DOI:
10.1039/C6RA26999K
(Paper)
RSC Adv., 2017,
7, 22215-22221
A label-free detection of diethylstilbestrol based on molecularly imprinted polymer-coated upconversion nanoparticles obtained by surface grafting†
Received
18th November 2016
, Accepted 29th January 2017
First published on 21st April 2017
Abstract
Herein, we report a novel fluorescent sensor based on molecularly imprinted polymer-coated upconversion nanoparticles (MIP-coated UCNPs) for the recognition and detection of diethylstilbestrol (DES). We used one-step process to modify the surface of the UCNPs with alkenyl groups, which was not only a simple process, but also greatly saved the preparation time. Then, a molecularly imprinted polymer was synthesized on the UCNPs by the surface-graft molecular imprinting method. MIP-coated UCNPs combine the advantages of UCNPs and molecularly imprinted polymers such as strong fluorescence properties, excellent stability, reusability, good adsorption capacity, and high specificity. After the removal of the template molecules, the UCNPs were able to selectively recognize DES, and the fluorescence intensity decreased as the concentration of DES increased. A good linear relationship was obtained in the concentration range of 50–1000 ng mL−1 with the correlation coefficient of 0.9989, and the detection limit was 12.8 ng mL−1 (S/N = 3). MIP-coated UCNPs could be applied for the detection of DES in real milk samples, and the average recoveries for five different concentrations ranged from 90.3% to 107.8%, with the relative standard deviations (RSD) below 2.7%. This fluorescence sensor provides a more convenient, simple and highly specific method for the on-field detection of DES and is promising for a wide range of applications in the future.
Introduction
As a type of synthetic estrogen medication, diethylstilbestrol (DES) is widely used as a growth stimulant in animals and for the prevention and treatment of pregnancy complications.1,2 In recent years, the carcinogenic and toxicological effects of DES in animals and human beings have attracted widespread concern.3–6 Humans may consume DES in many ways, especially, through meat products and milk. Therefore, it is necessary to develop an on-field fast detection method for DES monitoring to monitor food safety and health.7–9 Various methods for the detection of diethylstilbestrol in food are currently available. Gas/liquid chromatography-tandem mass spectrometry10–12 and immunoassay analytical methods13,14 are sensitive and accurate, but they require complicated sample treatment and are time-consuming. Colloidal-gold strip15,16 is fast and easy to operate, but the immune antibody is difficult to produce and may lose biological activity upon external treatment. With the emergence and development of new technologies, sensors have become a hot topic of research because of their fast response and efficiency.
In the field of sensors, upconversion nanoparticles (UCNPs) and molecularly imprinted polymers (MIPs) are of great interest. Moreover, the rare earth element-doped UCNPs are widely used in biosensing technology.17 The special fluorescence mechanism of UCNPs makes them a superior nanobiolabeled material.18 Compared to other common fluorescent probes, in particular, quantum dots (QDs),19,20 UCNPs present significant advantages such as high luminescence, no toxicity towards organisms, narrow absorption and emission peaks, long lifetime, and virtually zero interference background.21 MIPs, as an affinity medium, have the predetermined recognition ability for the imprinted template compound.22 As a highly selective solid-phase extraction material, MIPs are widely used in chromatographic separations, chemo/biosensing, catalysis, and other fields.23,24 Moreover, compared with natural antibodies, MIPs, as an attractive material, not only have a low cost and good stability but also can be directly synthesized.25 Based on these considerations, a label-free, sensitive, stable sensor for the on-field fast detection of DES in food was developed by combining the fluorescence characteristics of UCNPs and the selective recognition ability of MIPs.
Our research team has been studying the detection of estrogen by UCNPs and MIPs for a long time. To date, there have been a few reports on the combination of UCNPs and MIPs for sensing.26,27 In these reports, template monomers and functional monomers were combined by covalent bonding. Considering that covalent bonding between template and functional monomer can cause difficulty in target removing, which leads to lower adsorption capacity, we fabricated MIPs via non-covalent bonding and prepared MIPs-coated UCNPs for the fluorescence sensing of DES. The detection method based on this sensor has low toxicity and a good reproducibility, but the modification of UCNPs is a bit complex and the detection range is narrow. Therefore, it needs further improvement.
