DOI:
10.1039/C6RA26419K
(Paper)
RSC Adv., 2017,
7, 11085-11093
Preparation of γ-PGA hydrogels and swelling behaviors in salt solutions with different ionic valence numbers
Received
7th November 2016
, Accepted 7th February 2017
First published on 13th February 2017
Abstract
In this paper, a novel poly γ-glutamic acid (γ-PGA) hydrogel was successfully synthesized by solution polymerization and ethylene glycol diglycidyl ether (EGDE) was used as crosslinker. The structure and morphology of the γ-PGA was investigated using scanning electron microscopy (SEM) and Fourier transform infrared spectroscopy (FTIR) techniques. The influence of ionic (Na+, Ca2+ and Fe3+) concentrations, temperature and pH on the swelling degree of γ-PGA hydrogels was studied. The results revealed that the swelling capacity is enhanced with increasing pH, but decreased with increasing ionic concentration. The swelling kinetics study indicated that γ-PGA hydrogels were ion-sensitive and presented more limited swelling kinetics in metal ion solutions with higher ionic valence numbers. The results showed that the pseudo-second-order adsorption kinetics were predominant. Maximum swelling degrees were obtained for γ-PGA hydrogels with 21.03 g g−1 in 0.154 mol L−1 NaCl solution, 8.5 g g−1 in 0.154 mol L−1 CaCl2 solution and 12.8 g g−1 in 0.154 mol L−1 FeCl3 solution. Considering the simple preparation process, the ionic- and pH-sensitive properties, this γ-PGA hydrogel might have high potential to be used in medical and other fields.
1. Introduction
Over the past few decades, hydrogels have been widely used as functional materials in many applications and fields, for example, tissue implants,1–3 drug delivery4–6 and superabsorbents.7–9 The composition of hydrogels can be natural polymers or synthetic polymers.10 New hydrogels with biocompatibility and stimuli-response capacity, developed from naturally occurring polymers, have been broadly investigated and applied in medical products in recent years.11–13 These hydrogels are promising matrix materials for biomedical and tissue engineering due to their biocompatibility, tunable biomimetic properties and capability of absorbing liquid.14 As a result, hydrogels prepared from natural polymers were more developed in medical and tissue engineering such as wound dressings15 and absorbents,16 than in other fields.17,18
As newly developed absorbent materials, hydrogels can be practically cast into any size, shape or form.19 Moreover, hydrogels can absorb liquid to reach a thousand-fold of their dry weight due to the hydrophilic groups in their polymeric networks.20
Poly(γ-glutamic acid), γ-PGA, is an unusual anionic poly amino acid linked by the peptide bond between the α-amino group and the γ-carboxyl group21 (Scheme 1). Considerable attention has been focused on γ-PGA due to its water-solubility, biodegradability, edibility and non-toxicity towards human.22 As a result, γ-PGA and its derivatives have been broadly used in many industrial fields such as food industry,23,24 medicine,25,26 cosmetic27,28 and agriculture.29 In addition, γ-PGA offers a wide range of applications in water and wastewater treatment, including highly water absorbable hydrogels,30,31 catalytic fibers,32 metal chelates33–35 and bioflocculants.36–38
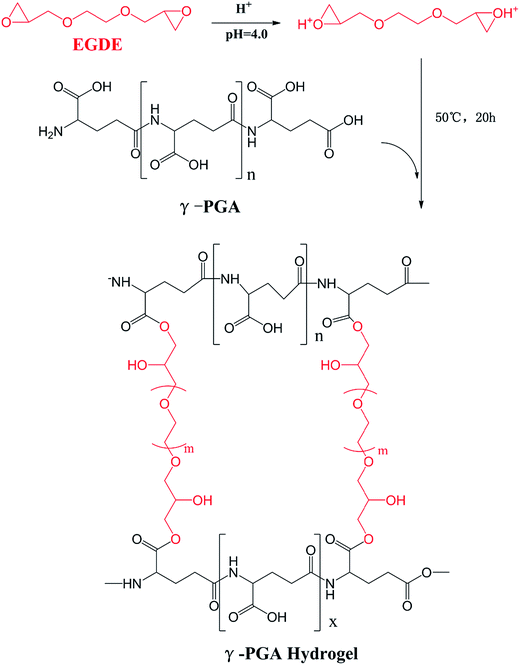 |
| Scheme 1 Synthetic of synthesis. | |
During the past decades, our society has suffered a lot from the rapid growth of industrial ion wastewater. In order to study the applicability of hydrogels in wastewater treatment, we further investigated the ion absorption properties of hydrogels prepared from γ-PGA, poly(N-hydroxyethylacrylamide),16 poly acrylic acid,39 poly aspartic acid and poly vinyl alcohol. It is commonly acknowledged that the swelling kinetic behavior of hydrogels in ion solutions is the major factor to determine their practicability. Therefore, it is important to further study this factor in more detail. Since the adsorption study of ion has been widely investigated, the main goal of this study has been focused on the effects of ionic valence number on the swelling kinetics of γ-PGA hydrogels. However, since hydrogels have been broadly applied in biomedical, it is of great necessary to study how hydrogels, especially those that have been reported with ion-sensitive properties, swell in commonly used biological liquids that are rich in different ions, such as sweat (Na+, K+), blood (Ca2+, Fe2+, Fe3+) and urine (Na+, K+). In previous studies, γ-PGA hydrogels were prepared by UV,40 γ-irradiation,31 chemical reagents.41–43 However, γ-PGA hydrogels prepared by γ-PGA and ethylene glycol diglycidyl ether (EGDE) are a representative model to research the swelling behavior of poly amino acid hydrogels. The preparation of these hydrogels only needs a simple aqueous mixture process which is convenient to control.44 Besides, NaCl, CaCl2, and FeCl3 were chosen to study how γ-PGA hydrogels swelled in ionic solutions with different valence numbers.
