DOI:
10.1039/C6RA24689C
(Paper)
RSC Adv., 2017,
7, 5322-5330
High fat diet induces alterations to intraepithelial lymphocyte and cytokine mRNA in the small intestine of C57BL/6 mice
Received
3rd October 2016
, Accepted 5th December 2016
First published on 7th December 2016
Abstract
The aim of this work was to investigate the possible effect of high fat diet (HFD) induced obesity on iIEL subsets and their cytokine mRNA levels in C57BL/6 mice. Two groups of C57BL/6 mice were kept in separate cages and fed with either a normal diet (control) or HFD. After 12 weeks of treatment the iIELs were isolated and analyzed by FACS. Additionally, the mRNA levels of IL-2, IL-15, TGF-β, TNF-α, IFN-γ granzyme B and perforin were quantified by RT-PCR. We found that HFD induced a proportional shift in TCRγδ+ and TCRαβ+ composition within the iIEL population. Also, a significant increase in the mRNA levels of TNF-α, perforin and granzyme B was detected on iIELs from HFD mice. Our findings may provide important insights into intestinal changes associated to HFD induced obesity, which could eventually lead to intestinal inflammation. However, further investigations are required to elucidate the molecular mechanism of HFD induced changes on iIELs.
Introduction
Worldwide, obesity is one of the most important public health problems. It has been described as a chronic degenerative disease, produced by an imbalance between intake and energy consumption, with contribution of environmental and genetic factors.1,2 Obesity is associated with type 2 diabetes mellitus, enhanced cardiovascular risk, fatty liver, and others metabolic alterations.3–5 Moreover, obesity is strongly linked to chronic low grade systemic inflammation, characterized by increased levels of IL-6, TNF-α and acute phase proteins.6–8 Although the mechanisms underlying the inflammation associated with obesity have not been entirely elucidated, it is well known that adipose tissue is an important source of pro-inflammatory cytokines.9 In addition, biological pro inflammatory mechanisms in gut could also contribute to this phenomenon.10,11
On the other hand, intestinal intraepithelial lymphocytes (iIELs) are an essential mechanism of cellular defense against pathogenic microorganisms. The iIELs have also important immunoregulatory functions, and a potent cytolitic activity.12–15 In mice, similar proportions of TCRαβ and TCRγδ expressing iIELs have been identified in the intestinal compartment, most of these cells are expressing the homodimer CD8αα, which has not been detected on other tissues.16–19 Likewise, CD4−CD8− double negative lymphocytes reside in the small intestine.20 Furthermore, CD4+ lymphocytes are a minor subset in the iIELs compartment, and some of these cells are CD4+CD8+ double positive.21,22 In addition, iIELs include thymus-dependent cells such as TCRαβ+CD8αβ+, as well as thymus-independent cells; such as both TCRαβ+CD8αα+ and TCRγδ+CD8αα+.23–25
iIELs may regulate intestinal functions by producing cytokines, which control intestinal homeostasis and injury. Under pathological environment, iIELs cytokine expression is altered, and these changes could mediate functional modifications in the small intestine. iIELs secrete mainly IFN-γ, TNF-α, IL-2, TGF-β, IL15 and others. This way, cytokines produced by iIELs are an important way to modulate the local intestinal immune response, as well as keep the integrity of the intestinal barrier.26
iIELs development is dependent of different factors, including intestinal microbiota,27,28 specific TCR engagement,29,30 and vitamin D.31 On the other hand, it has been reported that high fat diet (HFD) is associated with important changes in gut microbiota, triggering an inflammatory response;11,32 however, the possible changes on the iIELs subsets have not been explored on HFD induced obesity mice model. Herein, the effect of HFD on iIELs subsets, as well as mRNA cytokines levels in C57BL/6 mice was determined. We found that HFD induced significant changes in iIEL subsets, together with an increase on mRNA levels of TNF-α, perforin, and granzyme-B. Our data suggest that HFD lead to disrupt the intestinal homeostasis, producing changes on iIELs proportions and increasing mRNA levels of TNF-α, perforin, granzyme-B. Thus, HFD induced intestinal changes may contribute to trigger the inflammation linked to obesity.
Material and methods
Mice and antibodies
Five to seven week old C57BL/6NHsd male mice were purchased from Harlan Mexico, S.A. de C.V. (México, D.F.). Mice were maintained in a pathogen-free environment, under standard room conditions of temperature, humidity and free access to food and water. The mice were maintained in accordance with to the official Mexican guidelines for the use and care of experimental animals, and the protocol was approved by the ethics committee of the Department of Medical Sciences of Universidad de Guanajuato, México.
The following monoclonal antibodies were used for iIELs staining and FACS analysis: FITC-anti-CD8β, PE-anti-TCRγδ, PerCP-anti-CD8α, APC-anti-TCRβ, PerCP-anti-CD4, and purified anti-Fcγ receptor (CD16/CD32.). All anti mouse monoclonal antibodies were purchased from BD Biosciences (San Diego, CA), and diluted 1
:
10 in FACS buffer (PBS, FBS 5%, and sodium azide 0.05%) before use them.