In this study, we modified the surface of UCNPs with alkenyl groups by a one-step method, which was not only simple, but also greatly saved preparation time. The imprinted layer on the surface of the UCNPs was synthesized by the surface graft molecularly imprinting method. The imprinted layer could selectively and sensitively recognize the target, and experiments showed that the polymers retained the properties of stability and repeatability. Moreover, the adsorption capacity of this material was adequate, and the adsorption rate was relatively fast, such that in 40 min, an adsorption equilibrium could be achieved. This unique sensor was also demonstrated to be an efficient and easy to operate system for the selective detection of DES in milk.
Experimental
Reagents
All the reagents used herein were of at least analytical grade. YbCl3·6H2O (99.99%), YCl3·7H2O (99.99%), ErCl3·6H2O (99.9%), 1-octadecene (ODE), tetraethoxysilane (TEOS), and 3-(methacryloyloxy)propyl] trimethoxysilane (MPS) were purchased from Sigma-Aldrich (Shanghai, China). Oleic acid (OA), methacrylic acid (MAA), NaOH, and NH4F were purchased from Xiya Chemical Reagent (Shandong, China). Ethanol, methanol, glacial acetic acid (HAc), cyclohexane, ammonia (25%), acetonitrile, and toluene were purchased from Tianjin Fuyu Co. Ltd. (Tianjin, China). Ethylene glycol dimethacrylate (EGDMA), 2,2′-azobisisobutyronitrile (AIBN) (2,2′-azobis(2-methylpropionitrile)), diethylstilbestrol (DES), estradiol (E2), hexestrol (HEX), dienestrol (DIEN), and bisphenol A (BPA) were purchased from J&K Chemical Ltd. (Beijing, China). Millipore Milli-Q ultrapure water (Millipore, 18.2 MΩ cm) was prepared in our laboratory.
Apparatus
Ultraviolet-visible (UV-vis) spectra were obtained over 200–500 nm using a TU-1901 UV-vis spectrophotometer (Beijing Purkinje General Instrument Co. Ltd). X-ray diffraction (XRD) patterns were obtained using a D/max-2500 diffractometer (Rigaku, Japan). The morphology of the prepared samples was investigated by transmission electron microscopy (JEM-2010FEF, JEOL, Japan). Fourier transform infrared (FT-IR) spectra (4000–400 cm−1) were obtained by a FTS6000 spectrometer (Bio-rad, America) using KBr pellets. Fluorescence measurements were performed using an F-4500 fluorescence spectrophotometer (Hitachi, Japan) with an external 980 nm laser diode (Hi-TechOptoelectronic Co. Ltd, China) as the excitation source. Thermogravimetric analysis (TGA) was performed using a TG209 DSC204 DMA242 TMA202 (NETZSCH, Germany).
Synthesis of NaYF4:Yb,Er UCNPs
UCNPs were synthesized according to a previously reported method with a modified procedure.28 YbCl3·6H2O (0.18 mmol), YCl3·7H2O (0.80 mmol), and ErCl3·6H2O (0.02 mmol) were mixed with 10 mL oleic acid (OA) and 30 mL 1-octadecene (ODE) in a 100 mL flask. The mixture was heated to 160 °C for 30 min to form a uniform solution, oxygen and water were removed, and then the solution was cooled down to 50 °C. To this solution, 20 mL of a methanol solution containing NH4F (8 mmol) and NaOH (5 mmol) was added dropwise and stirred at 50 °C for 1 h. The solution was heated to 100 °C for 20 min to distill methanol, fast-heated to 320 °C, and then maintained at this temperature for 1 h under a N2 atmosphere. When reaction was complete, the resultant solution was allowed to naturally cool down and the UCNPs were obtained by centrifugation. The resultant mixture was washed four times with cyclohexane/ethanol mixture (1
:
1 v/v).
Silica coating of UCNPs and surface functionalization with vinyl groups by a one-step process
MPS-UCNPs@SiO2 were synthesized on the basis of previously reported method with a modified procedure.29,30 In a 250 mL round bottom flask, 100 mg of UCNPs were uniformly dispersed in 60 mL of ethanol. Then, 2.5 mL NH3·H2O, 20 mL ultrapure water, and 25 μL TEOS were added. The solution was stirred at 40 °C for 5 h. Subsequently, 30 μL HAc and 1.5 mL MPS were added and mixture was kept at 40 °C for 6 h under stirring. MPS-UCNPs@SiO2 were obtained by centrifugation, and washed four times with ethanol.
