DOI:
10.1039/C6RA26933H
(Paper)
RSC Adv., 2017,
7, 5628-5635
Novel UIO-66-NO2@XC-72 nanohybrid as an electrode material for simultaneous detection of ascorbic acid, dopamine and uric acid†
Received
17th November 2016
, Accepted 6th January 2017
First published on 17th January 2017
Abstract
UIO-66-NO2@XC-72 nanohybrid was synthesized from UIO-66-NO2 via hydrothermal synthesis method, followed by mixing with XC-72 carbon (XC-72). The compositions, microstructures, textural parameters and morphologies of the resulting nanomaterials were characterized by FTIR, XRD, N2 adsorption–desorption, SEM and TEM. The UIO-66-NO2@XC-72-modified glassy carbon electrode (GCE) sensor was successfully applied to the simultaneous determination of ascorbic acid (AA), dopamine (DA) and uric acid (UA). Owing to the large surface area of UIO-66-NO2 and good conductivity of XC-72, as well as the hydrogen bond effect between the UIO-66-NO2 and the analyte, the proposed sensor exhibited excellent linear responses to AA, DA and UA under optimized conditions. The detection ranges were 0.2–3.5 μM for AA, 0.03–2.0 μM for DA and 0.75–22 μM for UA, with the detection limits (S/N = 3) of 0.12 μM, 0.005 μM and 0.03 μM, respectively. Moreover, the high reproducibility and stability of the sensor were obtained in all the experiments. The sensor was also successfully applied to the determination of DA in hydrochloride injection solution and UA in urine sample. The MOFs@XC-72 would be potentially applied as a modified electrode sensor material for the determination of other small biomolecules.
1. Introduction
Ascorbic acid (AA), dopamine (DA) and uric acid (UA) usually coexist in human metabolism, renal and central nervous systems, playing important roles in real biological matrixes.1–5 The development of a sensitive and selective method for simultaneous detection of the three small biomolecules is therefore desirable.1,2,5 Electrochemical method possesses the advantages of rapidity, economy, convenience and sensitivity, combined with the high electrochemical activity of AA, DA and UA, thus it is usually adopted to detect the three species.3,4,6 However, the oxidation peaks of the three compounds are broad and overlap using an unmodified electrode. To date, there have been considerable efforts devoted to developing electrode sensor modifiers to promote the electron transfer and achieve high sensitivity and selectivity,7,8 such as carbon nanotubes,9–11 noble metals nanoparticles,12–16 mesoporous carbons,17 conductive polymers,3,18–21 and hybrids.22,23
Recently, metal–organic frameworks (MOFs) have attracted much attention as electrochemical sensor materials for their rich structure types, high surface area, tunable pores and various functionalities.24–29 Nevertheless, most MOFs have the major drawback of their poor stability, which are derived from the reversible nature of the coordination bonds.24,30 Moreover, nano-scale MOFs have more potential applications because they exhibit much higher surface areas, uniform sizes, well-defined functionality, and stable dispersions.31,32 However, numerous of synthesized MOFs are ascribed to the micron-sized materials, resulting in the weakened adhesion properties between MOFs and glassy carbon electrode (GCE). Furthermore, plenty of MOFs belong to poor electrical conductors.27,33,34 To overcome these problems, many nanostructured and conductive MOFs were synthesized and applied in sensors fields.35–37 For poor electron-conductive MOFs, an efficient method is to mix MOFs with conductive materials. UIO-66-R (–H, –NO2, –NH2) were of great interest due to their large surface area, good stability and unique functional groups.38–40 The applications of UIO-66-NO2 and UIO-66-NH2 as catalytic materials were related to the special functional groups, which can easily interact with the analyte, such as hydrogen bond effect, electrostatic effect. Besides, the band gap value (Eg) of UIO-66-R is a very important parameter for electrochemical reaction at sensors.41 According to the theoretical calculations and experiments, the Eg value of UIO-66-R decreased in the following order: –H > –NO2 > –NH2.42 Therefore, nano-UIO-66-NO2 was selected to be modified electrode sensors materials. To enhance the conductivity of the nanohybrid, the conductive XC-72 was mixed with the MOFs.26,43 However, there are a few literatures about UIO-66-NO2@XC-72 to determine AA, DA and UA.
Herein, we provide a strategy to prepare UIO-66-NO2 via hydrothermal synthesis method, followed by mixing with XC-72. When the hybrid was applied to modify the GCE, it was used to simultaneously detect the three compounds. Three well-defined separated peaks were observed by using cyclic voltammetry (CV) and differential pulse voltammetry (DPV) methods. Furthermore, the developed sensor exhibited high sensitivity, selectivity, reproducibility and stability. Besides, the sensor was also suitable to detect the real samples. The wide linear range and low detection limits for the three species were obtained due to the synergistic effect of UIO-66-NO2 and XC-72.