Diet intervention
Two groups of mice (n = 10 each group) were kept in separate cages. One of these designated as a control, fed with a normal diet (2014S Teklad Global 14% protein rodent maintenance diet, 4% fat); whereas another group was fed with a high fat diet for 12 weeks (TD.06414, adjusted calories diet: 60 kcal/fat, 34.3% fat, 27.3% protein). According to the diet manufacturer, the approximate fatty acid profile (% of total fat) is the following: 37% saturated, 47% monounsaturated, 16% polyunsaturated. Both diets were purchased from Harlan Mexico S.A. de C.V. After 12 weeks, mice were sacrificed and the small intestine was removed to isolate and purified the iIELs.
Body weight gain and glucose, cholesterol, and triglyceride serum levels measurement
The weight gain of each mouse was assessed with an analytical balance and recorded every 7 days until the end of the experiment. Blood samples were obtained from the retro-ocular plexus with a capillary microtube and immediately used for glucose, cholesterol and triglyceride determinations, with an Accutrend GCT system (according to the manufacturer's instructions). After that, mice were sacrificed by cervical dislocation and small intestine was collected in cold PBS.
iIELs purification and staining
iIELs were isolated from the small intestine as previously described.33 Briefly, Peyer's patches were identified and removed. After flushing with 50 mL of PBS (4 °C), gut was opened on a wet linen square. The mucosa was scraped with a scalpel and then dissociated by stirring for 20 min at room temperature in 50 mL of RPMI 1640 containing 10% heat inactivated FBS plus 1 mM dithioerythritol (Sigma Chemical Co., St. Louis, MO) and 1 mM EDTA. After centrifugation at 1500 rpm for 10 min, the cell pellet was resuspended in RPMI 1640 and passed through a glass wool column prewashed with RPMI 1640 containing 5 mM HEPES. The suspension obtained was then centrifuged and the pellet used to purify iIELs by centrifugation through a discontinuous Percoll (Amersham Pharmacia Biotech, Piscataway, NJ) gradient. Cells were resuspended in a 72% Percoll/PBS solution, overlayed with an equal volume of 36% Percoll/PBS and centrifuged at 2000 × g for 30 min at room temperature. Then, cells at the interface (iIELs) were, washed and incubated with anti-CD16/CD32 to block nonspecific mAb binding. Cells were then immunostained with different combinations of the indicated mAbs. Four-color multiparameter immunofluorescence staining was analyzed in a FACSCalibur flow cytometer (Becton Dickinson, San Jose, CA). iIELs were gated using forward and side scatter to exclude dead cells, and the data were analyzed using the Cell Quest software (Becton Dickinson, San Jose, CA).
Measurement of cytokine mRNA levels by RT-PCR
Total mRNA was isolated from iIELs using the RNeasy Mini Kit (QIAGEN, Valencia CA), each RNA sample (2 ng) was reverse-transcribed with 50 μM of oligo(dT)20, 10 mM dNTP mix, 0.1 M DTT, and 15 U μL−1 of ThermoScript™ RT-PCR system (Invitrogen, Alameda CA) in a volume of 20 μL at 42 °C for 60 min for synthesis of first-strand cDNA. The PCR amplification was processed in a Perkin Elmer Thermo Cycler 9700 as described above. The PCR products were subjected to electrophoresis in 1.8% agarose gels, stained with ethidium bromide and visualized under UV light. The specific oligonucleotide primers used in PCR amplification were as follows: IL-2 sense, 5′-TGA TGG ACC TAC AGG AGC TCC TGA G-3′; IL-2 antisense, 5′-GAG TCA AAT CCA GAA CAT GCC GCA G-3′; IL-15 sense, 5′-GGA AGG CTG AGT TCC ACA TC-3′; IL-15 antisense, 5′-AGG GAG ACC TAC ACT GAC AC-3′; IFN-γ sense, 5′-AGC GGC TGA CTG AAC TCA GAT TGT AGC TTG TAC CTT TAC TTC ACT G-3′; IFN-γ antisense, 5′-GTC ACA GTT TTC AGC TGT ATAGGG-3′; TNF-α sense, 5′-GGC AGG TCT ACT TTG GAG TCA TTG C-3′; TNF-α antisense, 5′-ACA TTC GAG GCT CCA GTG AAT TCG G-3′; TGF-β sense, 5′-TGG ACC GCA ACA ACG CCA TCT ATG-3′; TGF-β sense, 5′-TGG AGC TGA AGC AAT AGT TGG TAT CC-3′; granzyme B sense, 5′-GCC CAC AAC ATC AAA GAA CAG-3′; granzyme B antisense, 5′-AAC CAG CCA CAT AGC ACA CAT-3′; perforin sense, 5′-GTC ACG TCG AAG TAC TTG GTG-3′; perforin antisense, 5′-ATG GCT GAT AGC CTG TCT CAG-3′; β-actin sense, 5′-TGG, AAT CCT GTG GCA TCC ATG AAA C-3′; β-actin antisense, 5′-TAA AAC GCA GCT CAG TAA CAG TCC G-3′. The β-actin was used as internal control.