Synthesis of MIP-coated UCNPs
MIP-coated UCNPs were prepared as follows. MAA (1.8 mmol) and DES (0.3 mmol) were added to 41 mL acetonitrile. The mixture was slowly stirred using a magnetic stirrer for 24 h at room temperature to form the template–monomer complex. Modified UCNPs (60 mg), the free-radical initiator AIBN (30.0 mg), the cross-linkers EGDMA (3.0 mmol), and toluene (11 mL) were dissolved in the template–monomer solution in a 250 mL flask. Then, the flask was sealed under N2 and stirred under reflux in an oil bath at 45 °C for 5 h, then at 60 °C for 1 day, and finally at 80 °C for 2 h. After polymerization, MIP-coated UCNPs were obtained by centrifugation and washed several times with methanol
:
HAc (9
:
1, v/v) until templates could not be detected by UV-vis spectrophotometry. The non-imprinted polymers (NIPs) were prepared by the same method but in the absence of the template molecule DES.
Fluorescence measurements
Herein, the F-4500 fluorescence spectrometer (excitation power: 230 mW; excitation and emission slits: 1 nm) was used to detect all the fluorescence intensity and the emission intensity of 544 nm was chosen as quantitative standard. In 4 mL of acetonitrile, 2 mg MIP-coated UCNPs were dispersed and completely mixed. Then, 60 μL of this dispersion was added to 4.0 mL of acetonitrile solutions with different concentrations of DES; the fluorescence spectra for each concentration were obtained after oscillating the sample for 40 min at room temperature.
Characterization of the MIP-coated UCNPs
For the equilibrium binding experiments, 2 mL of each concentration of DES acetonitrile solution was added to 5 mg MIP-coated UCNPs or NIP-coated UCNPs. The mixture was shaken using a mediation oscillator at room temperature for 4 h. A UV-vis spectrophotometer was used to investigate the supernatant.
For the rebinding kinetics experiments, 10 mg of MIP-coated UCNPs or NIP-coated UCNPs and 5 mL of DES acetonitrile solution with a concentration of 100 μg mL−1 were mixed in a 7 mL Eppendorf tube. The mixed solution was shaken for different times (0, 5, 10, 15, 20, 30, 40, 50, and 60 min) at room temperature, and the supernatant was analyzed by a UV-vis spectrophotometer.
The selectivity test was carried out by detecting DIEN, HEX, BPA, and E2 as analogues of DES at different concentrations (0, 100, 200, 400, 600, 800, and 1000 ng mL−1). The measurements were conducted by the same method as the one detailed in “Fluorescence measurements”.
Detection of DES in real samples
Milk samples used in this study were purchased from a local supermarket and were analyzed to ensure that they did not contain any DES. The pre-treatment method was based on a previous report.31 Milk (1 mL) was placed in a centrifuge tube. DES was added at five different concentrations: 60, 120, 240, 480, and 960 ng mL−1. Then, the mixture was extracted with an equal volume of ethyl acetate and left to stand until stratification. The supernatant was collected and dried under N2. Finally, the residue was redissolved in acetonitrile for analysis.
Results and discussion
Preparation and characterization of MIP-coated UCNPs
Scheme 1 illustrates the DES identification mechanism and the synthetic procedure for MIP-coated UCNPs. The molecularly imprinted polymer was formed by a simple method of polymerization on the outer surface of the UCNPs. First, UNCPs with a uniform dimension were synthesized via a solvent thermal process. Then, the surface of the UCNPs was modified with alkenyl groups by a one-step process, which greatly saved preparation time. Before polymerization, DES and the functional monomer MAA were mixed in the ratio of 1
:
6 (ref. 27) in acetonitrile for 12 h, such that the carboxyl groups of MAA and the hydroxyl groups of DES could form the pre-polymer via hydrogen bonds. Then, the cross-linking agent (EGDMA) was added, and the polymerization occurred between the double bonds under the action of the initiator AIBN, and the imprinted polymeric layer was formed on the surface of the UCNPs. Finally, the synthesized product was eluted with methanol
:
HAc (9
:
1, v/v) to break the hydrogen bonds between the template and the monomer and remove the template. This way, specific recognition sites were formed in the imprinted cavities.