2. Experimental
2.1 Chemicals and apparatus
Zirconium chloride (ZrCl4), 2-nitroterephthalic acid (H2BDC-NO2) and N,N-dimethylformamide (DMF) were purchased from Aldrich. AA, DA and UA were obtained from Alfa Aesar and XC-72 carbon from Cabot Carbon Corporation (USA). Sodium dihydrogen phosphate (NaH2PO4) and disodium hydrogen phosphate (Na2HPO4) were bought from Aldrich. Phosphate buffer solutions (PBS, 0.1 M) were prepared from stock solutions of 0.1 M NaH2PO4, Na2HPO4 and H3PO4. All other reagents were at least analytical grade.
2.2 Preparation of UIO-66-NO2
The UIO-66-NO2 was prepared according to the reported method.44 Briefly, ZrCl4 (0.932 g), H2BDC-NO2 (0.845 g) and 0.67 mL concentrated HCl were added in 10 mL DMF in a Teflon-lined stainless steel autoclave, followed by ultrasonic treatment 5 min. The autoclave was placed in an oven at 120 °C for 16 h, and the mixture was filtered and washed with DMF and acetone, respectively. The final product was activated at 60 °C under a vacuum drying for 10 h.
2.3 Fabrication of modified GCE sensors
Prior to preparation procedure, the GCE was carefully polished with 0.1 and 0.05 μm Al2O3 power. Afterwards, the electrode was sonicated in nitric acid solution (HNO3
:
H2O = 1
:
1), acetone, and double distilled water, and then it was allowed to dry at room temperature. 5.0 mg of the individual samples (UIO-66-NO2, XC-72) or the mixed samples (the ratio of UIO-66-NO2 and XC-72 was 1
:
5, 1
:
6, 1
:
7, 1
:
8, 1
:
9, 1
:
10) were dispersed in 5 mL H2O followed by sonication for 30 min. The modified electrode sensors were prepared by casting 5 μL of the suspension on GCE surface, and dried under an infrared lamp (Scheme 1).
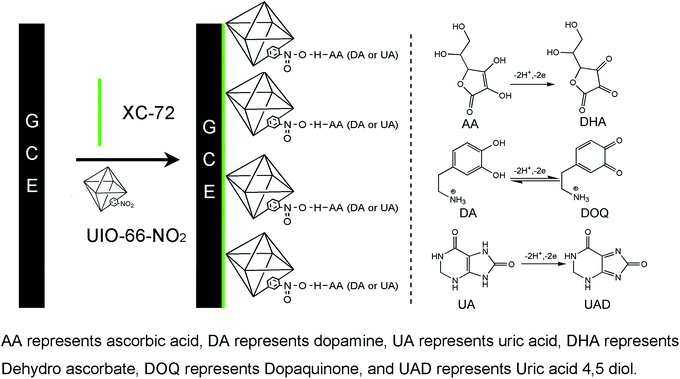 |
| Scheme 1 The modified electrode sensor preparation process and the redox reaction mechanism of AA, DA and UA at UIO-66-NO2@XC-72/GCE sensor. | |
2.4 Apparatus and instruments
FTIR spectrum was recorded on a Lambda 7600. Power X-ray diffraction (XRD) data were collected on a DX-2700B diffractometer. N2 adsorption–desorption isotherms were carried out by using a BeiShiDe Instrument-S&T. The morphologies were examined using a Quanta 200 scanning electron microscope and an H-7500 transmission electron microscope.
The cyclic voltammetry (CV), differential pulse voltammetry (DPV) measurements and electrochemical impedance spectroscopy (EIS) characterizations were performed on a Shiruisi electrochemical workstation. A conventional three electrode system was used in the measurements composed of a platinum wire counter electrode, a saturated calomel reference electrode (SCE), and a bare or modified glassy carbon working electrode.
3. Results and discussion
3.1 Physical characterization
Fig. S1† shows the FTIR spectrum of UIO-66-NO2. The absorption peaks at 3439 cm−1, 1658 cm−1, 1550 cm−1, and 1380 cm−1 should belong to –OH stretching vibration, –C
O vibration peak, –NO2 asymmetric stretching and symmetric stretching peaks, respectively.24 The XRD pattern of as-synthesized UIO-66-NO2 matches well with the simulated one, demonstrating the successful preparation of UIO-66-NO2.24,25 For XC-72 sample, the broad peak at 2θ = 24.6° is corresponding to the (0 0 2) peak of the hexagonal structure of graphitic structure.45 Besides, the UIO-66-NO2@XC-72 displays the characteristic diffraction peaks of both UIO-66-NO2 and XC-72, endowing the modified electrode sensor with synergistic advantages of the two materials (Fig. S2†).
Fig. S3a† reveals that all samples are typical characteristic of the microporous materials because they possess reversible, type-I isotherms with no obvious hysteresis.44,46 The pore size distributions (PSD) show the predominant pore size of the three samples from 0.49 nm for UIO-66-NO2, 0.43 nm for XC-72 to 0.45 nm for UIO-66-NO2@XC-72 (Fig. S3b†). Table 1 summarizes the Langmuir surface area, BET, micropore volume and average pore diameter data of UIO-66-NO2, XC-72 and UIO-66-NO2@XC-72. It can be seen that UIO-66-NO2@XC-72 exhibits the smaller surface area, micropore volumes and larger pore diameter compared with UIO-66-NO2 material. The results indicate that UIO-66-NO2 is successfully mixed with XC-72.
