed;
(c) T. Wu, J. Zhang, C. Zhou, L. Wang, X. Bu and P. Feng, J. Am. Chem. Soc., 2009, 131, 6111 CrossRef CAS PubMed;
(d) R. Banerjee, H. Furukawa, D. Britt, C. Knobler, M. O'Keeffe and O. M. Yaghi, J. Am. Chem. Soc., 2009, 131, 3875 CrossRef CAS PubMed.
-
(a) R. Banerjee, A. Phan, B. Wang, C. Knobler, H. Furukawa, M. O'Keeffe and O. M. Yaghi, Science, 2008, 319, 939 CrossRef CAS PubMed;
(b) B. Wang, A. P. Côté, H. Furukawa, M. O'Keeffe and O. M. Yaghi, Nature, 2008, 453, 207 CrossRef CAS PubMed;
(c) A. Phan, C. Doonan, F. J. Uribe-Romo, C. B. Knobler, M. O'keeffe and O. M. Yaghi, Acc. Chem. Res., 2009, 43, 58 CrossRef PubMed.
-
(a) F. Wang, H.-R. Fu, Y. Kang and J. Zhang, Chem. Commun., 2014, 50, 12065 RSC;
(b) F. Wang, H.-R. Fu and J. Zhang, Cryst. Growth Des., 2015, 15, 1568 CrossRef CAS;
(c) F. Wang, Y.-H. Tang and J. Zhang, Inorg. Chem., 2015, 54, 11064 CrossRef CAS PubMed;
(d) Y.-H. Tang, F. Wang, J.-X. Liu and J. Zhang, Chem. Commun., 2016, 52, 5625 RSC;
(e) M.-Y. Li, F. Wang and J. Zhang, Cryst. Growth Des., 2016, 16, 3063 CrossRef CAS.
-
(a) T. Panda, P. Pachfule, Y. Chen, J. Jiang and R. Banerjee, Chem. Commun., 2011, 47, 2011 RSC;
(b) S. Xiong, Y. Gong, H. Wang, H. Wang, Q. Liu, M. Gu, X. Wang, B. Chen and Z. Wang, Chem. Commun., 2014, 50, 12101 RSC;
(c) S. Xiong, Q. Liu, Q. Wang, W. Li, Y. Tang, X. Wang, S. Hu and B. Chen, J. Mater. Chem. A, 2015, 3, 10747 RSC.
-
(a) Y. Liu, W. Xuan and Y. Cui, Adv. Mater., 2010, 22, 411 CrossRef PubMed;
(b) J. S. Zhao, H. W. Li, Y. Z. Han, R. Li, X. S. Ding, X. Feng and B. Wang, J. Mater. Chem. A, 2015, 3, 12145 RSC;
(c) M. C. Das, Q. Guo, Y. He, J. Kim, C.-G. Zhao, K. Hong, S. Xiang, Z. Zhang, K. Mark Thomas, R. Krishna and B. Chen, J. Am. Chem. Soc., 2012, 134, 8703 CrossRef CAS PubMed.
-
(a) D. B. Dang, P. Y. Wu, C. He, Z. Xie and C. Y. Duan, J. Am. Chem. Soc., 2010, 132, 14321 CrossRef CAS PubMed;
(b) M. M. Wanderley, C. Wang, C.-D. Wu and W. Lin, J. Am. Chem. Soc., 2012, 134, 9050 CrossRef CAS PubMed;
(c) Z.-X. Xu, L. Liu and J. Zhang, Inorg. Chem., 2016, 55, 6355 CrossRef CAS PubMed.
-
(a) R. Vaidhyanathan, D. Bradshaw, J.-N. Rebilly, J. P. Barrio, J. A. Gould, N. G. Berry and M. J. Rosseinsky, Angew. Chem., Int. Ed., 2006, 45, 6495 CrossRef CAS PubMed;
(b) M. Wang, M.-H. Xie, C.-D. Wu and Y.-G. Wang, Chem. Commun., 2009, 2396 RSC.
- E. Yang, L. Wang, F. Wang, Q. Lin, Y. Kang and J. Zhang, Inorg. Chem., 2014, 53, 10027 CrossRef CAS PubMed.
- G. M. Sheldrick, Acta Crystallogr., Sect. A: Found. Crystallogr., 2008, 64, 112 CrossRef CAS PubMed.
- R. E. Marsh, Acta Crystallogr., Sect. B: Struct. Sci., 1999, 55, 931 Search PubMed.
- P. Van der Sluis and A. L. Spek, Acta Crystallogr., Sect. A: Found. Crystallogr., 1990, 46, 194 CrossRef.
Footnote |
† Electronic supplementary information (ESI) available: TGA, PXRD, and additional figures. CCDC 1511625–1511628. For ESI and crystallographic data in CIF or other electronic format see DOI: 10.1039/c6ra27069g |
|
This journal is © The Royal Society of Chemistry 2017 |