Additionally, quantitative real-time polymerase chain reactions (qRT-PCR) were performed using SYBR Green PCR master mix (Applied Biosystems, Foster City, CA) on a StepOnePlus real-time PCR system (Applied Biosystems) following standard procedures. Briefly, cDNA was synthesized from 1 μg of total RNA using High cDNA Reverse Transcription Kit (Applied Biosystems). For PCR reactions, 1 μL of cDNA template, 0.3 μL of forward and reverse primers for specific cytokine (10 μmol L−1 each), 10 μL of SYBR Green PCR Master Mix, and 8.4 μL of water were added to a final volume of 20 μL. Thermal cycling was performed as follows: 95 °C for 3 minutes as initial denaturing, followed by 40 cycles of 94 °C for 30 seconds, 60 °C for 30 seconds, and a final extension at 72 °C for 2 minutes. Threshold cycles (CT values) were determined using the StepOne software (Applied Biosystems). Real-time PCR results were normalized using β-actin as an internal control. Relative mRNA levels were calculated from the CT values for each sample.
Results
Effect of HFD on body weight and plasma triglycerides
After 12 weeks of treatment the body weight of HFD mice increased from 20.6 ± 2.4 to 42 ± 3.7 g, while a slight increase of body weight was observed in mice feed with normal diet (Fig. 1a). In addition, although serum levels of glucose and cholesterol were similar in both groups (Fig. 1b and c), a significant increase on triglycerides was detected in HFD mice (Fig. 1d).
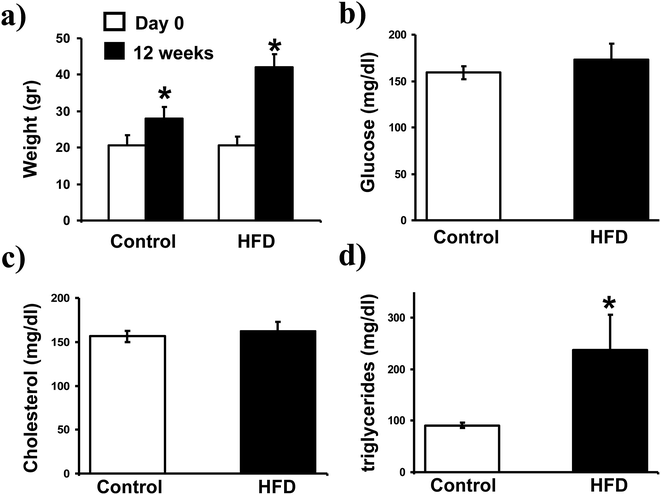 |
| Fig. 1 Body weight gain and glucose, cholesterol, triglycerides levels on plasma. The body weight gain of each mouse was assessed and recorded at day 0 and 12 weeks of the experiment; graph bars in (a) represent the arithmetic mean ± SD of weight in grams (g) of 10 mice. Blood samples were obtained from the retro-ocular plexus to determine glucose, cholesterol, and triglycerides levels with an Accutrend GCT system. The bars in the histograms represent the arithmetic mean ± SD of glucose (b), cholesterol (c) and triglyceride (d) in mg dL−1 of 10 mice. *p < 0.05 by U Mann Whitney. | |
Effect of HFD on TCRγδ iIELs
We found that HFD induced a significant increase on the proportion of TCRγδ iIELs (from 47 ± 3 to 67 ± 7%, p < 0.05, Fig. 2a and b). Accordingly, a significant decrease on the proportion of TCRαβ iIELs was detected in HFD mice (from 44 ± 4 to 23 ± 5%, p < 0.0.5, Fig. 2). In contrast, no significant changes on percentage of both TCRγδ and TCRαβ iIELs were observed in control mice (Fig. 2a and b).
