DOI:
10.1039/C6RA26167A
(Paper)
RSC Adv., 2017,
7, 11414-11419
Room temperature methane sensing properties of α-Fe2−xCuxO3 nanoparticles
Received
2nd November 2016
, Accepted 1st February 2017
First published on 14th February 2017
Abstract
α-Fe2−xCuxO3 (0 ≤ x ≤ 0.17) nanoparticles were synthesized using an improved homogenous co-precipitation method. The effects of doped Cu on crystal structure of α-Fe2O3 were investigated by field emission transmission electron microscopy, X-ray diffraction and Raman spectroscopy. Owing to the substitution of Cu2+ at the Fe3+ sites, the lattice parameters of α-Fe2−xCuxO3 with an average particle size of ∼40 nm and a single corundum structure increased with increasing doped Cu concentration. The shifting of Raman peaks for α-Fe2−xCuxO3 to higher wavenumber was also observed due to the strong electron–phonon interactions and structural distortion after doping Cu. According to the conductivity measurements, doping Cu can increase the concentration of hole carriers and enhance the conductivity of the p-type α-Fe2O3 semiconductor. Furthermore, CH4 sensing characterization showed that the α-Fe2−xCuxO3 nanoparticles are sensitive and have a good selectivity to CH4 at room temperature, and the response of the material is evidently improved by doping with Cu. The maximum response to 2000 ppm CH4 at room temperature and 50% relative humidity was obtained when x ≈ 0.10, meeting the common requirements in application. These results suggest that α-Fe2−xCuxO3 nanoparticles can be considered a potential candidate for methane detection at room temperature.
1. Introduction
Methane (CH4), the second potent greenhouse gas, is often found in environment, industrial and domestic areas.1–3 It is the major component of natural gas, which is used widely in industries, automobiles and homes. However, it is necessary to detect CH4 from coal mines, factories, farmland ventilation or natural gas-fueled appliances because it can form an explosive mixture in air if its concentration is in the range of 5–15%. There are several traditional techniques for CH4 detection, such as gas chromatography equipped with a flame ionization detector (GC-FID)4,5 or infrared spectral radiometry.6 However, these common measurement methods for CH4 have some disadvantages, requiring high cost equipment and strict working conditions. In the past few decades, metal-oxide semiconductors, such as SnO2,2 α-Fe2O3,7 and ZnO,8 have been investigated widely as CH4 sensing materials because of their high response and simplicity in synthesis. Although these metal-oxide semiconductors have more advantages for CH4 detection, there are also some problems including their low selectivity, particularly high working temperature (about 400–500 °C). The identification of CH4 at temperatures lower than 350 °C is relatively difficult owing to its high thermodynamic stability compared to that of other hydrocarbons.3 High working temperature in an atmosphere containing CH4 is dangerous and may result in an explosion. Hence, there is strong demand for a reliable sensor that can detect CH4 at or near room temperature. Nowadays, much effort has been devoted to reduce the gas-sensing temperature of metal oxides. For example, materials, such as Pd and Pt, catalyzing CH4 decomposition at a relatively low temperature of 300 °C are loaded mainly in the stable metal oxides.2,9 Unfortunately, the loading of noble metals has high cost and causes environmental pollution. Recently, great emphasis is being given on nanostructured4,10 and doped metal11 oxides as sensor materials to reduce the gas-sensing temperature. Nevertheless, the operating temperature of the vast majority of CH4 sensors is not room temperature, and further studies are still necessary.
As a result, in the present study, α-Fe2−xCuxO3 nanoparticles were selected and synthesized by an improved homogenous co-precipitation method. Based on the characteristics for the morphology, crystal structure and electric conductivity, the effects of doped Cu on crystal structure distortion and semiconducting property were investigated. The CH4 sensing properties of the α-Fe2−xCuxO3 nanoparticles were then examined. As expected, the sensor using α-Fe2−xCuxO3 nanoparticles as a gas sensing material exhibited a receivable response to CH4 at room temperature.