Table 1 Porous textural parameters of the UIO-66-NO2, XC-72 and UIO-66-NO2@XC-72
Samples |
SLangmuir/m2 g−1 |
SBET/m2 g−1 |
Micropore volume/cm3 g−1 |
Average pore diameter/nm |
UIO-66-NO2 |
978 |
865 |
0.14 |
3.01 |
XC-72 |
324 |
213 |
0.03 |
14.3 |
UIO-66-NO2@XC-72 |
644 |
528 |
0.06 |
13.0 |
Fig. 1(a and d) show that the UIO-66-NO2 possesses nanometre scale particles with the sizes of about 150 nm. The XC-72 displays spherical particles with the sizes of less than 100 nm in Fig. 1(b and e). Fig. 1(c and f) reveal that the UIO-66-NO2 is well-dispersed over XC-72 and its main morphology is not destroyed for UIO-66-NO2@XC-72.
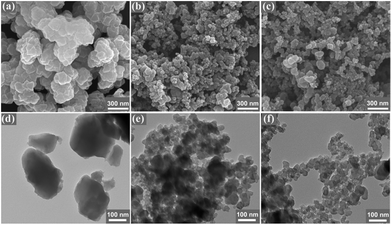 |
| Fig. 1 SEM and TEM images of UIO-66-NO2 (a and d), XC-72 (b and e) and UIO-66-NO2@XC-72 (c and f). Ratio of UIO-66-NO2 and XC-72 was 1 : 6 in UIO-66-NO2@XC-72. | |
3.2 Electrochemical characterization
The CV curves and EIS of the bare GCE and the different sensors are recorded in Fig. 2. The peak potential separation (ΔEp) reflects the electron transfer rate for the CV responses.47 As shown in Fig. 2a, the bare electrode has a pair of reversible redox peaks (ipa = 24.98 μA, ipc = −21.58 μA, ΔEp = 0.18 V), while the UIO-66-NO2/GCE sensor exhibits a broadened peak potential and small peak current, suggesting that the sensor surface is blocked due to the poor electron transfer of UIO-66-NO2 material.48 When XC-72 and UIO-66-NO2@XC-72 modified electrode surface, the ΔEp values decline to 0.16 and 0.15 V, respectively. The XC-72/GCE (ipa = 32.88 μA, ipc = −31.11 μA) and UIO-66-NO2@XC-72/GCE sensors (ipa = 30.65 μA, ipc = −29.81 μA) possess higher current, and smaller ΔEp profiles, indicating that XC-72 possesses good electronic conductivity and is beneficial to electron transfer.48–50 When 5.0 mM Ru(NH3)6Cl3 was added into 0.1 M KCl solution, the similar results can be obtained from Fig. 2b. It can be seen that the UIO-66-NO2/GCE sensor exhibits a broadened peak, while the XC-72/GCE (ipa = 37.67 μA, ipc = −27.95 μA, ΔEp = 0.15 V) and UIO-66-NO2@XC-72/GCE (ipa = 34.81 μA, ipc = −23.59 μA, ΔEp = 0.16 V) sensors receive higher current and similar ΔEp value compare with bare electrode (ipa = 20.41 μA, ipc = −12.59 μA, ΔEp = 0.16 V). The results confirm XC-72/GCE and UIO-66-NO2@XC-72/GCE sensors have better electrochemical properties than UIO-66-NO2/GCE.
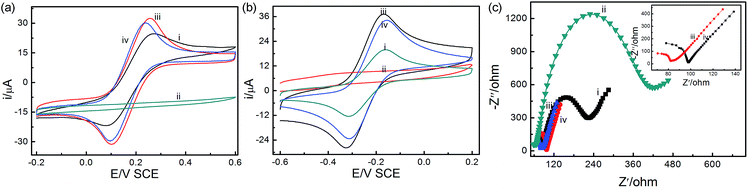 |
| Fig. 2 The cyclic voltammetry (CV) curves of bare GCE (i), UIO-66-NO2/GCE (ii), XC-72/GCE (iii) and UIO-66-NO2@XC-72/GCE (iv) sensors in (a) 5 mM K3[Fe(CN)6]/K4[Fe(CN)6] solution, (b) 5 mM Ru(NH3)6Cl3 solution containing 0.1 mM KCl as supporting electrolyte. (c) Electrochemical impedance spectroscopy (EIS) of the different electrode sensors in 5 mM K3[Fe(CN)6]/K4[Fe(CN)6] solution with 0.1 mM KCl. Scan rate: 100 mV s−1. | |
It is also investigated by EIS measurements that the electron transfer kinetics occur at the interface of electrode/electrolyte (Fig. 2c).51 The distinct semicircle curve at high frequencies reflects the ability of electron transfer (Rct) of different sensors47,48 and the smaller diameter of Rct represents higher electron transfer at the interface of electrode/electrolyte.49,52 The Rct values increase in the order of the XC-72/GCE sensor < UIO-66-NO2@XC-72/GCE sensor < bare GCE < UIO-66-NO2/GCE sensor. It reveals that the conductivity and electrochemical properties are well improved at XC-72 and UIO-66-NO2@XC-72 modified electrode sensor. The results are consistent with our data obtained by CV.