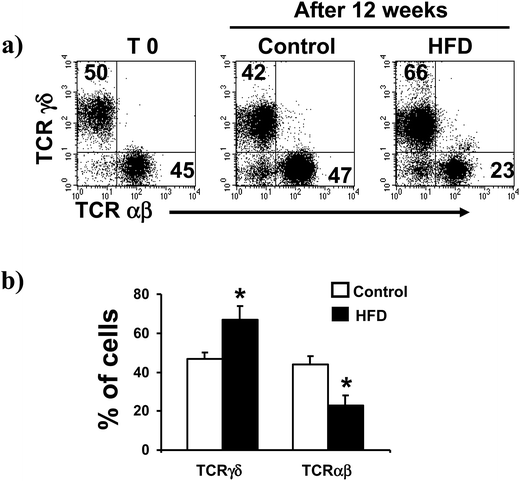 |
| Fig. 2 TCRγδ and TCRαβ expressing cells in the small intestine of control and HFD feed mice. Freshly isolated iIELs were stained with anti TCRγδ, and TCRαβ monoclonal antibodies and analyzed by flow cytometry. (a) Left panel correspond to representative flow cytometry dot plot of TCRγδ and TCRαβ expression on day 0, and middle and right panels correspond to 12 weeks of control and HFD mouse, respectively. (b) HFD induced a significant increase in the proportion of TCRγδ iIELs, and a significant decrease on TCRαβ iIELs. Results are the mean ± SD of 10 mice in each group, *p < 0.05 compared with control mice by U Mann Whitney. | |
HFD had not effect on iIELs expressing CD4, CD8αβ or CD8αα
Additional flow cytometry analysis showed that TCRαβ+CD4+ cells were not affected by HFD, from 4.4 ± 0.8% in control to 4.3 ± 1.0% in HFD mice (Fig. 3a). Likewise, the proportions of both CD8αβ+ and CD8αα subsets gated on TCRαβ+ iIELs were similar in HFD and control mice at the beginning and after 12 weeks of HFD. The percentage of iIELs expressing TCRαβ+CD8αβ+ was 31 ± 1.9% in control and 34 ± 2% in HFD mice; while the percentage of iIELs expressing TCRαβ+CD8αα+ was 55 ± 4% in control and 48 ± 4%, in HFD mice, respectively (Fig. 3b). Similarly, no significant changes were observed in TCRγδ lymphocytes expressing CD8αα or CD8αβ in either HFD or control mice. The percentage of iIELs expressing TCRγδ+CD8αα+ was 88 ± 5% in control and 85 ± 3% in HFD mice; while the percentage of iIELs expressing TCRγδ+CD8αβ was 3.4 ± 4%, in control and 5.5 ± 2% in HFD mice, respectively (Fig. 4).
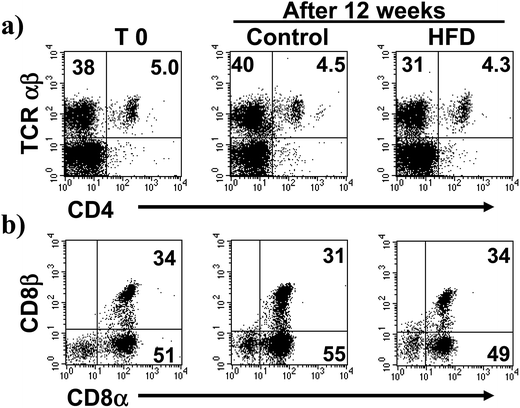 |
| Fig. 3 Subsets on TCRαβ+ expressing cells in the small intestine of control and HFD feed mice. Freshly isolated iIELs were stained with anti TCRγδ, TCRαβ, CD8α and CD8β monoclonal antibodies and analyzed by flow cytometry. (a) Correspond to representative flow cytometry dot plot of TCRαβ+CD4+ expression on day 0 (left panel), and middle and right panels correspond to 12 weeks of control and HFD mice, respectively. (b) Correspond to representative flow cytometry dot plot of CD8α and CD8β expression iIELs gated on TCRαβ+ cells on day 0 (left panel), and middle and right panels correspond to 12 weeks of control and HFD mice, respectively. | |
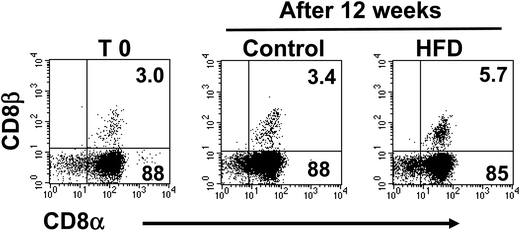 |
| Fig. 4 Subsets on TCRδγ+ expressing cells in the small intestine of control and HFD feed mice. Freshly isolated iIELs were stained with anti TCRγδ, TCRαβ, CD8α and CD8β monoclonal antibodies and analyzed by flow cytometry. Left panel corresponds to representative flow cytometry dot plot of CD8α and CD8β expression iIELs gated on TCRγδ+ cells on day 0, and middle and right panels correspond to 12 weeks of control and HFD mice, respectively. | |
High fat diet induces changes in the pattern of cytokine synthesis by iIELs
To determine the possible effect of HFD on mRNA levels of different cytokines (IL-2, IL-15, TGF-β, TNF-α, IFN-γ) and cytotoxic molecules produced by iIELs, the mRNAs levels were assessed by both conventional and semiquantitative RT-PCR. We found that HFD did not induce changes on mRNA levels of IL2, IL15 nor TGF-β (Fig. 5a and b). In contrast, mRNA levels of TNF-α and perforin were significantly higher in HFD mice compared to control (Fig. 5b). Interestingly, a huge mRNA level of granzyme B was detected in HFD mice, whereas a slightly granzyme B mRNA level was observed in iIELs from control mice (Fig. 5a and b). Although, high mRNA levels of IFN-γ were observed in both HFD and control mice, no significant differences were detected between both groups (Fig. 5a and b).