To achieve this, we prepared γ-PGA hydrogels by EGDE via a simple mixture process in the aqueous solution in this paper. The chemical characteristics was analyzed by FTIR and SEM. Moreover, we studied the effects of ionic concentration, temperature and pH on the swelling kinetics. The pseudo-first-order and pseudo-second-order equations were utilized to verify the mechanism and kinetics of the swelling process.
2. Materials and methods
2.1. Materials
γ-Polyglutamic acid (γ-PGA, average molecular weight, 730 kDa) was purchased from Shineking Biotechnology (Nanjing, China). Ethylene glycol diglycidyl ether (EGDE) was purchased from Tongshi Chemical Ltd. (Yantai, China). NaCl, CaCl2, and FeCl3·6H2O were purchased from Sinopharm group Co. Ltd (Shanghai, China).
2.2. Preparation of γ-PGA hydrogel
γ-PGA hydrogels were synthesized by a simple mixture process in the aqueous solution (Scheme 1). γ-PGA was dissolved in deionized water to a 12% wt aqueous solution, and then ethylene glycol diglycidyl ether (EGDE) was added as the crosslinking agent. The weight of EGDE was equal to 40% of the weight of γ-PGA. The pH of the solutions was adjusted to 4.0, and the mixture was maintained at 50 °C for 20 h. The formed hydrogel was cut into cubes with an approximately 2 mm side length and immersed in an excess of deionized water for 1 week with daily water exchanges to remove the unreacted monomer.
2.3. Chemical characterization
The structure of γ-PGA hydrogel samples was analysed by Fourier transform infrared (FTIR) spectra (Thermo Fisher Technology, America). The scan mode was the transmittance mode with a 4 cm−1 scan resolution. γ-PGA hydrogel samples were freeze dried and smashed to powder.
SEM (TM-3030, Hitachi, Japan) was used to observe the surface and cross-section morphologies of the hydrogels. First, freeze dried γ-PGA hydrogel samples were immobilized by Hardy fiber microtome. Then the surface samples and cross-section samples were cut into 1 mm slices. Finally, all samples were coated with gold for 10 s prior to SEM observation (accelerating voltage = 5000 V).
All freeze dried γ-PGA hydrogel samples were swelled to equilibrium in different swelling media and then were pre-frozen at −10 °C for 3 h in an ultra-low temperature freezer (DW-HL388, Meiling Cryogenic Technology, China). Finally, samples were freeze dried in a freeze-dryer (FD-1CL, DTY Technology, China).
2.4. The swelling capacity in different conditions
2.4.1. The effect of ion concentration. As shown in Table 1, the swelling capacities of γ-PGA hydrogels were determined in NaCl, CaCl2 and FeCl3 solutions with different concentrations according to the above method described in a previous study, respectively.16
Table 1 Concentrations of NaCl, CaCl2 and FeCl3 solutions (N1, N2 and N3 indicate the different concentrations of Na+. C1, C2 and C3 indicate the different concentrations of Ca2+. F1, F2 and F3 indicate the different concentrations of Fe3+)
|
N1 |
N2 |
N3 |
C1 |
C2 |
C3 |
F1 |
F2 |
F3 |
Metal ion |
NaCl |
CaCl2 |
FeCl3 |
Molar concentrations (mol L−1) |
0.154 |
0.769 |
1.539 |
0.154 |
0.769 |
1.539 |
0.015 |
0.077 |
0.154 |
Mass concentrations (g L−1) |
9.000 |
45.00 |
90.00 |
17.08 |
85.39 |
170.8 |
4.160 |
20.79 |
41.58 |
2.4.2. The effect of temperature. The swelling capacities of γ-PGA hydrogels were determined in 0.154 mol L−1 NaCl, CaCl2 and FeCl3 solutions according to the above method described in a previous study, respectively.16 The temperatures of the swelling environment were set to be 15, 30, 45 and 60 °C in a cultivation tank, respectively.
2.4.3. The effect of pH. The swelling capacities of γ-PGA hydrogels were determined in 0.154 mol L−1 NaCl and CaCl2 solutions according to the above method described in a previous study, respectively.16 The pHs of the swelling environment were set to be 1, 2, 3, 4, 5, 6 and 7, respectively. The desired acidic pHs were adjusted by HCl solution. The pHs were precisely determined by a pH meter.
2.5. Swelling kinetics study
γ-PGA hydrogel cubes (5 × 5 × 5 mm) were previously dried at 50 °C. The weights of the wet hydrogels (Wo) were measured at predetermined time while equilibrium in different swelling media at room temperature. The hydrogels were then thoroughly dried and the weights of the dry hydrogels (Wd) were measured.45,46 The swelling degree (Q) was calculated from the following expression (1):
The rate of swelling in DI water for PGA hydrogels can be readily obtained from the equation for the pseudo-first-order and pseudo-second-order models derived on the basis of swelling capacity on solid phase expressed (2) and (3) as follows, respectively:16
|
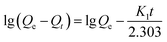 | (2) |
|
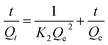 | (3) |
where
Qt (g g
−1) and
Qe (g g
−1) are the amount of swelling in equilibrium and at time
t, respectively;
K1 (min
−1) and
K2 (min
−1) are the rate constant.