3.3 Electrocatalytic oxidation of AA, DA and UA
Fig. 3a depicts the three individual and mixed oxidation peaks at the potentials of 0.015 V, 0.23 V and 0.38 V at the UIO-66-NO2@XC-72/GCE sensor, corresponding to AA, DA and UA, respectively. Fig. 3b shows the CVs responses of the ternary mixture of AA, DA and UA at different electrodes. For bare GCE, the oxidation peaks of the three species nearly overlapped and a broad peak was found at 0.5 V, proving that it possessed poor sensitivity and selectivity towards the oxidation of the three compounds. For comparison, the UIO-66-NO2/GCE sensor had no obvious redox peaks and possessed a large background current due to the weak conductivity of the MOFs. For XC-72/GCE sensor, the oxidation peaks of DA and UA at 0.2 V and 0.34 V suggested that the electroactive area of the electrode sensor increased significantly. Interestingly, there were obvious peaks of the three species at the UIO-66-NO2@XC-72/GCE sensor, indicating high electrochemical activities in response to AA, DA and UA. The results demonstrated that the UIO-66-NO2@XC-72/GCE sensor effectively facilitated the individual and simultaneous determination of the small biomolecules.
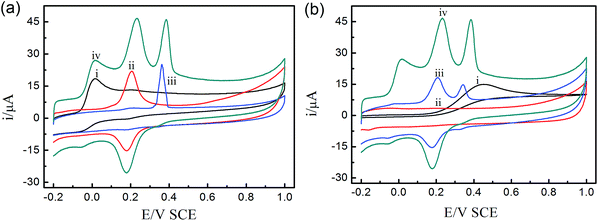 |
| Fig. 3 (a) CVs of (a) 3 μM AA (i), 1.5 μM DA (ii), 1.5 μM UA (iii) and the mixture of AA (3 μM), DA (1.5 μM) and UA (1.5 μM) (iv), respectively at UIO-66-NO2@XC-72/GCE sensor, and (b) bare GCE (i), UIO-66-NO2/GCE sensor (ii), XC-72/GCE sensor (iii), and UIO-66-NO2@XC-72/GCE sensor (iv). Supporting electrolyte: 0.1 M phosphate buffer solutions (PBS). Scan rate: 50 mV s−1 (pH 6.0). | |
The experimental phenomena can be ascribed as follows: (i) XC-72 with high electrical conductivity can promote the electron transfer between modified electrode sensor surface and biomolecules, and enhance voltammetry peak separation. (ii) The UIO-66-NO2@XC-72/GCE sensor possesses excellent electrochemical properties due to the synergetic effect of UIO-66-NO2 and XC-72. (iii) The hydrogen bond between O (from –NO2 group of UIO-66-NO2) and H (from AA, DA and UA) can be helpful to improve the easy adsorption of AA, DA and UA, and finally promotes the response of the electrode sensor towards determination of the three biomolecules, especially contributes to the appearance of oxidation peak of AA (Scheme 1).
3.4 Optimization of the experimental conditions
To obtain the high sensitivity and selectivity, a series of optimized conditions were carried out in this paper. In this regard, the ratio of UIO-66-NO2 and XC-72, the accumulation time, pH value and the scan rate were investigated. The effect factors were performed using CV method except for the accumulation time, which was employed DPV method.
The ratio of UIO-66-NO2 and XC-72 plays important roles in the fabrication of the UIO-66-NO2@XC-72/GCE sensor. It can be seen from Fig. 4a that the peak currents of AA and UA increased with the increasing ratio of the two materials until it obtained 1
:
7. Continuing increasing the ratio, the peak currents of the two materials until it obtained 1
:
7. Continuing increasing the ratio, the peak currents of the two biomolecules decreased sharply. For DA, the maximum of the peak current was achieved at the ratio of 1
:
8. The reason can be ascribed to the synergistic effect of the UIO-66-NO2 and XC-72. The low ratio indicated the amount of XC-72 decreased, causing the conductivity of the fabricated sensor was poor and the electron transfer between the sensor and the analyte was impeded. By contrast, the high ratio meant the number of UIO-66-NO2 decreased, the hydrogen bond between MOFs and the analyte was weaker and the surface area of the sensor became smaller. Based on the above experiments, the ratio of 1
:
7 was chosen for the following experiments.