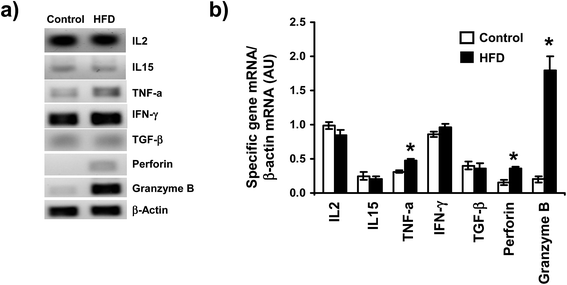 |
| Fig. 5 Cytokines mRNA expression on total iIELs from ND and HFD feed mice. After 12 weeks of treatment, total RNA was isolated from iIELs from control and HFD mice, each mRNA sample was quantified and the levels of mRNA of IL2, IL15, TNFα, IFN-γ, TGF-β, perforin and granzyme B were evaluated by conventional RT-PCR. The β-actin was used as internal control. (a) The PCR products were subjected to electrophoresis in 1.8% agarose gel, stained with ethidium bromide and visualized under UV light. (b) Quantitative real-time polymerase chain reactions (qRT-PCR) were performed using SYBR Green. RT-PCR results were normalized using β-actin as an internal control. Relative mRNA levels were calculated from the CT values for each sample and the ratios were reported as arbitrary units (AU). *p < 0.05 compared with control mice by U Mann Whitney. | |
Discussion
Intraepithelial lymphocytes are localized into the intestinal wall. To date there is not enough information about the mechanisms how iIELs protect the local cells from antigens and pathogenic micro-organisms; neither how cytokines produced by iIELs may regulate the local cellular functions and keep intestinal homeostasis.
It is well known, that consumption of a high fat diet (HFD) is associated with development of obesity in humans and animals. In mice, HFD induce disorders like those that occur in human, such as intestinal inflammation and insulin resistance.32 On the other hand, intestinal intraepithelial lymphocytes (iIELs) play an important role in the local immune response of the small intestine. iIELs are heterogeneous regarding to their phenotype, ontogeny, and functions. iIELs are organized in distinct subsets of T cells expressing either TCRγδ+ or TCRαβ+, with potent cytolytic and immunoregulatory functions. However, the mechanisms and functions of both TCRγδ+ and TCRαβ+ iIELs have not been completely elucidated. Herein, we have determined the effect of HFD induced obesity on iIELs subsets in C57BL/6 mice. Our results clearly have shown that HFD induced a shift on proportion of TCRγδ+ and TCRαβ+ within the iIELs population.
A recent report described that HFD promotes an important decrease in the proportion of iIELs and goblet cells, altering the secretion of hormones as well as maintenance of intestinal barrier; but the TCRγδ+ and TCRαβ+ iIELs subsets were no studied.34 Previous studies reported that different factors, such as pathogens and gut microbiota might change the proportions of iIELs. In this regard, infections with either S. typhimurium, T. gondii, or N. brasiliensis induced the expansion of iIELs expressing TCRγδ; suggesting that TCRγδ contributed to maintain the integrity and function of intestinal epithelial tight junctions and goblet cells during infection.33,35–37 Similarly, beneficial interactions between commensal microbiota and iIELs expressing TCRγδ have been described.38,39
On the other hand, interactions between bacteria and HFD induced important changes in the gut microbiota10 and a proinflammatory response in the small intestine on progression of obesity was triggered.32 Therefore, is clear that HFD induces changes on intestinal environment and iIELs might have an important role inducing local inflammation. However, so far there are few studies to elucidate the functions of iIELs in mice models of obesity.
In our experimental model of HFD induced obesity, the serum levels of cholesterol and glucose were similar between control and HFD mice; but an important increase on triglycerides and body weight was detected in HFD mice.
On this regards, different results on biochemical parameters have been published; some studies have shown that high fat diet increased blood levels of glucose, cholesterol, and triglycerides.40,41 Whereas de Sousa et al. have not observed changes on glucose and triglycerides, but an important increase on cholesterol was detected.42 Similarly, West et al. did not find any difference on glucose blood levels in several mice strains feed with HFD.43 Discordance on these results could be due to different fatty acid profile on diet formulation,42,44,45 time of feeding mice,46,47 and mouse strain used.43,48 Thus, the effect of HFD induced obesity on iIELs was associated firstly with HFD induced obesity and not by other biochemical changes such as high levels of glucose (linked to type 2 diabetes mellitus) or cholesterol (linked to cardiovascular disease).