2. Experimental
All the chemical reagents were analytically pure without further purification. α-Fe2−xCuxO3 nanoparticles were prepared by an improved homogenous co-precipitation method. First, FeCl3·6H2O and CuCl2·2H2O were mixed and dissolved with different mol ratios of n(Cu2+)/n(Fe3+) at a given constant Fe3+ concentration of 0.10 mol L−1 (shown in Table 1), which was marked as solution A. Ammonia was diluted five times with distilled water according to the volume ratio, marked as solution B. Solutions A and B were dropped slowly into a distilled water reaction medium, in which sodium stearate was used as the dispersing agent. During the reaction, the reaction medium was stirred by a magnetic stirring apparatus at 20 °C, and the dropping speeds of solutions A and B were controlled at a certain ratio to maintain the pH value of the entire system at about 3. The formed precipitates were centrifugally separated, washed, heated at 60 °C for 10 h, and then annealed at 300 °C in an oxygen atmosphere for 1 h.
Table 1 Mol ratio of n(Cu2+)/n(Fe3+) for preparing the hydroxide of α-Fe2−xCuxO3 nanoparticles
Sample ID |
Value of x |
n(Cu2+)/n(Fe3+) |
Sample ID |
Value of x |
n(Cu 2+)/n(Fe3+) |
CF-0 |
0 |
0 |
CF-5 |
0.10 |
0.05 |
CF-1 |
0.02 |
0.01 |
CF-6 |
0.11 |
0.06 |
CF-2 |
0.04 |
0.02 |
CF-7 |
0.13 |
0.07 |
CF-3 |
0.06 |
0.03 |
CF-8 |
0.15 |
0.08 |
CF-4 |
0.08 |
0.04 |
CF-9 |
0.17 |
0.09 |
Subsequently, α-Fe2−xCuxO3 gas sensors were fabricated as follows. The prepared α-Fe2−xCuxO3 nanoparticles were mixed with the solution of polyvinyl alcohol to form a paste, which was coated onto the α-Al2O3 ceramic sheets (22 mm in length, 4 mm in width, attached with a pair of Ag electrodes and shown as Fig. 1a) using a small brush to form a film with a thickness of around 0.5 mm.
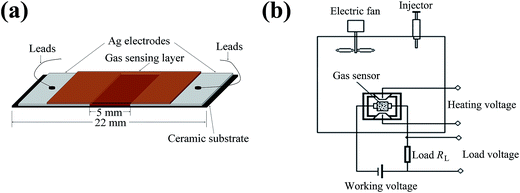 |
| Fig. 1 Schematic of (a) α-Fe2−xCuxO3 gas sensors and (b) equipment for the sensor response measurements. | |
The morphology and crystallization of the α-Fe2−xCuxO3 nanoparticles were observed using a FEI Tecnai G2 F20 field emission transmission electron microscope (TEM) with an acceleration voltage of 200 kV. The structural characterization was performed using a PANalytical X' Pert PRO X-ray diffractometer (XRD) with Cu Kα1 radiation (λ = 0.15406 nm). The vibrational modes and structural distortion of the α-Fe2−xCuxO3 nanoparticles were investigated using a Renishaw inVia confocal microprobe Raman spectroscopy with the laser excitation of 785 nm, which provided a power at the sample surface of ∼1.7 mW and was focused through the 50× objective to a 1–2 μm spot, at room temperature. The electrical property of the α-Fe2−xCuxO3 gas sensors was measured using a two probe technique. The gas sensing experiments were carried out using a static testing method.12 The schematic of the equipment for sensor resistance measurement is shown in Fig. 1b. The response of the sensor was determined by the resistance ratio of sensor device in clean air (Ra) and in an atmosphere containing test gas (Rg), i.e., the sensor response S = Ra/Rg. The value of Ra and Rg can be obtained by measuring the voltage on the load resistance RL.