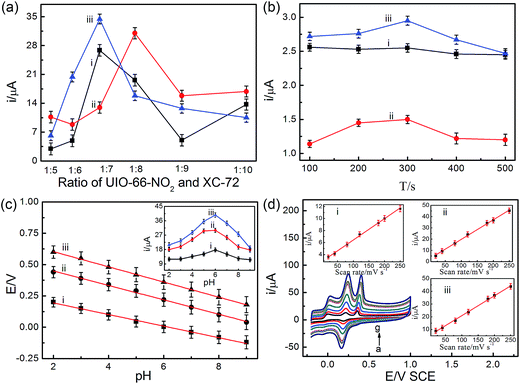 |
| Fig. 4 Effects of the ratio of UIO-66-NO2 and XC-72 (a), the accumulation time (b), pH value (c) and the scan rate (d) on the peak current for the oxidation of 3 μM AA (i), 1.5 μM DA (ii), and 1.5 μM UA (iii) in 0.1 M PBS at UIO-66-NO2@XC-72/GCE sensor. Other conditions are the same as Fig. 3. | |
From Fig. 4b, it was found that the accumulation time can affect the oxidation peak currents of the three biomolecules at the UIO-66-NO2@XC-72/GCE sensor. The currents of DA and UA first increased and then decreased gradually with the increasing accumulation time, and the maximum value was 300 s. The current of AA nearly kept a constant with the change of the accumulation time. Therefore, 300 s was chosen in the following research for simultaneous determination of the three compounds.
It is well known that the pH value has a profound effect on the electrochemical response towards the determination of the analytes. From Fig. 4c, with the increase of pH from 2.0 to 9.0, the oxidation potentials of the three biomolecules shifted negatively with linear slopes of 46.3, 57.5 and 60.6 mV per pH, respectively. The slopes were close to the theory value of 59 mV per pH, demonstrating that equal number of protons and electrons have taken part in the electrochemical redox reaction process.53,54 It also can be seen that the peak currents of AA, DA and UA increased with the increasing pH value (from 2.0 to 6.0), and then it decreased from pH 6.0 to 9.0. In addition, the pKa values of AA, DA and UA were 4.10, 8.87 and 5.75, respectively. DA existed as cationic species at pH 6.0. The carboxylic functional groups of MOFs at the electrode surface could effectively capture the cationic species.3 However, AA and UA existed as un-ionized species at pH 6.0. In this case, the hydrogen bond effect between MOFs and the three biomolecules plays more important roles (Scheme 1). Therefore, 0.1 M PBS of pH 6.0 was selected as an optimized value for the further experiments.
To evaluate the reaction kinetics, the CV responses at different scan rates for the three compounds was investigated. Fig. 4d shows that the oxidation peak currents of AA, DA and UA gradually increased as the scan rate increases, with a linear relationship between the peak currents and the scan rate in the range of 20–250 mV s−1 (the inset of Fig. 4d). The regression equations were expressed as follows IAA (μA) = 2.86 + 0.036CAA (μM) (R2 = 0.998), IDA (μA) = 2.32 + 0.17CDA (μM) (R2 = 0.998) and IUA (μA) = 5.26 + 0.16CUA (μM) (R2 = 0.998). It demonstrates that electrochemical reaction of the three biomolecules at UIO-66-NO2@XC-72/GCE sensor was a surface-controlled process.
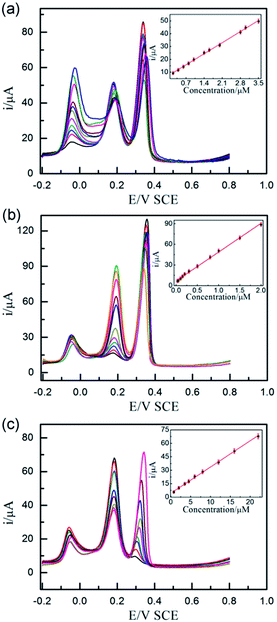 |
| Fig. 5 DPVs of different concentrations of AA (a) (from inner to outer): 0.2, 0.4, 0.6, 0.8, 1.0, 1.4, 1.6, 2.0, 2.8, 3.0, 3.5 μM containing 1.5 μM DA, 1.5 μM UA, different concentrations of DA (b) (from inner to outer): 0.03, 0.05, 0.1, 0.15, 0.2, 0.3, 0.5, 0.8, 1.0, 1.5, 2.0 μM containing 3 μM AA, 1.5 μM UA, and different concentrations of UA (c) (from inner to outer): 0.75, 2.0, 3.5, 4.5, 6.0, 8.0, 12, 16, 22 μM containing 3 μM AA, 1.5 μM DA at UIO-66-NO2@XC-72/GCE sensor. Other conditions are the same as Fig. 3. The inset figures show the plots of the peak currents vs. the concentration of AA, DA and UA, respectively. | |
3.5 Simultaneous determination of AA, DA and UA
Fig. 5 displays DPV responses of the mixture of AA, DA and UA when the concentration of the detected analyte changed with the other two species keeping constant at the optimized electrode sensor. The experiments indicated the UIO-66-NO2@XC-72/GCE sensor exhibited wide linear relationships between the oxidation peak current responses and the analytes concentrations, with the correlation coefficient of 0.998, 0.998 and 0.999, respectively. The following linear regression equations were expressed as IAA (μA) = 7.08 + 12.35CAA (μM), IDA (μA) = 6.90 + 41.81CDA (μM) and IUA (μA) = 4.34 + 2.93CUA (μM). The detection ranges were 0.2–3.5 μM for AA, 0.03–2.0 μM for DA and 0.75–22 μM for UA with the detection limits were 0.12, 0.005 and 0.03 μM (S/N = 3), respectively.