Moreover, an increase on proportion of TCRγδ+ and a decrease on TCRαβ+ within the iIELs population was found after 12 weeks of HFD. Previously, important changes in the proportions of bowel immune populations in the colon, including a reduced percentage of Tregs and an increase in IL-17-producing γδ T cells, have been reported in mice feed with HFD.49 A similar effect was reported using dietary nucleotides, and this phenomenon was dependent of IL-7 produced by intestinal epithelial cells.50
iIELs modulate important biological cellular functions by their cytotoxic activity and cytokines production on the small intestine.26 Here we have detected increased mRNA levels of TNF-α, perforin, and granzyme B in iIELs from HFD mice. In this regard, a recent report described that in vivo stimulation with anti-CD3 monoclonal antibody activate TCRγδ to release granzyme B, inducing DNA fragmentation on surrounding epithelial cells.51 In addition, a maximum cytotoxic activity of iIELs is preceded by perforin and granzyme B mRNA expression in iIELs after viral infection.52 Additionally, we showed higher mRNA levels of TNF-α in iIELs from HFD compared to control mice, suggesting that HFD promote the iIELs activation and synthesis of this cytokine. Consistently, previous reports have detected important changes on the levels of TNF-α mRNA after HFD feeding.34,53
Interestingly, HFD did not induce any change on mRNA levels of TGF-β, IL2 and IL15; cytokines that promote proliferation and protection of intestinal barrier. Therefore, it is possible that HFD induced damage on intestinal barrier and deregulation on iIELs functions, increasing mRNA levels of TNF-α, perforin, and granzyme B. Previously, it has been described that HFD induced obesity is associated with alteration in occludin distribution and disruption of tight junctions of epithelial cells, a phenomenon that may result in an enhanced stimulation of iIELs and triggering an inflammatory event.11 Curiously, high levels of IFN-γ mRNA were detected on iIELs of both groups, suggesting IFN-γ is not modified by HFD, suggesting that IFN-γ is mainly involved in specific immune response to intestinal damage induced by either bacteria or virus infection.54–56
On the other hand, previous studies by DNA microarrays suggest that TCRγδ+ iIELs could participate in the intestinal lipid metabolism and cholesterol homeostasis.57 Thus, it is possible that increase of TCRγδ+ cells by HFD could be induced by different diet components to maintenance of a stable equilibrium through physiological processes; whereas, TCRαβ+ iIELs may have a more restrictive role on these functions. Therefore, it may reflect selective functions of TCRαβ+ and TCRγδ+ subsets on the intestine compartment of HFD mice. Although, the functional differences among TCRαβ+ and TCRγδ+ are still uncertain.
Conclusions
In summary, we have described that HFD induces important changes on proportion of iIELs as well as a significant increase on mRNA levels of TNF-α, perforin, and granzyme B in whole iIELs population. These findings may provide important insights into intestinal changes produced on HFD induced obesity, which could eventually trigger intestinal inflammation. However, further investigations are required to elucidate the specific molecular mechanism of HFD induced changes on iIELs and cytokines mRNA; primarily, because diet formulation (fatty acid profile) may produce different effect in gut.
Conflict of interest
All authors declare no conflict of interest.
Acknowledgements
This work was supported by CONACYT (Grant number 79561) and DAIP, Universidad de Guanajuato (Grant number 000027/08).
References
- S. O'Rahilly and I. S. Farooqi, Human obesity as a heritable disorder of the central control of energy balance, Int. J. Obes., 2008, 32(suppl. 7), S55–S61 CrossRef PubMed.
- I. S. Farooqi, Genetic, molecular and physiological insights into human obesity, Eur. J. Clin. Invest., 2011, 41, 451–455 CrossRef PubMed.
- A. Bartolomucci, A. Cabassi, P. Govoni, G. Ceresini, C. Cero, D. Berra, H. Dadomo, P. Franceschini, G. Dell'Omo, S. Parmigiani and P. Palanza, Metabolic consequences and vulnerability to diet-induced obesity in male mice under chronic social stress, PLoS One, 2009, 4, e4331 Search PubMed.
- M. Munoz, R. A. Mazure and J. M. Culebras, Obesity and the immune system, Nutr. Hosp., 2004, 19, 319–324 CAS.
- G. S. Hotamisligil, Inflammation and metabolic disorders, Nature, 2006, 444, 860–867 CrossRef CAS PubMed.
- H. Xu, G. T. Barnes, Q. Yang, G. Tan, D. Yang, C. J. Chou, J. Sole, A. Nichols, J. S. Ross, L. A. Tartaglia and H. Chen, Chronic inflammation in fat plays a crucial role in the development of obesity-related insulin resistance, J. Clin. Invest., 2003, 112, 1821–1830 CAS.
- M. F. Gregor and G. S. Hotamisligil, Inflammatory mechanisms in obesity, Annu. Rev. Immunol., 2011, 29, 415–445 CrossRef CAS PubMed.
- A. Syrenicz, B. Garanty-Bogacka, M. Syrenicz, A. Gebala and M. Walczak, Low-grade systemic inflammation and the risk of type 2 diabetes in obese children and adolescents, Neuroendocrinol. Lett., 2006, 27, 453–458 CAS.
- K. Karmiris, I. E. Koutroubakis and E. A. Kouroumalis, The emerging role of adipocytokines as inflammatory mediators in inflammatory bowel disease, Inflammatory Bowel Dis., 2005, 11, 847–855 CrossRef.
- H. Li, C. Lelliott, P. Hakansson, K. Ploj, A. Tuneld, M. Verolin-Johansson, L. Benthem, B. Carlsson, L. Storlien and E. Michaelsson, Intestinal, adipose, and liver inflammation in diet-induced obese mice, Metabolism, 2008, 57, 1704–1710 CrossRef CAS PubMed.
- C. B. de La Serre, C. L. Ellis, J. Lee, A. L. Hartman, J. C. Rutledge and H. E. Raybould, Propensity to high-fat diet-induced obesity in rats is associated with changes in the gut microbiota and gut inflammation, Am. J. Physiol.: Gastrointest. Liver Physiol., 2010, 299, G440–G448 CrossRef CAS PubMed.