3. Results and discussion
3.1. Preparation and characterization of γ-PGA hydrogels
γ-PGA hydrogels were prepared by a simple mixture process in the aqueous solution (Scheme 1). At the beginning, under acidic condition, H+ combined with the epoxy group from EGDE and formed the ionized hydrogen group. Then, nucleophilic addition happened between –COOH from γ-PGA and the ionized epoxy group. Finally, H+ was separated and crosslinking bond was formed. The whole reaction mechanism belonged to SN2 type.47
As shown in Fig. 1, FTIR spectroscopy was carried out to further confirm the crosslinking reaction. The broad peak at around 3436 cm−1 is attributed to the overlap of N–H and O–H stretching vibrations. The peak located at 1080.1 cm−1 arises from the stretching vibrations of C–O–C bond due to the addition of EGDE.48 It shows that there is ether bond in the γ-PGA hydrogel.49 In addition, it is worth noting that the peak at 1639.4 cm−1 strengthened while the peak at 1702.4 cm−1 weakened in γ-PGA hydrogels. These peaks belong to the C
O stretching of ester and the C
O stretching of the carbonyl (the peak I of carbonyl),50 respectively. This indicates that the crosslinking reaction occurred between the carbonyl of γ-PGA and the epoxide groups of EGDE and then ester bond formed. All of these changes illustrate that there is chemical reaction between EGDE and γ-PGA, and the reaction leads to the formation of new ester bond as crosslinking linkage.
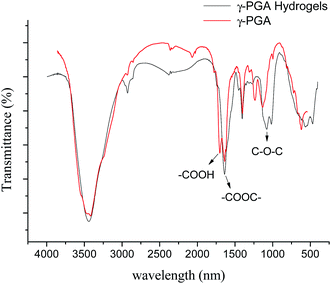 |
| Fig. 1 FTIR spectra of γ-PGA and γ-PGA hydrogel. | |
To observe the macroscopic form and the microstructure of γ-PGA hydrogels, the morphology of swelling equilibrium hydrogels was listed in Table 2. As was shown in Table 2 (a), (b) and (c), after swelling equilibrium, the form of γ-PGA hydrogels displayed a similar color as swelled ionic solutions and kept a regular cubic shape. This indicated that swelled γ-PGA hydrogels also adsorbed metal ions into its structure, and this means that γ-PGA hydrogels have appropriate mechanical strength to be applied.
Table 2 The morphology of γ-PGA hydrogels after swelling equilibrium in 0.154 mol L−1 NaCl, CaCl2, FeCl3 solutions
|
DI water |
NaCl |
CaCl2 |
FeCl3 |
Form |
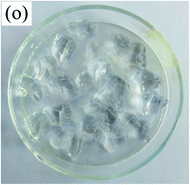 |
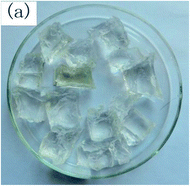 |
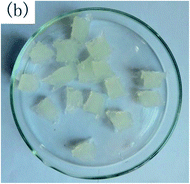 |
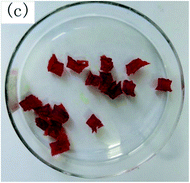 |
Cross-section |
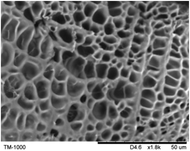 |
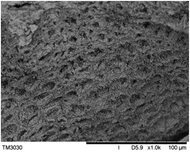 |
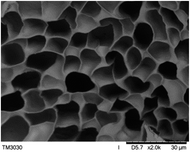 |
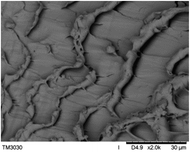 |
Surface |
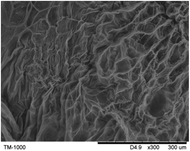 |
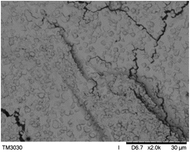 |
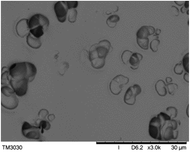 |
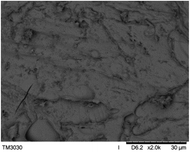 |
The surface and cross-section morphology of hydrogels was visualized by SEM. Table 2 presented a three dimensional network, and the porous structure of hydrogels was densely covered with irregularly shaped holes. As the ionic valence number increased, the networks became smaller. When swelled in FeCl3 solution, the porous structure in hydrogels collapsed and showed narrowly shaped micropores. These trends suggested that increasing ionic valence number may restrict the swelling behavior of hydrogels.51 As shown in Table 2, γ-PGA hydrogels swelled in different ionic solutions did not have any significant change in the surface. The result showed that γ-PGA hydrogels swelled in DI water and ionic solutions both have a rough wrinkled surface without obvious holes.10
3.2. The effects of ionic concentration, temperature and pH
3.2.1. The effect of temperature. Fig. 2 depicts the effect of temperature on the swelling capacity of γ-PGA hydrogels. In general, the swelling degree of γ-PGA hydrogels in NaCl solution was slightly raised with the temperature increasing from 15 to 60 °C. But in CaCl2 and FeCl3 solutions, the swelling degree barely changed with the temperature rise. This indicated that the swelling process of γ-PGA hydrogels is slightly influenced by the temperature in NaCl solution and is barely affected in CaCl2 and FeCl3 solutions. Compared to poly(N-hydroxyethylacrylamide) hydrogels, γ-PGA hydrogels presented the similar phenomenon that the effect of temperature was unapparent.16
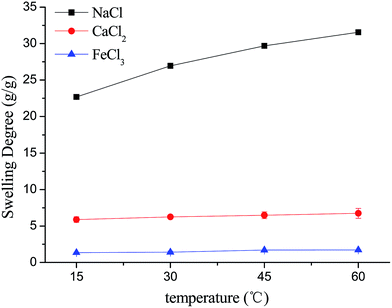 |
| Fig. 2 The effect of temperature on the swelling behavior of γ-PGA hydrogels. | |
3.2.2. The effect of ionic concentration. The effect of solution concentration on the swelling capacity of γ-PGA hydrogels was shown in Fig. 3. Generally, the swelling capacity was decreased with the increase of ionic concentration according to the Flory's theory52 that the charge screening effect of increased additional cations causing a weakened electrostatic repulsion between –COO−, led to a depressed swelling on the polymer network.