The performance of the UIO-66-NO2@XC-72/GCE senor was compared with some existing sensors, as shown in Table 2. The minimum concentrations of AA, DA and UA and the detection limits of this paper are lower than that of those previously reported materials.3,10,13,18,19,47,49,55–57 These results confirmed that the UIO-66-NO2@XC-72 material was a promising material for the electroanalytical detection of the three biomolecules.
Table 2 Comparison of the optimized sensors for the detection of AA, DA and UA with other modified electrode sensors
Methods |
Linear range (μM) |
Detection limit (μM) |
References |
AA |
DA |
UA |
AA |
DA |
UA |
*Polymer represents poly(eriochrome black T). |
DpAu/PTCA-Cys/GCE |
20–700 |
2–402 |
0.4–252 |
6.40 |
0.67 |
0.12 |
3 |
Carbon nanotube/GCE |
80–1360 |
0.5–10 |
— |
20 |
0.10 |
— |
10 |
Au/RGO/GCE |
240–1500 |
6.8–41 |
8.8–53 |
51 |
1.40 |
1.80 |
13 |
*Polymer/GCEa |
150–1000 |
0.1–200 |
10–130 |
10 |
0.02 |
1.00 |
18 |
P-ATD/GCE |
30–300 |
5–50 |
10–100 |
2.01 |
0.33 |
0.19 |
19 |
NCNF/GCE |
50–3000 |
1–200 |
5.0–200 |
50 |
0.50 |
1.00 |
49 |
NiCo-NPs-in-N/C |
500–1500 |
0.5–900 |
10–500 |
0.09 |
0.08 |
0.01 |
47 |
rGO–CNT/ITO |
10–200 |
0.2–8.0 |
0.2–16 |
5.31 |
0.04 |
0.17 |
55 |
SZP/MB |
100–1600 |
6–100 |
22–350 |
8.30 |
1.70 |
3.70 |
57 |
GEF/CFE |
74–2306 |
1.4–125.7 |
4.0–371 |
73.52 |
1.36 |
3.98 |
56 |
This work |
0.2–3.5 |
0.03–2.0 |
0.8–22 |
0.12 |
0.005 |
0.03 |
|
3.6 Reproducibility and stability
The reproducibility of the UIO-66-NO2@XC-72/GCE sensor was evaluated. The relative standard deviations (RSD) for five different electrode sensors were 5.04% for AA, 4.08% for DA and 4.92% for UA. The RSD of the same sensor in eight successive experiments were 3.02% for AA, 2.54% for DA and 2.92% for UA, suggesting the proposed sensor exhibited excellent reproducibility. Furthermore, the sensor was stored in the refrigerator at 4 °C. After 10 days, there was only an 8% loss in the response current of the developed sensor. After 30 days, the proposed sensor can retain 85% of its initial response, which was stable enough for the application in electrochemical sensor.
3.7 Real sample analysis
The proposed method was evaluated for the determination of AA, DA and UA in the real samples. DA hydrochloride injection solution and human urine were measured for analysis. Generally, DA and UA samples were diluted 10 times and 100 times with PBS, respectively. The spiked sample with certain amounts of DA and UA were added to the corresponding samples. Subsequently, the experiments were carried out five times and the Table 3 summarized the analytical results. It can be seen that the RSD is in the range of 1.38–2.32% and the recovery is between 98.3–101.3%. It indicated that the proposed sensor possessed sufficient precision and high accuracy.
Table 3 Recoveries of the determination of DA and UA in the real samples
Samples |
Detected (μM) |
Added (μM) |
Found (μM) |
Recovery (%) |
RSD (%) (n = 3) |
Injection |
|
10 |
38.40 |
101.3 |
1.38 |
Solution |
27.90 |
15 |
43.10 |
100.5 |
1.96 |
(DA) |
|
20 |
47.10 |
98.3 |
1.52 |
|
|
10 |
24.12 |
101.0 |
1.75 |
Urine |
13.87 |
20 |
34.09 |
100.7 |
2.32 |
(UA) |
|
35 |
48.47 |
99.2 |
1.94 |
4. Conclusions
In conclusion, a new approach for fabrication of the UIO-66-NO2@XC-72/GCE sensor was presented for the first time to simultaneously determine AA, DA and UA. The procedure of fabricating sensor was simple, convenient and rapid. The developed sensor exhibited high sensitivity and selectivity with wide linear concentration range and low detection limit. Interestingly, the satisfactory results indicate that MOFs could be a promising candidate for application in electroanalysis, and promisingly, it was used by mixing with other conductive materials to fabricate excellent sensors to detect other electrochemical biomolecules.
Acknowledgements
This work is financially supported by the “Iconic Innovation Project” plan of Henan Institute of Science and Technology (No. 2015BZ02) and the Henan Innovation Foundation for Higher Education (No. 2012HASTIT037).
References
- A. A. Abdelwahab and Y.-B. Shim, Sens. Actuators, B, 2015, 221, 659–665 CrossRef CAS.
- M. Noroozifar, M. Khorasani-Motlagh, R. Akbari and M. Bemanadi Parizi, Biosens. Bioelectron., 2011, 28, 56–63 CrossRef CAS PubMed.