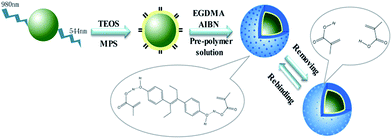 |
| Scheme 1 Schematic of the preparation procedure for MIP-coated UCNPs. | |
The morphology of MIP-coated UCNPs was characterized by TEM. As it can be seen in Fig. 1a, the UCNPs were hexagonal with a uniform size (100–110 nm). Fig. 1b shows the TEM image of silica-coated UCNPs, and it could be observed that there was a thin shell (3 nm) on the surface of the UCNPs. In the TEM image of MIP-coated UCNPs, shown in Fig. 1c, a shell of about 8 nm could be clearly observed, indicating that core–shell UCNPs were obtained. Fig. S1† shows the TGA results for UCNPs and MIP-coated UCNPs. The decomposition of MIPs leads to a quick weight loss (16%) from 350 °C to 800 °C. The curves indicated that H2O and oleic acid adsorbed on the surface of UCNPs were about 4% and the amount of MIPs coating the surface was about 12%. Thus, these results demonstrated that the coating procedure was successful.
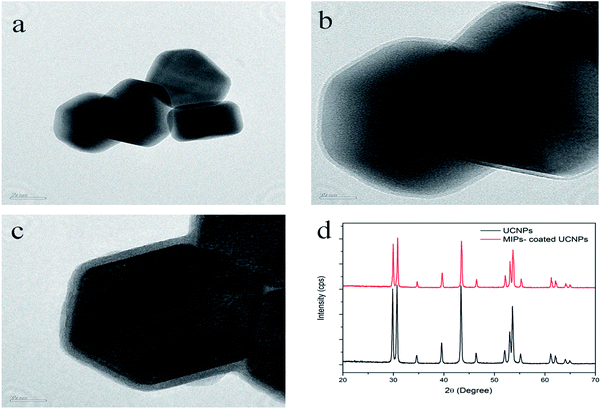 |
| Fig. 1 TEM image of UCNPs (a), MPS-UCNPs@SiO2 (b), and MIP-coated UCNPs (c); (d) XRD patterns of UCNPs and MIP-coated UCNPs. | |
The XRD patterns of UCNPs and MIP-coated UCNPs are shown in Fig. 1d. The XRD spectrum of UCNPs showed a crystalline β phase, and it was in good agreement with the JCPDS card 28-1192. The XRD pattern of UCNPs was similar to that of MIP-coated UCNPs, thus proving that the crystalline phase of UCNPs did not change after coating with MIPs. Moreover, due to the MIP layer surface, the XRD pattern of MIP-coated UCNPs had a lower response, which demonstrated that the MIP layer was successfully coated onto the UCNPs.
To further confirm the surface modification procedures, the products were characterized by FT-IR spectrometry. Fig. 2a shows the IR spectrum of OA-UCNPs without any modification. The absorption peak at 1703 cm−1 was attributed to the stretching vibrations of the carbonyl group (C
O). The two peaks at 2926 cm−1 and 2856 cm−1 were ascribed to the methylene groups (–CH2–) of oleic acid. In the IR spectrum of MPS-UCNPs@SiO2, shown in Fig. 2b, we could see a strong peak at 1090 cm−1 and two small peaks at 808 cm−1 and 942 cm−1, corresponding to the symmetrical stretching vibration and bending vibration of the silicon–oxygen bond (Si–O), respectively. The characteristic peaks at 1716 cm−1 and 1636 cm−1 were attributed to the stretching vibrations of the carbonyl group (C
O) and the carbon–carbon double bond (C
C) of MPS. Thus, it was verified that the UCNPs were successfully encapsulated by a silica shell and modified with MPS. After coating with a MIP layer, the IR spectrum, as shown in Fig. 2c, revealed two peaks at 2925 cm−1 and 2955 cm−1, corresponding to the bending vibrations of methylene (–CH2–) and methyl groups (CH3–), respectively. The absorption peaks at 1722 cm−1 and 1163 cm−1 were attributed to the stretching vibrations of the carbonyl group (C
O) and ether group (C–O–C), respectively. The identifications of the peaks of IR spectra are described in Table S1.†
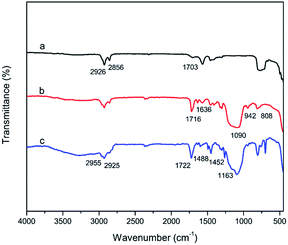 |
| Fig. 2 FT-IR spectra of UCNPs (a), MPS-UCNPs@SiO2 (b), and MIP-coated UCNPs (c). | |
Equilibrium binding
The static adsorption experiments of the MIPs-coated UCNPs (NIPs-coated UCNPs) to DES were carried out by employing 5 mg of the MIPs-coated UCNPs (NIPs-coated UCNPs) incubated with DES acetonitrile solution (10, 20, 40, 60, 80, 100, and 120 μg mL−1) for 3 h. Fig. 3a shows that the adsorption capacity Q (mg g−1) gradually increased as the DES concentration increased. The Q of NIP-coated UCNPs was significantly lower than that of MIP-coated UCNPs. The adsorption of MIPs-coated UCNPs and NIPs-coated UCNPs tended to be saturated at the concentration of 100 μg mL−1 and the adsorption capacity of MIP-coated UCNPs was significantly higher than that of NIP-coated UCNPs. The equilibrium binding isotherm was obtained by Langmuir fitting of the data. Fig. 3c shows the Langmuir adsorption isotherm, and the equation was CDES/Q = 0.000127CDES + 0.00354. The maximum adsorption capacity (Qmax) is 7.87 mg g−1. The greater adsorption capacity provided a foundation to achieve a wider detection range, which avoided gradient dilutions during real sample monitoring.