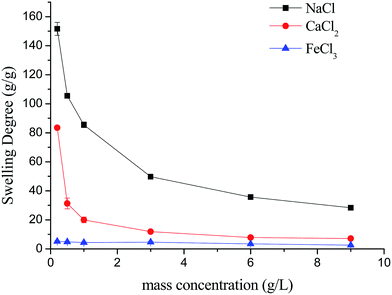 |
| Fig. 3 The effect of solution concentration on the swelling behavior of γ-PGA hydrogels. | |
It is worth noting that the swelling degree of hydrogels was significantly reduced with the ionic valence number advancing. As a result of deswelling of γ-PGA hydrogels, metal ions with a higher valence number also caused a weakened electrostatic repulsion between –COO− due to the chelation between –COO− and metal ions.53
3.2.3. The effect of pH. Fig. 4 depicts the effect of pH on the swelling capacity of γ-PGA hydrogels. As Fe3+ will form precipitates when pH is higher (about 3), the effect of pH in FeCl3 solution was not studied. Generally, increasing pH enhanced the swelling capacity of the hydrogel. This phenomenon was attributed to the theory that the –COO− groups within the network of hydrogels were protonated at a lower pH.52,54 The metal ions had to compete with the proton for absorbency, and the protonated functional groups hindered the interaction between the hydrogel and metal cations.16
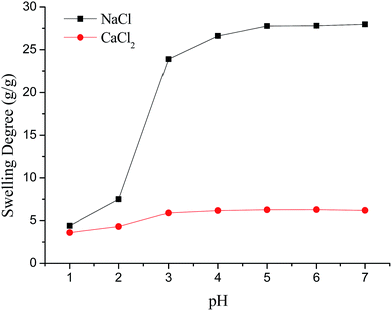 |
| Fig. 4 The effect of pH on the swelling behavior of γ-PGA hydrogels. | |
Scheme 2 described the swelling-deswelling network structure of γ-PGA hydrogels at various pH levels. In low pH range (1–2), there were more cations in the solution, –COO− groups were protonated and the repelling force between –COO− and –COO− was weak. With the increasing of pH, the amounts of –COO− were also increased and this caused a stronger rejection force. As a result, the swelling degree was increased with the increasing of pH.
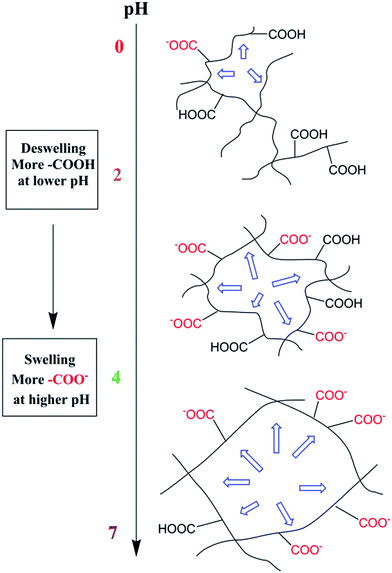 |
| Scheme 2 A scheme of the swelling–deswelling network structure of γ-PGA hydrogels at various pH levels. | |
3.3. Swelling kinetics study
3.3.1. The effect of ionic valence number. As shown in Fig. 5 and Scheme 3, the swelling kinetics of γ-PGA hydrogels in NaCl, CaCl2 and FeCl3 solutions presented different trends. At the beginning, the swelling degree increased rapidly in NaCl, CaCl2 and FeCl3 solutions. Then at around 200 min, the elevation of swelling degrees slowed down in both NaCl and CaCl2 solutions, which surprisingly began to drop in FeCl3 solution. At the last stage, the swelling degrees kept on a steady level in both solutions. Finally, the experimental swelling degrees of γ-PGA hydrogels in NaCl, CaCl2 and FeCl3 solutions were 20.44, 8.47, 1.48 g g−1, respectively.