- W. Zhang, Y. Chai, R. Yuan, J. Han and S. Chen, Sens. Actuators, B, 2013, 183, 157–162 CrossRef CAS.
- Y. J. Yang and W. Li, Biosens. Bioelectron., 2014, 56, 300–306 CrossRef CAS PubMed.
- L. Zhao, H. Li, S. Gao, M. Li, S. Xu, C. Li, W. Guo, C. Qu and B. Yang, Electrochim. Acta, 2015, 168, 191–198 CrossRef CAS.
- M. Behpour, S. M. Ghoreishi, E. Honarmand and M. Salavati-Niasari, Analyst, 2011, 136, 1979–1986 RSC.
- D. Datta, R. K. Bera, S. Jana, B. Manna, D. Roy, A. Anoop, C. R. Raj and T. Pathak, Chem.–Asian J., 2015, 10, 1554–1560 CrossRef CAS PubMed.
- Z. Yang, X. Zheng, Z. Li and J. Zheng, Analyst, 2016, 141, 4757–4765 RSC.
- N. Jia, Z. Wang, G. Yang, H. Shen and L. Zhu, Electrochem. Commun., 2007, 9, 233–238 CrossRef CAS.
- Z. Wang, J. Liu, Q. Liang, Y. Wang and G. Luo, Analyst, 2002, 127, 653–658 RSC.
- Z. Wang, M. Shoji and H. Ogata, Analyst, 2011, 136, 4903–4905 RSC.
- W. A. El-Said, J.-H. Lee, B.-K. Oh and J.-W. Choi, Electrochem. Commun., 2010, 12, 1756–1759 CrossRef CAS.
- C. Wang, J. Du, H. Wang, C. E. Zou, F. Jiang, P. Yang and Y. Du, Sens. Actuators, B, 2014, 204, 302–309 CrossRef CAS.
- Y. Temerk and H. Ibrahim, Sens. Actuators, B, 2016, 224, 868–877 CrossRef CAS.
- L. X. Chen, J. N. Zheng, A. J. Wang, L. J. Wu, J. R. Chen and J. J. Feng, Analyst, 2015, 140, 3183–3192 RSC.
- S. Mahshid, C. Li, S. S. Mahshid, M. Askari, A. Dolati, L. Yang, S. Luo and Q. Cai, Analyst, 2011, 136, 2322–2329 RSC.
- J. Bai, B. Lu, X. Bo and L. Guo, Electrochem. Commun., 2010, 12, 1563–1567 CrossRef CAS.
- H. Yao, Y. Sun, X. Lin, Y. Tang and L. Huang, Electrochim. Acta, 2007, 52, 6165–6171 CrossRef CAS.
- P. Kalimuthu and S. A. John, Talanta, 2010, 80, 1686–1691 CrossRef CAS PubMed.
- M. Pandurangachar, B. E. Kumara Swamy, B. N. Chandrashekar, O. Gilbert and B. S. Sherigara, J. Mol. Liq., 2011, 158, 13–17 CrossRef CAS.
- X. Jiang and X. Lin, Analyst, 2005, 130, 391–396 RSC.
- K. Deng, J. Zhou and X. Li, Electrochim. Acta, 2013, 114, 341–346 CrossRef CAS.
- S. Yu, C. Luo, L. Wang, H. Peng and Z. Zhu, Analyst, 2013, 138, 1149–1155 RSC.
- M. Kandiah, M. H. Nilsen, S. Usseglio, S. Jakobsen, U. Olsbye, M. Tilset, C. Larabi, E. A. Quadrelli, F. Bonino and K. P. Lillerud, Chem. Mater., 2010, 22, 6632–6640 CrossRef CAS.
- S. S. Nagarkar, A. V. Desai and S. K. Ghosh, Chemistry, 2015, 21, 9994–9997 CrossRef CAS PubMed.
- Y. Bai, Y. Dou, L. H. Xie, W. Rutledge, J. R. Li and H. C. Zhou, Chem. Soc. Rev., 2016, 45, 2327–2367 RSC.
- S. Han, S. C. Warren, S. M. Yoon, C. D. Malliakas, X. Hou, Y. Wei, M. G. Kanatzidis and B. A. Grzybowski, J. Am. Chem. Soc., 2015, 137, 8169–8175 CrossRef CAS PubMed.
- C. Hou, Y. L. Bai, X. Bao, L. Xu, R. G. Lin, S. Zhu, J. Fang and J. Xu, Dalton Trans., 2015, 44, 7770–7773 RSC.
- X. L. Hu, F. H. Liu, C. Qin, K. Z. Shao and Z. M. Su, Dalton Trans., 2015, 44, 7822–7827 RSC.
- S. J. Garibay and S. M. Cohen, Chem. Commun., 2010, 46, 7700–7702 RSC.
- S. Dang, T. Wang, F. Yi, Q. Liu, W. Yang and Z. M. Sun, Chem.–Asian J., 2015, 10, 1703–1709 CrossRef CAS PubMed.