3. Results and discussion
3.1. Morphological characteristics of the α-Fe2−xCuxO3 nanoparticles
Fig. 2 presents FE-TEM images of α-Fe2−xCuxO3 nanoparticles. Part (a) and (b) show the bright field TEM image and high resolution TEM (HRTEM) image of undoped α-Fe2O3 grains, whereas parts (c) and (d) provide the bright field TEM image and HRTEM image of α-Fe1.92Cu0.08O3, respectively. Fig. 2a shows that the undoped α-Fe2O3 powder consists of irregular particles with sizes in the range from 20 to 80 nm. To obtain more detailed structural information, a corresponding HRTEM image was recorded on the edge of the nanoparticle, shown in Fig. 2b. From the magnified HRTEM image of the selected area (inset of Fig. 2b), the lattice fringes could be observed clearly and the lattice spacing was 0.362 nm, corresponding to the (012) plane of α-Fe2O3. In comparison, the Cu doped α-Fe2O3 (α-Fe1.92Cu0.08O3) particles with a mean particle size of ∼40 nm showed monodispersity (see Fig. 2c). The corresponding HRTEM image (Fig. 2d) displays the (104) plane with the d spacing of 0.266 nm for α-Fe1.92Cu0.08O3. However, the d spacing did not increase significantly as expected, comparing with the value of undoped α-Fe2O3. TEM analysis of α-Fe2−xCuxO3 nanoparticles is comparable to that of the Cu-doped α-Fe2O3 cubes reported in Sun's study.11
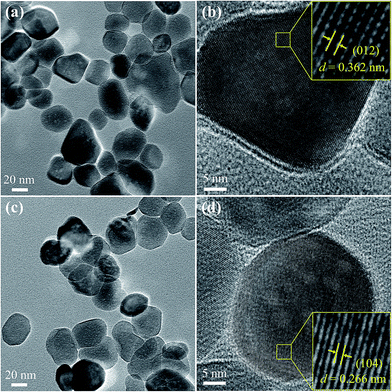 |
| Fig. 2 TEM images of α-Fe2−xCuxO3 nanoparticles: (a) bright field TEM image and (b) HRTEM image showing grain with the (012) plane of undoped α-Fe2O3, (c) bright field TEM image and (d) HRTEM image showing grains with the (104) plane of α-Fe1.92Cu0.08O3. | |
3.2. Structural characteristics of the α-Fe2−xCuxO3 nanoparticles
The XRD patterns of the α-Fe2−xCuxO3 nanoparticles are shown in Fig. 3a. For undoped α-Fe2O3, all the diffraction peaks can be indexed by a single phase with the rhombohedral crystal structure of α-Fe2O3, which is consistent with the standard data file (PDF # 033-0664). For the α-Fe2−xCuxO3 (0 < x ≤ 0.17) nanoparticles, all diffraction peaks can also be successfully indexed to rhombohedral hematite and no other evident trace of impurity phase particularly copper oxide is detected. This indicates that the added Cu could be doped into the crystal structure of α-Fe2O3, retaining the rhombohedral structure of hematite. Furthermore, from the zoomed-in (104) and (110) diffraction peaks of the α-Fe2−xCuxO3 nanoparticles, as seen in Fig. 3b, the diffraction peaks all shifted to lower 2θ angles compared to those of undoped α-Fe2O3, and the offset of peaks increased with increasing doped Cu concentration. To investigate the effects of doped Cu on the crystal structure of α-Fe2O3, the room-temperature lattice parameters of α-Fe2−xCuxO3 nanoparticles were obtained by the software X'Pert HighScore Plus based on the XRD data, as depicted in Fig. 3c. The results show that the lattice parameters a, b and c, all increased with increasing doped Cu concentration. The ionic radii (six-coordination in a rhombohedral structure) of Fe3+ and Cu2+ are similar at 0.0645 nm and 0.073 nm,13 respectively. Therefore, Cu2+ can be doped into the crystal structure of α-Fe2O3 and partly substitute Fe3+. There is no doubt that the lattice parameters may increase when the substitutions exist. Hence, the shift of diffraction peaks and increase in lattice parameters are in association with the substitutions of Fe3+ by Cu2+. In addition, with increasing doped Cu concentration (x), the lattice parameters of α-Fe2−xCuxO3 increased gradually, which was accompanied by the shift of the diffraction peaks to lower 2θ angles.