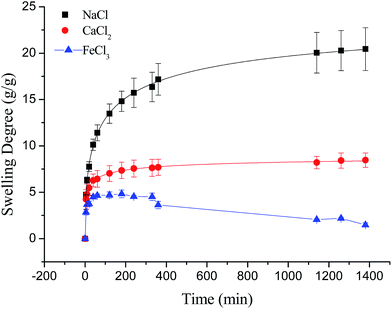 |
| Fig. 5 The swelling kinetics of γ-PGA hydrogels in NaCl, CaCl2 and FeCl3 solutions. | |
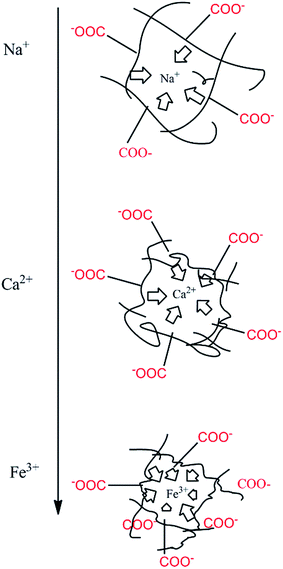 |
| Scheme 3 A scheme of the ionic charge screening effect. | |
Compared to poly(aspartic acid) hydrogels, the swelling kinetics of γ-PGA hydrogels were more likely to be influenced by ions.52 This phenomenon can be explained by the chemical structure of γ-PGA hydrogels which contained a vast amount of –COOH in the polymeric network.55 As a result, γ-PGA hydrogels were more ion-sensitive and presented more limited swelling kinetics in metal ion solutions with higher ionic valence numbers.
3.3.2. The effect of NaCl concentration. To study the effect of NaCl concentration on the swelling kinetics of γ-PGA hydrogels, the swelling degrees at predetermined time were measured and the swelling kinetics of γ-PGA hydrogels was fitted by kinetic models. Among several established kinetic models, the pseudo-first-order and pseudo-second-order kinetic models were the most broadly applied models for investigating the swelling kinetics in ionic solutions such as Pb2+ and Cu2+ ionic solution.16Fig. 6 depicted the plots for pseudo-first-order and pseudo-second-order model in N1, N2 and N3. Table 3 listed the kinetic parameters of pseudo-first-order and pseudo-second-order models. In contrast to the pseudo-first-order, pseudo-second-order model displayed a relatively higher correlation coefficient (Radj2 > 0.99), and had a closer calculated equilibrium swelling degree (Qe,c) to the experimental equilibrium swelling degree (Qe,e). These indicated that the pseudo-second-order model was more appropriate for fitting the experimental kinetics data, and also presented that the rate limiting step of swelling in NaCl was chemisorption or chelation involving valence forces through the sharing or exchange of electrons between the γ-PGA hydrogel and metal ions.16
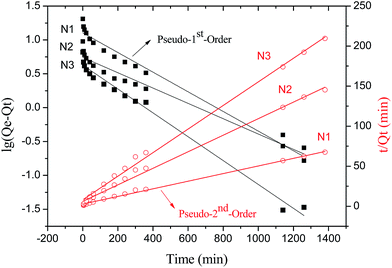 |
| Fig. 6 The plots for pseudo-first and -second-order model in NaCl solution. | |
Table 3 Rate constants and calculated swelling degrees of γ-PGA hydrogels in NaCl solution
|
Qe,e (g g−1) |
Pseudo-first-order |
Pseudo-second-order |
K1 (min−1) |
Qe,c (g g−1) |
Radj2 |
K2 (min−1) |
Qe,c (g g−1) |
Radj2 |
N1 |
20.44 |
0.003339 |
12.71 |
0.9723 |
0.0008349 |
21.03 |
0.9965 |
N2 |
8.39 |
0.002602 |
5.51 |
0.9577 |
0.001775 |
9.68 |
0.9946 |
N3 |
6.05 |
0.004053 |
4.24 |
0.9842 |
0.002703 |
6.79 |
0.9959 |
As shown in Fig. 7, the swelling kinetics of γ-PGA hydrogels in both concentrations presented similar trends. The swelling degrees curves showed a sharper transition from the high initial rate to the slow rate towards the end of the swelling process, which was consistent with the previous study.43,56 Compared to γ-PGA/ε-polylysine hydrogels, γ-PGA hydrogels presented a more limited swelling kinetics and a lower swelling degree in NaCl solution. This is due to the fact that γ-PGA/ε-polylysine hydrogels contained both deprotonatable –COOH and protonatable –NH2. This structure can alleviate the charge screening effect of ions in network.43
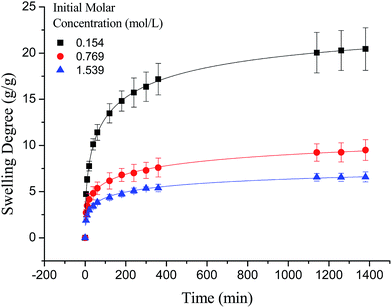 |
| Fig. 7 The swelling kinetics of γ-PGA hydrogels in NaCl solution. | |
3.3.3. The effect of CaCl2 concentration. Similar to the swelling kinetics in NaCl solution, the swelling kinetics of γ-PGA hydrogels in CaCl2 solution also presented typical pseudo-second-order kinetics curves. Fig. 8 showed the plots for pseudo-first-order and pseudo-second-order model in C1, C2 and C3. Table 4 listed the kinetic parameters of pseudo-first-order and pseudo-second-order models. In contrast to the pseudo-first-order, pseudo-second-order model presented a relatively higher correlation coefficient (Radj2 > 0.99), and had a closer calculated equilibrium swelling degree (Qe,c) to the experimental equilibrium swelling degree (Qe,e). These indicated that the pseudo-second-order model was more appropriate for fitting the experimental kinetics data, and also presented that the rate limiting step of swelling in CaCl2 solutions was also chemisorption or chelation involving valence forces through the sharing or exchange of electrons between the γ-PGA hydrogel and metal ions (Fig. 9).