- Y. Fang, S. Guo, C. Zhu, S. Dong and E. Wang, Chem.–Asian J., 2010, 5, 1838–1845 CrossRef CAS PubMed.
- Y. Kobayashi, B. Jacobs, M. D. Allendorf and J. R. Long, Chem. Mater., 2010, 22, 4120–4122 CrossRef CAS.
- T. C. Narayan, T. Miyakai, S. Seki and M. Dinca, J. Am. Chem. Soc., 2012, 134, 12932–12935 CrossRef CAS PubMed.
- Y. Li, C. Huangfu, H. Du, W. Liu, Y. Li and J. Ye, J. Electroanal. Chem., 2013, 709, 65–69 CrossRef CAS.
- A. A. Talin, A. Centrone, A. C. Ford, M. E. Foster, V. Stavila, P. Haney, R. A. Kinney, V. Szalai, F. El Gabaly, H. P. Yoon, F. Leonard and M. D. Allendorf, Science, 2014, 343, 66–69 CrossRef CAS PubMed.
- B. Le Ouay, M. Boudot, T. Kitao, T. Yanagida, S. Kitagawa and T. Uemura, J. Am. Chem. Soc., 2016, 138, 10088–10091 CrossRef CAS PubMed.
- F. Ragon, B. Campo, Q. Yang, C. Martineau, A. D. Wiersum, A. Lago, V. Guillerm, C. Hemsley, J. F. Eubank, M. Vishnuvarthan, F. Taulelle, P. Horcajada, A. Vimont, P. L. Llewellyn, M. Daturi, S. Devautour-Vinot, G. Maurin, C. Serre, T. Devic and G. Clet, J. Mater. Chem. A, 2015, 3, 3294–3309 CAS.
- X. Zhao, X. Liu, Z. Zhang, X. Liu and W. Zhang, RSC Adv., 2016, 6, 92011–92019 RSC.
- S. Ling and B. Slater, Chem. Sci., 2016, 7, 4706–4712 RSC.
- E. A. Kozlova, V. N. Panchenko, Z. Hasan, N. A. Khan, M. N. Timofeeva and S. H. Jhung, Catal. Today, 2016, 266, 136–143 CrossRef CAS.
- V. N. Panchenko, M. N. Timofeeva and S. H. Jhung, Catal. Rev., 2016, 58, 209–307 CAS.
- J. Hu, H.-B. Zhang, S. Hong, Z.-G. Jiang, C. Gui, X. Li and Z.-Z. Yu, Ind. Eng. Chem. Res., 2014, 53, 2270–2276 CrossRef CAS.
- W. W. Zhao, C. Y. Zhang, Z. G. Yan, L. P. Bai, X. Wang, H. Huang, Y. Y. Zhou, Y. Xie, F. S. Li and J. R. Li, J. Chromatogr. A, 2014, 1370, 121–128 CrossRef CAS PubMed.
- X. Ren, D. Shao, S. Yang, J. Hu, G. Sheng, X. Tan and X. Wang, Chem. Eng. J., 2011, 170, 170–177 CrossRef CAS.
- Y. Huang, W. Qin, Z. Li and Y. Li, Dalton Trans., 2012, 41, 9283–9285 RSC.
- X. Zhang, W. Yan, J. Zhang, Y. Li, W. Tang and Q. Xu, RSC Adv., 2015, 5, 65532–65539 RSC.
- Z. Xue, Y. Feng, H. Guo, C. Hu, A. Mahmoud idris Mohmed, J. Li and X. Lu, RSC Adv., 2014, 4, 5849 RSC.
- J. Sun, L. Li, X. Zhang, D. Liu, S. Lv, D. Zhu, T. Wu and T. You, RSC Adv., 2015, 5, 11925–11932 RSC.
- H. Imran, P. N. Manikandan and V. Dharuman, RSC Adv., 2015, 5, 63513–63520 RSC.
- H. L. Poh and M. Pumera, Chem.–Asian J., 2012, 7, 412–416 CrossRef CAS PubMed.
- L. Zhang, L. Ning, S. Li, H. Pang, Z. Zhang, H. Ma and H. Yan, RSC Adv., 2016, 6, 66468–66476 RSC.
- F. Ye, C. Feng, J. Jiang and S. Han, Electrochim. Acta, 2015, 182, 935–945 CrossRef CAS.
- X. Zhang, L.-X. Ma and Y.-C. Zhang, Electrochim. Acta, 2015, 177, 118–127 CrossRef CAS.
- Y. Zhang, Y. Ji, Z. Wang, S. Liu and T. Zhang, RSC Adv., 2015, 5, 106307–106314 RSC.
- J. Du, R. Yue, F. Ren, Z. Yao, F. Jiang, P. Yang and Y. Du, Biosens. Bioelectron., 2014, 53, 220–224 CrossRef CAS PubMed.
- J. Argüello, V. L. Leidens, H. A. Magosso, R. R. Ramos and Y. Gushikem, Electrochim. Acta, 2008, 54, 560–565 CrossRef.
Footnote |
† Electronic supplementary information (ESI) available. See DOI: 10.1039/c6ra26933h |
|
This journal is © The Royal Society of Chemistry 2017 |