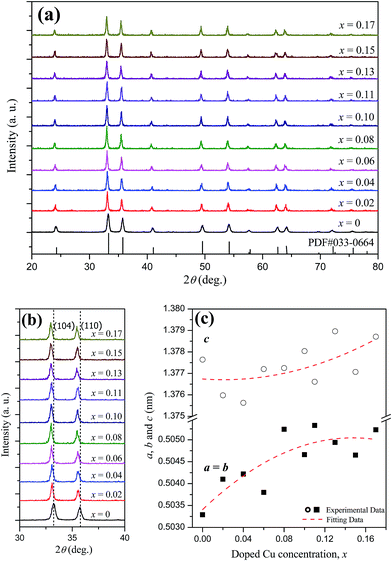 |
| Fig. 3 (a) XRD patterns, (b) zoomed-in (104) and (110) peaks, and (c) room-temperature lattice parameters of α-Fe2−xCuxO3 nanoparticles. | |
In addition, to further investigate the structural distortion of Cu-doped α-Fe2O3 nanoparticles, the Raman spectra of the α-Fe2−xCuxO3 nanoparticles at room temperature were collected (see Fig. 4). The Raman peaks located at 222 cm−1 and 493 cm−1 correspond to A1g modes, whereas the peaks at 241 cm−1, 289 cm−1, 405 cm−1, and 606 cm−1 are assigned to Eg modes. This confirms the corundum structure of α-Fe2−xCuxO3 (0 ≤ x ≤ 0.17), which is consistent with the values reported in the literature.14,15 In general, substitutions of larger ions at the Fe sites may lead to an increase in the mean Fe–O bond length, resulting in a shift of the Raman peaks to lower wavenumbers. However, in the present study, it is puzzling that all of the Raman peaks of Cu doped α-Fe2O3 shifted slightly to higher wavenumbers, compared to those of undoped α-Fe2O3. According to Yogi and Varshney, similar Raman spectrum changes were also observed and attributed to the strong electron–phonon interactions and structural distortion in this system after doping Cu, accompanied with an increase in chemical bond strength.14 These structural changes of the α-Fe2−xCuxO3 nanoparticles induced by doped Cu are expected to have an effect on the electrical and CH4 sensing properties.
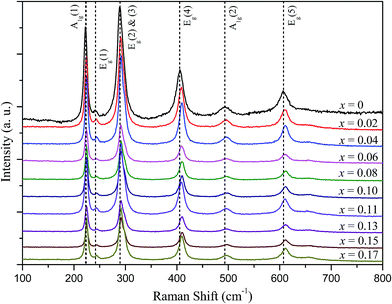 |
| Fig. 4 Raman spectra of α-Fe2−xCuxO3 nanoparticles at room temperature. | |
3.3. Electrical properties of the α-Fe2−xCuxO3 gas sensors
Fig. 5 shows the conductivities of the α-Fe2−xCuxO3 gas sensors at room temperature. The conductivities of the sensors were calculated using eqn (1): |
 | (1) |
where σ is the conductivity, ρ is the resistivity, L is the distance of the Ag electrodes, R is the resistance of α-Fe2−xCuxO3 gas sensor and S is the cross-sectional area of α-Fe2−xCuxO3 layer. As shown in Fig. 5, the conductivity of the α-Fe2−xCuxO3 gas sensor increased from 1.2 × 10−5 to 6.3 × 10−5 S m−1 with increasing x in α-Fe2−xCuxO3 from 0 to 0.17. Clearly, Cu doping enhances the electric conduction capability of α-Fe2O3 gas sensor greatly.