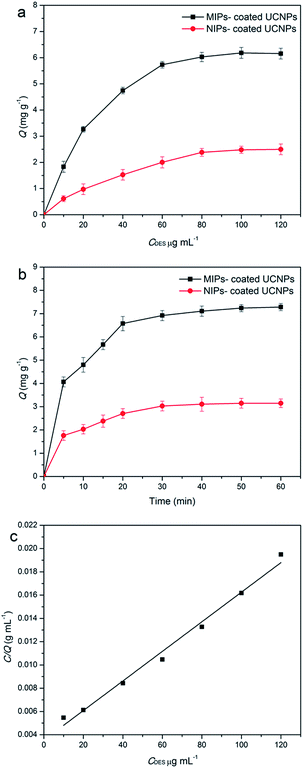 |
| Fig. 3 (a) Adsorption isotherms of MIP-coated UCNPs and NIP-coated UCNPs at 30 °C. (b) Kinetic uptake plot of MIP-coated UCNPs and NIP-coated UCNPs at 30 °C. (c) Langmuir plot of MIP-coated UCNP Q versus DES concentration. | |
To demonstrate the kinetic adsorption process of MIP-coated UCNPs and NIP-coated UCNPs, experiments were conducted by incubating 10 mg of MIP-coated UCNPs or NIP-coated UCNPs in a DES acetonitrile solution (5 mL, 100 μg mL−1) for different time periods. The results, as shown in Fig. 3b, suggested that MIP-coated UCNPs had a quick binding rate in 20 min and the adsorption capacity was close to the maximum value. However, we observed that for MIP-coated UCNPs, the adsorption towards DES was low within the 20 to 40 min period. Finally, we chose 40 min as the response time to ensure that MIP-coated UCNPs adsorb as much DES as possible. The adsorption rate of MIP-coated UCNPs was significantly higher than that previously reported,32 which was attributed to the surface graft molecularly imprinting method. The higher adsorption rate meant that MIP-coated UCNPs have promising applications in on-field detection.
Fluorescence optosensing of DES based on MIP-coated UCNPs
To acquire the standard curve, MIP-coated UCNPs were respectively added to a series of concentrations of DES solutions, and then the fluorescence response spectra were obtained. According to the kinetic adsorption experiment described above, 40 min was considered to be the optimal incubation time for MIP-coated UCNPs with DES. As shown in Fig. 4, within the DES concentration range of 50–1000 ng mL−1, the fluorescence intensity of MIP-coated UCNPs decreased as the concentration of DES increased, showing a good linear relationship. The quenching mechanism might be attributed to the charge transfer from UCNPs to DES.33 The DES absorption peak (Fig. S2a†) and the UCNPs emission peak (at 541 nm) did not overlap. Therefore, the quenching mechanism is not through fluorescence resonance energy transfer. Upon comparing Fig. S2a with S2b,† it can be observed that the band gap of UCNPs was close to the absorption peak of DES. Therefore, the charge on UCNPs could be transferred to the adsorbed DES, leading to fluorescence quenching. The fluorescence quenching in this system could be described by the Stern–Volmer equation:
where F0 is the initial fluorescence intensity, F is the fluorescence intensity in the presence of DES, KSV (mL ng−1) is the quenching constant, and Cq (μg mL−1) is the concentration of DES. The fluorescence intensity at different concentrations of DES is shown in Fig. 4a. The regression equation is F0/F = 0.00087Cq + 0.0415, with a correlation coefficient of 0.9989. The detection limit was found to be 12.8 ng mL−1 by calculating (S/N = 3).