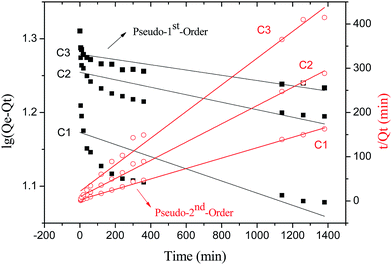 |
| Fig. 8 The plots for pseudo-first and -second-order model in CaCl2 solution. | |
Table 4 Rate constants and calculated swelling degrees of γ-PGA hydrogels in CaCl2 solution
|
Qe,e (g g−1) |
Pseudo-first-order |
Pseudo-second-order |
K1 (min−1) |
Qe,c (g g−1) |
Radj2 |
K2 (min−1) |
Qe,c (g g−1) |
Radj2 |
C1 |
8.47 |
0.000190 |
14.89 |
0.3753 |
0.005049 |
8.50 |
0.9986 |
C2 |
4.79 |
0.000117 |
17.97 |
0.5533 |
0.004540 |
4.85 |
0.9967 |
C3 |
3.33 |
0.000082 |
19.01 |
0.6868 |
0.004230 |
3.32 |
0.9883 |
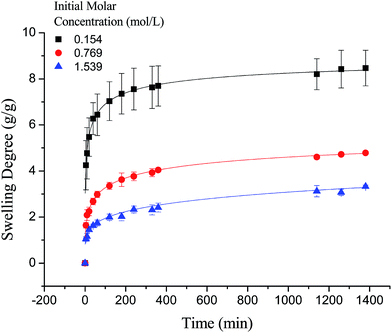 |
| Fig. 9 The swelling kinetics of γ-PGA hydrogels in CaCl2 solution. | |
3.3.4. The effect of FeCl3 concentration. As shown in Fig. 10, the swelling kinetics of γ-PGA hydrogels in FeCl3 solution presented different trends compared to those in NaCl and CaCl2 solutions. At the beginning, the swelling degrees were increased rapidly as was observed in NaCl and CaCl2 solutions. However, when γ-PGA hydrogels swelled at 330 min, the swelling degrees were then decreased and dropped in merely about 5 g g−1. This may be due to the stronger charge screening effect of Fe3+ compared to Na+ and Ca2+. When γ-PGA hydrogels adsorbed Fe3+ ions and reached the critical point, the strong attractive force between –COO− and Fe3+ equal to the total rejection force between –COO− and –COO− and other forces caused swelling at that moment. Then hydrogels adsorbed more ions, the strong attractive force between –COO− and Fe3+ became stronger and caused the collapse of the network in hydrogels. Finally, γ-PGA hydrogels reached equilibrium state as was shown in Table 2, and the swelling degrees in 0.0154 mol L−1 solution dropped from 12.8 to 4.8 g g−1.
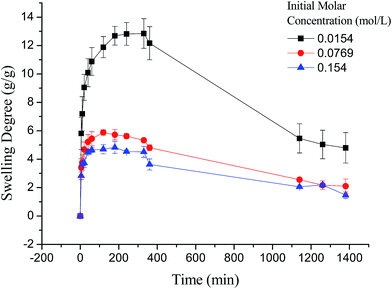 |
| Fig. 10 The swelling kinetics of γ-PGA hydrogels in FeCl3 solution. | |
4. Conclusion
In the study, γ-PGA hydrogels were successfully prepared by EDGE in a simple mixture process. The FTIR proved that ester bonds formed as a crosslinking linkage between γ-PGA and EGDE, indicating that the hydrogel was indeed a copolymer. The morphology of γ-PGA hydrogels that swelled in equilibrium displayed a similar color as swelled ionic solutions and kept a regular cubic shape. The SEM morphology of cross-section showed the porous structure of γ-PGA hydrogels and the densely covered irregularly shaped holes, while the surface morphology showed the rough wrinkled surface of γ-PGA hydrogels without obvious holes. The ionic concentration and pH significantly influenced the swelling capacity of γ-PGA hydrogels whereas the temperature had no significant effects. To be specific, the swelling capacity was decreased with the increase of ionic concentration, and was increased with the increase of pH. The swelling kinetics study indicated that γ-PGA hydrogels were ion-sensitive and presented more limited swelling kinetics in metal ion solutions with higher ionic valence numbers. And the following adsorption process demonstrated a better fitted pseudo-second-order rate equation model. Maximum swelling degree were obtained for γ-PGA hydrogels with 21.03 g g−1 in 0.154 mol L−1 NaCl solution, 8.5 g g−1 in 0.154 mol L−1 CaCl2 solution and 12.8 g g−1 in 0.154 mol L−1 FeCl3 solution.
Overall, γ-PGA hydrogel showed ionic sensitivity and pH sensitivity. Characterization tests and swelling kinetics study indicated that γ-PGA hydrogel could be used in some key applications such as wound dressing, absorbent, pH-sensitive smart material, etc.
Acknowledgements
This work was financially supported by the National Natural Science Foundation of China (No. 31200719, 51403152, 51473122), the Natural Science Foundation of Tianjin (16JCTPJC44400, 14JCQNJC14200).
References
- J. Ahn, L. Kuffova, K. Merrett, D. Mitra, J. V. Forrester, F. Li and M. Griffith, Acta Biomater., 2013, 9, 7796–7805 CrossRef CAS PubMed.