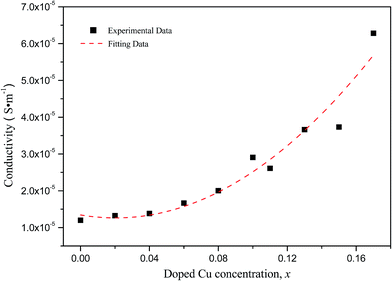 |
| Fig. 5 Conductivities of α-Fe2−xCuxO3 gas sensors at room temperature. | |
According to Long's research,16 α-Fe2O3 annealed in air is an n-type semiconductor and the one annealed in oxygen usually a p-type semiconductor because a large amount of metallic ionic vacancies (point defects) will be formed if annealed in an oxygen atmosphere. These metallic ionic vacancies can accept electrons, and the holes are the majority carriers in α-Fe2O3 semiconductor material. For Cu doped α-Fe2O3, when a part of Fe3+ sites are occupied by Cu2+, holes with a +1 charge are formed simultaneously. This will no doubt increase the concentration of hole carriers and enhance the conductivity of the p-type α-Fe2O3 semiconductor. On the other hand, acceptor energy level (usually called isolated energy level) can be introduced between the valence band and conduction band if the substitutions of Cu2+ ions occur at the Fe sites. The acceptor impurities on the acceptor energy level will ionize and lead to an increase in conductive holes in the valence band. As a result, the conduction capability of the Fe2O3 semiconductor is enhanced, and the conductivity of the α-Fe2−xCuxO3 sensor increases with increasing doped Cu concentration.
3.4. CH4 sensing properties of the α-Fe2−xCuxO3 gas sensors
Fig. 6 depicts CH4 gas response of the α-Fe2−xCuxO3 gas sensors in an atmosphere containing CH4 (2000 ppm) at 25 °C and 50% relative humidity (RH); the inset shows the gas response of the undoped α-Fe2O3 and α-Fe1.92Cu0.08O3 gas sensors to CH4 with different concentrations at 25 °C and 50% RH. The undoped α-Fe2O3 nanoparticles have a lower response to 2000 ppm CH4 gas at room temperature. In contrast, the response of the α-Fe2−xCuxO3 (0 < x ≤ 0.17) gas sensor is significantly higher at room temperature and at the same concentration of CH4 gas. The maximum response to 2000 ppm CH4 at room temperature was obtained when x in α-Fe2−xCuxO3 ≈ 0.10. Although the maximum response is only ∼1.06, this material is also a potential candidate for methane gas sensor applications because the working temperature is much lower in the present case. The sensor using α-Fe2−xCuxO3 nanoparticles as gas sensing material exhibits a receivable response to CH4 at room temperature. The inset in Fig. 6 indicates that the response increased almost linearly with increasing concentration of CH4 gas from 500 to 4000 ppm, meeting the common requirements in application.
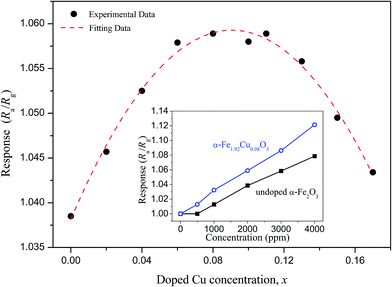 |
| Fig. 6 CH4 gas response of the α-Fe2−xCuxO3 gas sensors in an atmosphere containing CH4 (2000 ppm) at 25 °C and 50% RH. The inset shows the gas response of undoped α-Fe2O3 and α-Fe1.92Cu0.08O3 gas sensors to CH4 with different concentrations at 25 °C and 50% RH. | |
Moreover, for environmental monitoring of methane emissions at room temperature, the selectivity of the α-Fe2−xCuxO3 gas sensors was also evaluated. Fig. 7a shows the response of the α-Fe2−xCuxO3 gas sensors to different test gases (1000 ppm), i.e., CO2, C2H5OH and NH3, at 25 °C and 50% RH. In the CO2 sensing testing, only 700 ppm CO2 was injected because clean air contains around 300 ppm CO2. This shows that the response of the sensors to CH4 is the highest, indicating that the α-Fe2−xCuxO3 sensor has good selectivity for CH4, even though it exhibits a certain response to C2H5OH. As expected, Cu doping can significantly improve the selectivity of the sensor for CH4. Fig. 7b displays the effect of humidity on the sensor response at 25 °C. Water vapors in the atmosphere had an evident effect on the performance of the α-Fe2−xCuxO3 sensor; the response to CH4 was higher in dryer air than in a humid environment. The adsorption of water affects the electronic properties of α-Fe2−xCuxO3, usually acting as a donor. Thus, long exposure in a humid environment can lead to hydration of the surface layer, and a corresponding drift of the sensor performance.17 However, avoiding or mitigating the impact of water vapor is a major problem for gas sensors working at room temperatures. Although some coatings, for example, Teflon, have been used to either consume water vapor or permit the passage of selected gases to the sensor,18 further related research is needed.