- T. Chardes, D. Buzoni-Gatel, A. Lepage, F. Bernard and D. Bout, Toxoplasma gondii oral infection induces specific cytotoxic CD8 alpha/beta+ Thy-1+ gut intraepithelial lymphocytes, lytic for parasite-infected enterocytes, J. Immunol., 1994, 153, 4596–4603 CAS.
- K. W. Beagley and A. J. Husband, Intraepithelial lymphocytes: origins, distribution, and function, Crit. Rev. Immunol., 1998, 18, 237–254 CrossRef CAS PubMed.
- H. Kiyono, K. Fujihashi, T. Taguchi, W. K. Aicher and J. R. McGhee, Regulatory functions for murine intraepithelial lymphocytes in mucosal responses, Immunol. Res., 1991, 10, 324–330 CrossRef CAS PubMed.
- K. Fujihashi, T. Taguchi, J. R. McGhee, J. H. Eldridge, M. G. Bruce, D. R. Green, B. Singh and H. Kiyono, Regulatory function for murine intraepithelial lymphocytes. Two subsets of CD3+, T cell receptor-1+ intraepithelial lymphocyte T cells abrogate oral tolerance, J. Immunol., 1990, 145, 2010–2019 CAS.
- Y. Fujiura, M. Kawaguchi, Y. Kondo, S. Obana, H. Yamamoto, M. Nanno and H. Ishikawa, Development of CD8 alphaalpha+ intestinal intraepithelial T cells in beta 2-microglobulin- and/or TAP1-deficient mice, J. Immunol., 1996, 156, 2710–2715 CAS.
- B. S. Podd, C. Aberg, T. L. Christopher, F. Perez-Cano and V. Camerini, Late postnatal expansion of self-reactive CD8 alphaalpha+ intestinal intraepithelial lymphocytes in mice, Autoimmunity, 2004, 37, 537–547 CrossRef CAS PubMed.
- B. C. Sydora, L. Brossay, A. Hagenbaugh, M. Kronenberg and H. Cheroutre, TAP-independent selection of CD8+ intestinal intraepithelial lymphocytes, J. Immunol., 1996, 156, 4209–4216 CAS.
- L. Gapin, H. Cheroutre and M. Kronenberg, Cutting edge: TCR alphabeta+ CD8 alphaalpha+ T cells are found in intestinal intraepithelial lymphocytes of mice that lack classical MHC class I molecules, J. Immunol., 1999, 163, 4100–4104 CAS.
- M. Ogimoto, G. Matsuzaki, Y. Yoshikai, Y. Tauchi and K. Nomoto, Appearance of TCR-alphabeta+ CD4−CD8− skin intraepithelial lymphocytes in radiation bone marrow chimeras, J. Immunol., 1993, 151, 3000–3006 CAS.
- R. L. Mosley, D. Styre and J. R. Klein, CD4+CD8+ murine intestinal intraepithelial lymphocytes, Int. Immunol., 1990, 2, 361–365 CrossRef CAS PubMed.
- T. Sasahara, H. Tamauchi, N. Ikewaki and K. Kubota, Unique properties of a cytotoxic CD4+CD8+ intraepithelial T-cell line established from the mouse intestinal epithelium, Microbiol. Immunol., 1994, 38, 191–199 CrossRef CAS PubMed.
- O. Neuhaus, M. Emoto, C. Blum, S. Yamamoto and S. H. Kaufmann, Control of thymus-independent intestinal intraepithelial lymphocytes by beta 2-microglobulin, Eur. J. Immunol., 1995, 25, 2332–2339 CrossRef CAS PubMed.
- G. Das and C. A. Janeway Jr, Development of CD8 alpha/alpha and CD8 alpha/beta T cells in major histocompatibility complex class I-deficient mice, J. Exp. Med., 1999, 190, 881–884 CrossRef CAS PubMed.
- S. H. Park, D. Guy-Grand, F. A. Lemonnier, C. R. Wang, A. Bendelac and B. Jabri, Selection and expansion of CD8 alpha/alpha(1) T cell receptor alpha/beta(1) intestinal intraepithelial lymphocytes in the absence of both classical major histocompatibility complex class I and nonclassical CD1 molecules, J. Exp. Med., 1999, 190, 885–890 CrossRef CAS PubMed.
- Y. Qiu and H. Yang, Effects of intraepithelial lymphocyte-derived cytokines on intestinal mucosal barrier function, J. Interferon Cytokine Res., 2013, 33, 551–562 CrossRef CAS PubMed.
- J. J. Cebra, S. B. Periwal, G. Lee, F. Lee and K. E. Shroff, Development and maintenance of the gut-associated lymphoid tissue (GALT): the roles of enteric bacteria and viruses, Dev. Immunol., 1998, 6, 13–18 CrossRef CAS PubMed.
- C. M. Galdeano and G. Perdigon, The probiotic bacterium Lactobacillus casei induces activation of the gut mucosal immune system through innate immunity, Clin. Vaccine Immunol., 2006, 13, 219–226 CrossRef CAS PubMed.