- J. W. Freeman, M. D. Woods, D. A. Cromer, E. C. Ekwueme, T. Andric, E. A. Atiemo, C. H. Bijoux and C. T. Laurencin, J. Biomech., 2011, 44, 694–699 CrossRef PubMed.
- H. Lin, C. Peng and W. Wu, J. Mater. Sci.: Mater. Med., 2014, 25, 259–269 CrossRef CAS PubMed.
- J. Peng, T. Qi, J. Liao, B. Chu, Q. Yang, W. Li, Y. Qu, F. Luo and Z. Qian, Biomaterials, 2013, 34, 8726–8740 CrossRef CAS PubMed.
- J. S. Im, J. Yun, Y. Lim, H. Kim and Y. Lee, Acta Biomater., 2010, 6, 102–109 CrossRef CAS PubMed.
- N. Han, P. A. Bradley, J. Johnson, K. S. Parikh, A. Hissong, M. A. Calhoun, J. J. Lannutti and J. O. Winter, J. Biomater. Sci., Polym. Ed., 2013, 24, 2018–2030 CrossRef CAS PubMed.
- R. C. F. Leitao, C. P. de Moura, L. R. D. Da Silva, N. M. P. S. Ricardo, J. P. A. Feitosa, E. C. Muniz, A. R. Fajardo and F. H. A. Rodrigues, Quim. Nova, 2015, 38, 370–377 CAS.
- Z. Mirzakhanian, K. Faghihi, A. Barati and H. R. Momeni, J. Biomater. Sci., Polym. Ed., 2015, 26, 1439–1451 CrossRef CAS PubMed.
- J. Yu, H. Zhang, Y. Li, Q. Lu, Q. Wang and W. Yang, Colloid Polym. Sci., 2016, 294, 257–270 CAS.
- J. P. D. Garcia, M. Hsieh, B. T. Doma, D. C. Peruelo, I. Chen and H. Lee, Polymers, 2013, 6, 39–58 CrossRef.
- S. C. Shukla, A. Singh, A. K. Pandey and A. Mishra, Biochem. Eng. J., 2012, 65, 70–81 CrossRef CAS.
- A. B. Scranton, B. Rangarajan and J. Klier, in ed. N. Peppas and R. Langer, Biomedical applications of polyelectrolytes, Springer, Berlin, Heidelberg, 1995, vol. 122, pp. 1–54 Search PubMed.
- D. Ji, T. Kuo, H. Wu, J. Yang and S. Lee, Carbohydr. Polym., 2012, 89, 1123–1130 CrossRef CAS PubMed.
- T. K. Giri, A. Thakur, A. Alexander, Ajazuddin, H. Badwaik and D. K. Tripathi, Acta Pharm. Sin. B, 2012, 2, 439–449 CrossRef CAS.
- Y. Lee, Carbohydr. Polym., 2012, 809–819 CrossRef CAS.
- R. Hua and Z. Li, Chem. Eng. J., 2014, 249, 189–200 CrossRef CAS.
- E. H. Lee, H. Uyama, O. H. Kwon and M. H. Sung, Polym. Bull., 2009, 63, 735–742 CrossRef CAS.
- S. Chung, C. Gentilini, A. Callanan, M. Hedegaard, S. Hassing and M. M. Stevens, J. Mater. Chem. B, 2013, 1, 1397–1401 RSC.
- J. Yan, Z. Li, J. Zhang and C. Qiao, Adv. Mater. Res., 2012, 476–478, 2100–2104 CAS.
- A. Chetouani, M. Elkolli, M. Bounekhel and D. Benachour, Polym. Bull., 2014, 71, 2303–2316 CrossRef CAS.
- I. Bajaj and R. Singhal, Bioresour. Technol., 2011, 102, 5551–5561 CrossRef CAS PubMed.
- I. Shih, P. Wu and C. Shieh, Process Biochem., 2005, 40, 2827–2832 CrossRef CAS.
- A. R. Bhat, V. U. Irorere, T. Bartlett, D. Hill, G. Kedia, M. R. Morris, D. Charalampopoulos and I. Radecka, AMB Express, 2013, 3, 1–9 CrossRef PubMed.
- A. R. Bhat, V. U. Irorere, T. Bartlett, D. Hill, G. Kedia, D. Charalampopoulos, S. Nualkaekul and I. Radecka, Int. J. Food Microbiol., 2015, 196, 24–31 CrossRef CAS PubMed.
- T. Akao, T. Kimura, Y. Hirofuji, K. Matsunaga, R. Imayoshi, J. Nagao, T. Cho, H. Matsumoto, S. Ohtono, J. Ohno, K. Taniguchi and H. Kaminishi, J. Drug Targeting, 2010, 18, 550–556 CrossRef CAS PubMed.
- F. L. Mi, Y. Y. Wu, Y. H. Lin, K. Sonaje, Y. C. Ho, C. T. Chen, J. H. Juang and H. W. Sung, Bioconjugate Chem., 2008, 19, 1248–1255 CrossRef CAS PubMed.
- Z. Rongli, L. Ling, X. Sheng, Z. Cuige, L. Xiaoya and L. Jing, Colloids Surf., A, 2015, 470, 218–223 CrossRef.
- H. Poo, C. Park, M. Kwak, D. Choi, S. Hong, I. Lee, Y. T. Lim, Y. K. Choi, S. Bae, H. Uyama, C. Kim and M. Sung, Chem. Biodiversity, 2010, 7, 1555–1562 CAS.