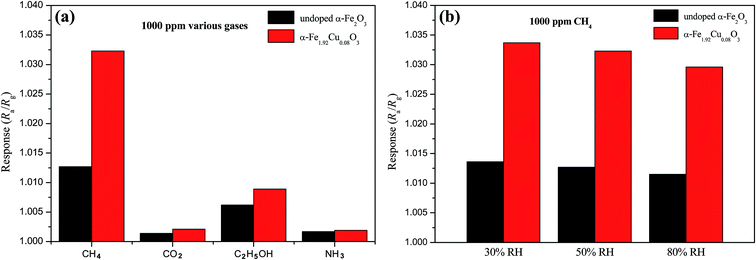 |
| Fig. 7 (a) Response of the α-Fe2−xCuxO3 gas sensors to different test gases at 25 °C and 50% RH. (b) Effect of humidity on the sensor response to 1000 ppm CH4 at 25 °C. | |
The CH4 sensing mechanism (depicted in Fig. 8) of α-Fe2−xCuxO3 nanoparticles is a surface controlled process and can be understood as follows. In vacuum, there are a great number of unstable active sites on the surface of the p-type α-Fe2−xCuxO3 semiconductor, with holes as majority carriers. When α-Fe2−xCuxO3 nanoparticles are exposed to air, a certain amount of oxygen from the air is adsorbed on the surface of α-Fe2−xCuxO3 in the form of O2− or O−, by transferring electrons from the conduction band to the adsorbed oxygen atoms. In this process, the formation of chemisorbed oxygen species results in a decrease in the free electron concentration in the material conduction band. Consequently, the conductivity of the p-type semiconductor will increase.10,11 It should be noted that the amount of chemisorbed oxygen species may be affected, even decreased, with the increasing adsorption of water vapor on the surface of α-Fe2−xCuxO3, which is not conducive to improving the gas sensing performance. Once the α-Fe2−xCuxO3 nanoparticles are exposed to an atmosphere containing CH4, surface reaction between the adsorbed oxygen species and CH4 molecules will occur, resulting in the release of electrons trapped in the ionized oxygen species back into the materials and electron–hole recombination, thereby decreasing the concentration of hole carriers and lowering the measured conductivity.
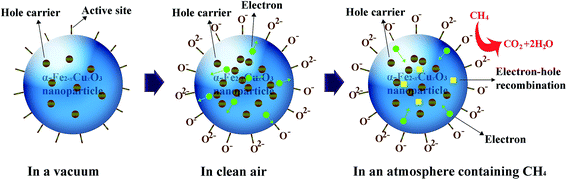 |
| Fig. 8 Schematic explaining the methane sensing mechanism of α-Fe2−xCuxO3 nanoparticles. | |
4. Conclusion
α-Fe2−xCuxO3 (0 ≤ x ≤ 0.17) nanoparticles were synthesized by an improved homogenous co-precipitation method. The morphological and structural characteristics showed that α-Fe2−xCuxO3 (0 < x ≤ 0.17) nanoparticles with an average particle size of ∼40 nm also have a single phase with a rhombohedral corundum structure. Owing to the substitution of Cu2+ at Fe3+ sites, the lattice parameters of α-Fe2−xCuxO3 increase with increasing doped Cu concentration. The shift of Raman peaks for α-Fe2−xCuxO3 to higher wavenumbers was observed due to the strong electron–phonon interaction and structural distortion after doping Cu. Doping Cu can also increase the concentration of hole carriers and enhance the conductivity of p-type α-Fe2O3 semiconductor. The CH4 sensing characterization show that the α-Fe2−xCuxO3 nanoparticles are sensitive and have good selectivity to CH4 at room temperature, and the response of the α-Fe2−xCuxO3 (0 < x ≤ 0.17) gas sensor is significantly improved by doping Cu. The maximum response to 2000 ppm CH4 at room temperature and 50% RH was obtained when x in α-Fe2−xCuxO3 ≈ 0.10, meeting the common requirements in application. Based on these results, the α-Fe2−xCuxO3 (0 < x ≤ 0.17) nanoparticles can be considered potential candidates for methane detection at room temperature.