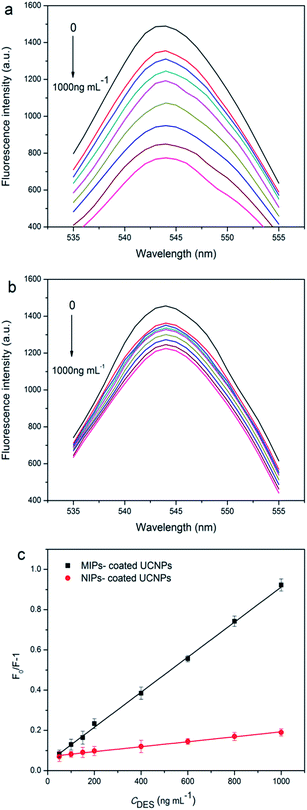 |
| Fig. 4 Fluorescence spectra of MIP-coated UCNPs (a) and NIP-coated UCNPs (b) with different concentrations of DES; (c) Stern–Volmer curves of DES concentration of MIPs-coated UCNPs and NIPs-coated UCNPs. | |
Upon comparing Fig. 4a with Fig. 4b, it could be observed that both MIP-coated UCNPs and NIP-coated UCNPs showed a response to DES. The ratio between the KSV of MIP-coated UCNPs and the KSV of NIP-coated UCNPs was 6.87, as obtained from Fig. 4c, which indicated that the as-synthetized materials had a good specificity towards the target molecule. The main reason for the enhanced response was that MIP-coated UCNPs could match the target molecules much better through the recognition sites. Compared with the results obtained by other procedures for the detection of DES, shown in Table S2,† this procedure was reproducible and without a complicated operation process.
Selectivity of the MIP-coated UCNPs
When DES was removed after elution, specific recognition sites were formed on the imprinted cavities, which could selectively rebind DES. To verify the specificity of this sensor, three structural analogues of DES (DINE, HEX, and BPA) and a functional analogue of DES (E2) were selected (structures shown in Fig. S3†). As shown in Fig. 5, MIP-coated UCNPs showed a good specificity towards DES, and the quenching with the analogues was due to nonspecific adsorption on the imprinted cavities. The KSV value of MIP-coated UCNPs for DES was much larger than that for the structural and functional analogues because of the shape, location, and the size of the binding cavities. In summary, these results confirmed that this sensor has a high selectivity towards DES.
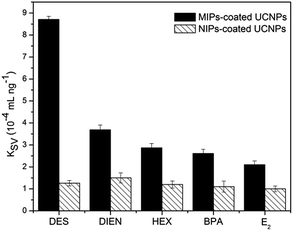 |
| Fig. 5 The Stern–Volmer quenching constants of different analogues with MIPs-coated UCNPs and NIPs-coated UCNPs. | |
Fluorescence repeatability and stability of the MIP-coated UCNPs
The repeatability of the sensor was evaluated by the following method: 5 mg of MIP-coated UCNPs and 2 mL of DES acetonitrile solution with the concentration of 100 μL mL−1 were added to a 5 mL Eppendorf tube and stirred for 4 h at room temperature. The supernatant was analyzed by UV-vis spectrophotometry and MIP-coated UCNPs were obtained by centrifugation and washed three times with methanol
:
HAc (9
:
1, v/v). The abovementioned steps were repeated 7 times. As shown in Fig. S4a,† the MIP-coated UCNPs could still reach 90% of the adsorption capacity after 7 cycles. This result demonstrated that MIP-coated UCNPs could be recycled, thus reducing testing costs.
To investigate the analytical stability of the MIPs-coated UCNPs, the following experiment was designed. The repeated test of the fluorescent response intensity was performed every 10 min. The results, as shown in Fig. S4b,† indicated that the fluorescence response intensity of MIP-coated UCNPs was stable within 60 min. Moreover, the repeated test of the fluorescent response intensity was also performed every day. On the seventh day, MIP-coated UCNPs still retained 95% of its initial response, as shown in Fig. S4c.† This result indicated that MIP-coated UCNPs could be stable for at least 7 days at room temperature. The excellent fluorescence stability of this sensor showed a promising application for the on-field detection of DES.