- X. Yu, M. Wang, Q. Wang and X. Wang, in Biosynthesis of polyglutamic acid and its application on agriculture, 2011, vol. 183–185, pp. 1219–1223 Search PubMed.
- C. T. Tsao, C. H. Chang, Y. Y. Lin, M. F. Wu, J. L. Wang, J. L. Han and K. H. Hsieh, Carbohydr. Res., 2010, 345, 1774–1780 CrossRef CAS PubMed.
- S. H. Choi, K. S. Whang, J. S. Park, W. Y. Choi and M. H. Yoon, Macromol. Res., 2005, 13, 339–343 CrossRef CAS.
- J. S. Im, B. C. Bai, S. J. In and Y. Lee, J. Colloid Interface Sci., 2010, 346, 216–221 CrossRef CAS PubMed.
- N. Bereli, D. Turkmen, K. Kose and A. Denizli, Mater. Sci. Eng., C, 2012, 32, 2052–2059 CrossRef CAS.
- F. Y. Siao, J. F. Lu, J. S. Wang, B. S. Inbaraj and B. H. Chen, J. Agric. Food Chem., 2009, 57, 777–784 CrossRef CAS PubMed.
- T. L. Wang, T. H. Kao, B. S. Inbaraj, Y. T. Su and B. H. Chen, J. Agric. Food Chem., 2010, 58, 12562–12567 CrossRef CAS PubMed.
- G. Yang, N. Wang and C. Zhang, Adv. Mater. Res., 2011, 183–185, 125–129 CAS.
- I. B. Bajaj and R. S. Singhal, Food Bioprocess Technol., 2011, 4, 745–752 CrossRef CAS.
- C. Zhao, Y. Zhang, X. Wei, Z. Hu, F. Zhu, L. Xu, M. Luo and H. Liu, Appl. Biochem. Biotechnol., 2013, 170, 562–572 CrossRef CAS PubMed.
- B. Mandal and S. K. Ray, Mater. Sci. Eng., C, 2014, 44, 132–143 CrossRef CAS PubMed.
- W. Zeng, W. Hu, H. Li, Y. Jing, H. Kang, Q. Jiang and C. Zhang, Chin. J. Polym. Sci., 2014, 32, 1507–1514 CrossRef CAS.
- S. Lu, Y. Ning, Z. Hao, C. Li, T. Lei, W. Yen, L. Hui and L. Ying, Mater. Sci. Eng., C, 2015, 48, 533–540 CrossRef PubMed.
- J. Park, W. Choi, S. Choi and M. Yoon, J. Appl. Biol. Chem., 2005, 48, 213–217 CAS.
- H. Jiachuan, L. Zheng, X. Wen, Y. Ning, G. Jixian, Z. Jianfei and Q. Changsheng, Mater. Sci. Eng., C, 2016, 61, 879–892 CrossRef PubMed.
- X. Yang, in Preparation and characterization of gamma-poly(glutamic acid) copolymer with glycol diglycidyl ether, 2011, vol. 8, pp. 11–15 Search PubMed.
- F. Martello, A. Tocchio, M. Tamplenizza, I. Gerges, V. Pistis, R. Recenti, M. Bortolin, M. Del Fabbro, S. Argentiere, P. Milani and C. Lenardi, Acta Biomater., 2014, 10, 1206–1215 CrossRef CAS PubMed.
- M. Kunioka and H. Choi, J. Environ. Polym. Degrad., 1996, 4, 123–129 CrossRef CAS.
- J. Park, W. Choi, S. Choi and M. Yoon, J. Appl. Biol. Chem., 2005, 48, 213–217 CAS.
- D. Huang, Z. Peng, Z. Hu, S. Zhang, J. He, L. Cao, Y. Zhou and F. Zhao, React. Funct. Polym., 2013, 73, 168–174 CrossRef CAS.
- Z. Wu, D. Huang, Z. Hu, Y. Zhou, F. Zhao, Z. Peng and B. Wang, Fibers Polym., 2014, 15, 1146–1152 CrossRef CAS.
- W. Shige, C. Xueyan, S. Mingwu, G. Rui, I. Banyai and S. Xiangyang, Colloids Surf., B, 2012, 89, 254–264 CrossRef PubMed.
- M. A. Mekewi and A. S. Darwish, Mater. Res. Bull., 2015, 70, 607–620 CrossRef CAS.
- Y. Zhao, H. Su, L. Fang and T. Tan, Polymer, 2005, 46, 5368–5376 CrossRef CAS.
- T. Tsujimoto, J. Kimura, Y. Takeuchi, H. Uyama, C. Park and M. Sung, J. Microbiol. Biotechnol., 2010, 20, 1436–1439 CrossRef CAS PubMed.
- G. R. Mahdavinia, A. Pourjavadi, H. Hosseinzadeh and M. J. Zohuriaan, Eur. Polym. J., 2004, 40, 1399–1407 CrossRef CAS.
- N. Lee, T. Go, S. Lee, S. Jeong, G. Park, C. Hong and H. Son, Saudi J. Biol. Sci., 2014, 21, 153–158 CrossRef CAS PubMed.
- H. Omidian, S. A. Hashemi, P. G. Sammes and I. Meldrum, Polymer, 1998, 39, 6697–6704 CrossRef CAS.
|
This journal is © The Royal Society of Chemistry 2017 |