Acknowledgements
This study was supported by the National Natural Science Foundation of China (grant number 51502249); the Scientific Research Fund of Education Department of Sichuan Province (grant numbers 16ZA0133 and 15ZB0108); and the Doctoral Foundation of Southwest University of Science and Technology (grant number 15zx7105).
References
- J. Hill, C. McSweene, A. D. G. Wright, G. Bishop-Hurley and K. Kalantar-zadeh, Trends Biotechnol., 2016, 34, 26 CrossRef CAS PubMed.
- Z. K. Horastani, S. M. Sayedi, M. H. Sheikhi and E. Rahimi, Mater. Sci. Semicond. Process., 2015, 35, 38 CrossRef.
- A. Das, V. Bonu, A. K. Prasad, D. Panda, S. Dhara and A. K. Tyagi, J. Mater. Chem. C, 2014, 2, 164 RSC.
- T. Sarangi, M. Naja, S. Lal, S. Venkataramani, P. Bhardwaj, N. Ojha, R. Kumar and H. C. Chandola, Atmos. Environ., 2016, 125, 450 CrossRef CAS.
- S. Maduskar, A. R. Teixeira, A. D. Paulsen, C. Krumm, T. J. Mountziaris, W. Fan and P. J. Dauenhauer, Lab Chip, 2015, 15, 440 RSC.
- J. A. Sinclair, P. G. J. Irwin, S. B. Calcutt and E. L. Wilson, Icarus, 2015, 260, 103 CrossRef CAS.
- B. Dong, Z. Han, Y. B. Zhang, Y. Y. Yu, A. G. Kong and Y. K. Shan, Chem.–Eur. J., 2016, 22, 2046 CrossRef CAS PubMed.
- J. Hu, F. Q. Gao, Z. T. Zhao, S. B. Sang, P. W. Li, W. D. Zhang, X. T. Zhou and Y. Chen, Appl. Surf. Sci., 2016, 363, 181 CrossRef CAS.
- S. M. Sedghi, Y. Mortazavi and A. Khodadadi, Sens. Actuators, B, 2010, 145, 7 CrossRef.
- A. Amutha, S. Amirthapandian, A. K. Prasad, B. K. Panigrahi and P. Thangadurai, J. Nanopart. Res., 2015, 17, 289 CrossRef.
- P. Sun, C. Wang, X. Zhou, P. F. Cheng, K. Shimanoe, G. Y. Lu and N. Yamazoe, Sens. Actuators, B, 2014, 193, 616 CrossRef CAS.
- H. F. Liu, T. J. Peng, H. J. Sun, L. Fan and B. G. Guo, Adv. Mater. Res., 2010, 96, 105 CrossRef CAS.
- R. D. Shannon, Acta Crystallogr., 1976, 32, 751 CrossRef.
- A. Yogi and D. Varshney, Mater. Sci. Semicond. Process., 2014, 21, 38 CrossRef CAS.
- L. Kopanja, I. Milosevic, M. Panjan, V. Damnjanovic and M. Tadic, Appl. Surf. Sci., 2016, 362, 380 CrossRef CAS.
- N. V. Long, Y. Yang, M. Yuasa, C. M. Thi, Y. Cao, T. Nann and M. Nogami, RSC Adv., 2014, 4, 8250 RSC.
- A. Mirzaei, B. Hashemi and K. Janghorban, J. Mater. Sci.: Mater. Electron., 2016, 27, 3109 CrossRef CAS.
- S. Yamaguchi, Mater. Chem., 1981, 6, 505 CrossRef CAS.
|
This journal is © The Royal Society of Chemistry 2017 |