Applications in the analysis of a milk sample
To further demonstrate the applicability of MIP-coated UCNPs in a real sample, the concentration of DES was determined in milk. The concentrations of spiked DES in milk samples were 60, 120, 240, 480, and 960 ng mL−1. In Table 1, the average recoveries were found to be in the range from 90.3 to 107.8% with RSDs from 1.5 to 2.7%. These results indicated that the developed method was accurate and the pre-processing procedure was simple, demonstrating that the method could be applied in real sample monitoring.
Table 1 Recovery of DES at different concentrations in milk samples (n = 5)
Sample |
Spiking levels (ng mL−1) |
Amount measured (ng mL−1) |
Recovery (%) |
RSD (%) |
1 |
60 |
54.15 |
90.3 |
2.3 |
2 |
120 |
127.07 |
105.9 |
1.5 |
3 |
240 |
235.39 |
98.1 |
1.9 |
4 |
480 |
503.73 |
104.9 |
1.7 |
5 |
960 |
1034.57 |
107.8 |
2.7 |
Conclusion
In summary, a novel procedure for detecting diethylstilbestrol was successfully developed on the basis of MIP-coated UCNPs, which combined the fluorescence properties of NaYF4:Yb]r upconversion nanoparticles with the selectivity of a molecularly imprinted polymer. We deposited a silicon shell and modified the double bond on the surface of the UCNPs by a one-step process and prepared the imprinted layer on the exterior of the UCNPs by the surface graft molecular imprinting method. The synthetic procedure was simple and easy to control. The label-free sensor was considered to have an excellent fluorescence, outstanding stability, good repeatability, high selectivity, and a wide detection range, and could be applied for the analysis of real samples. It was suggested that the simple and reliable preparation strategy of MIP-coated UCNPs could be a great potential method for customizing sensing materials according to the hazardous compounds found in food products.
Acknowledgements
This work was supported by the National Natural Science Foundation of China (No. 21477162, No. AWS15J006), the Science and Technology Planning Projects of Tianjin (No. 14ZCZDSF00021), the National Key Scientific Instrument and Equipment Development Project (No. 2013YQ14037106).
Notes and references
- J. Wang, H. Ye, Z. Jiang, N. Chen and J. Huang, Anal. Chim. Acta, 2004, 508, 171–176 CrossRef CAS.
- S. Zhang, B. Du, H. Li, X. D. Xin, H. M. Ma, D. Wu, L. G. Yan and Q. Wei, Biosens. Bioelectron., 2014, 52, 225–231 CrossRef CAS PubMed.
- D. Ganmaa and A. Sato, Med. Hypotheses, 2005, 65, 1028–1037 CrossRef CAS PubMed.
- D. Ganmaa, H. Tezuka, D. Enkhmaa, K. Hoshi and A. Sato, Int. J. Cancer, 2006, 118, 2363–2365 CrossRef CAS PubMed.
- L. Q. Qin, P. Y. Wang, T. Kaneko, K. Hoshi and A. Sato, Med. Hypotheses, 2004, 62, 133–142 CrossRef CAS PubMed.
- Q. L. Zhang, J. Li, T. T. Ma and Z. T. Zhang, Food Chem., 2008, 111, 498–502 CrossRef CAS PubMed.
- W. Yan, Y. Li, L. Zhao and J. M. Lin, J. Chromatogr. A, 2009, 1216, 7539–7545 CrossRef CAS PubMed.
- K. McMartin, K. Kennedy, P. Greenspan, S. Alam, P. Greiner and J. Yam, J. Environ. Pathol. Toxicol., 1978, 1, 279 CAS.
- J. Król, W. Pobłocki, T. Bockenheimer and P. Hliwa, Aquacult. Int., 2014, 22, 53–62 CrossRef.
- C. L. Ke, Z. H. Wang, J. L. Gan, Y. G. Gu, K. Huang, L. D. Li and Q. Lin, RSC Adv., 2014, 4, 2355–2359 RSC.
- G. Kaklamanos, G. Theodoridis and T. Dabalis, J. Chromatogr. A, 2009, 1216, 8072–8079 CrossRef CAS PubMed.
- F. Guo, Q. Liu, G. B. Qu, S. J. Song, J. T. Sun, J. B. Shi and G. B. Jiang, J. Chromatogr. A, 2013, 1281, 9–18 CrossRef CAS PubMed.
- Z. D. Yan, P. Xiong, N. Gan, J. L. He, N. B. Long, F. T. Hu and T. H. Li, J. Electroanal. Chem., 2015, 736, 30–37 CrossRef CAS.
- J. S. Tang, L. Xiang, F. Zhao, F. K. Pan, S. Y. Wang and X. F. Zhan, Anal. Lett., 2015, 48, 796–808 CrossRef CAS.
- D. Mukunzi, B. N. Tochi, J. Isanga, L. Q. Liu, H. Kuang and C. L. Xu, Food Agric. Immunol., 2016, 27, 855–869 CrossRef CAS.
- Y. Chen, Z. Q. Wang, Z. H. Wang, S. S. Tang, Y. Zhu and X. L. Xiao, J. Agric. Food. Chem., 2008, 56, 2944–2952 CrossRef CAS PubMed.
- L. Cheng, C. Wang and Z. Liu, Nanoscale, 2013, 5, 23–37 RSC.
- L. Wang and Y. Li, Chem. Mater., 2007, 19, 727–734 CrossRef CAS.
- W. Zhang, X. W. He, Y. Chen, W. Y. Li and Y. K. Zhang, Biosens. Bioelectron., 2012, 31, 84–89 CrossRef CAS PubMed.
- D. Y. Li, X. W. He, Y. Chen, W. Y. Li and Y. K. Zhang, ACS Appl. Mater. Interfaces, 2013, 5, 12609–12616 CAS.
- F. Wang and X. Liu, Chem. Soc. Rev., 2009, 38, 976–989 RSC.
- K. Haupt, Anal. Chem., 2003, 75, 376A–383A CrossRef CAS PubMed.
- K. Haupt and K. Mosbach, Chem. Rev., 2000, 100, 2495–2504 CrossRef CAS PubMed.
- A. Poma, A. Guerreiro, M. J. Whitcombe, E. V. Piletska, A. P. Turner and S. A. Piletsky, Adv. Funct. Mater., 2013, 23, 2821–2827 CrossRef CAS PubMed.
- J. Wackerlig and P. A. Lieberzeit, Sens. Actuators, B, 2015, 207, 144–157 CrossRef CAS.
- Y. Tang, Z. Gao, S. Wang, X. Gao, J. Gao, Y. Ma, X. Liu and J. Li, Biosens. Bioelectron., 2015, 71, 44–50 CrossRef CAS PubMed.
- J. Tian, J. Bai, Y. Peng, Z. Qie, Y. Zhao, B. Ning, X. Dan and Z. X. Gao, Analyst, 2015, 140, 5301–5307 RSC.
- Y. Cen, J. Tang, X. J. Kong, S. Wu, J. Yuan and R. Q. Yu, Nanoscale, 2015, 7, 13951–13957 RSC.
- K. Stalder and W. Stöber, Nature, 1965, 207, 874–875 CrossRef CAS PubMed.
- S. Wu, N. Duan, X. Ma, Y. Xia, Y. Yu, Z. Wang and H. Wang, Chem. Commun., 2012, 48, 4866–4868 RSC.
- X. Zhang, Y. Peng, J. Bai, B. Ning, S. Sun, X. Hong, Y. Y. Liu, Y. Liu and Z. X. Gao, Sens. Actuators, B, 2014, 200, 69–75 CrossRef CAS.
- T. Guo, Q. Deng, G. Fang, C. Liu, X. Huang and S. Wang, Biosens. Bioelectron., 2015, 74, 498–503 CrossRef CAS PubMed.
- X. Zhou, P. Ma, A. Wang, C. Yu, T. Qian, S. Wu and J. Shen, Biosens. Bioelectron., 2015, 64, 404–410 CrossRef CAS PubMed.
Footnote |
† Electronic supplementary information (ESI) available: TGA curves of UCNPs and MIPs-coated UCNPs, UV absorption spectra of DES and UCNPs, chemical structures of five analogues, repeatability and stability of MIPs-coated UCNPs, effects of identification of the peak of the IR spectra, comparison between this method and other methods. See DOI: 10.1039/c6ra26999k |
|
This journal is © The Royal Society of Chemistry 2017 |
Click here to see how this site uses Cookies. View our privacy policy here.