- B. C. Sydora, B. D. Jamieson, R. Ahmed and M. Kronenberg, Intestinal intraepithelial lymphocytes respond to systemic lymphocytic choriomeningitis virus infection, Cell. Immunol., 1996, 167, 161–169 CrossRef CAS PubMed.
- M. Kaneko, T. Mizunuma, H. Takimoto and Y. Kumazawa, Development of TCR alphabeta CD8 alphaalpha intestinal intraepithelial lymphocytes is promoted by interleukin-15-producing epithelial cells constitutively stimulated by Gram-negative bacteria via TLR4, Biol. Pharm. Bull., 2004, 27, 883–889 CAS.
- D. Bruce and M. T. Cantorna, Intrinsic requirement for the vitamin D receptor in the development of CD8alphaalpha-expressing T cells, J. Immunol., 2011, 186, 2819–2825 CrossRef CAS PubMed.
- S. Ding, M. M. Chi, B. P. Scull, R. Rigby, N. M. Schwerbrock, S. Magness, C. Jobin and P. K. Lund, High-fat diet: bacteria interactions promote intestinal inflammation which precedes and correlates with obesity and insulin resistance in mouse, PLoS One, 2010, 5, e12191 Search PubMed.
- A. Davies, S. Lopez-Briones, H. Ong, C. O'Neil-Marshall, F. A. Lemonnier, K. Nagaraju, E. S. Metcalf and M. J. Soloski, Infection-induced expansion of a MHC Class Ib-dependent intestinal intraepithelial gammadelta T cell subset, J. Immunol., 2004, 172, 6828–6837 CrossRef CAS.
- A. Soares, E. J. Beraldi, P. E. Ferreira, R. B. Bazotte and N. C. Buttow, Intestinal and neuronal myenteric adaptations in the small intestine induced by a high-fat diet in mice, BMC Gastroenterol., 2015, 15, 3 CrossRef PubMed.
- J. E. Dalton, S. M. Cruickshank, C. E. Egan, R. Mears, D. J. Newton, E. M. Andrew, B. Lawrence, G. Howell, K. J. Else, M. J. Gubbels, B. Striepen, J. E. Smith, S. J. White and S. R. Carding, Intraepithelial gammadelta+ lymphocytes maintain the integrity of intestinal epithelial tight junctions in response to infection, Gastroenterology, 2006, 131, 818–829 CrossRef CAS PubMed.
- Z. Li, C. Zhang, Z. Zhou, J. Zhang, J. Zhang and Z. Tian, Small intestinal intraepithelial lymphocytes expressing CD8 and T cell receptor gammadelta are involved in bacterial clearance during Salmonella enterica serovar Typhimurium infection, Infect. Immun., 2012, 80, 565–574 CrossRef CAS PubMed.
- K. Inagaki-Ohara, Y. Sakamoto, T. Dohi and A. L. Smith, gammadelta T cells play a protective role during infection with Nippostrongylus brasiliensis by promoting goblet cell function in the small intestine, Immunology, 2011, 134, 448–458 CrossRef CAS PubMed.
- A. S. Ismail, C. L. Behrendt and L. V. Hooper, Reciprocal interactions between commensal bacteria and gamma delta intraepithelial lymphocytes during mucosal injury, J. Immunol., 2009, 182, 3047–3054 CrossRef CAS PubMed.
- A. S. Ismail, K. M. Severson, S. Vaishnava, C. L. Behrendt, X. Yu, J. L. Benjamin, K. A. Ruhn, B. Hou, A. L. DeFranco, F. Yarovinsky and L. V. Hooper, Gammadelta intraepithelial lymphocytes are essential mediators of host-microbial homeostasis at the intestinal mucosal surface, Proc. Natl. Acad. Sci. U. S. A., 2011, 108, 8743–8748 CrossRef CAS PubMed.
- P. R. de Oliveira, C. A. da Costa, G. F. de Bem, L. C. de Cavalho, M. A. de Souza, M. de Lemos Neto, P. J. da Cunha Sousa, R. S. de Moura and A. C. Resende, Effects of an extract obtained from fruits of Euterpe oleracea Mart. in the components of metabolic syndrome induced in C57BL/6J mice fed a high-fat diet, J. Cardiovasc. Pharmacol., 2010, 56, 619–626 CrossRef PubMed.
- L. McAllan, P. Skuse, P. D. Cotter, P. O'Connor, J. F. Cryan, R. P. Ross, G. Fitzgerald, H. M. Roche and K. N. Nilaweera, Protein quality and the protein to carbohydrate ratio within a high fat diet influences energy balance and the gut microbiota in C57BL/6J mice, PLoS One, 2014, 9, e88904 Search PubMed.
- M. E. de Sousa Rodrigues, M. Bekhbat, M. C. Houser, J. Chang, D. I. Walker, D. P. Jones, C. M. Oller do Nascimento, C. J. Barnum and M. G. Tansey, Chronic psychological stress and high-fat high-fructose diet disrupt metabolic and inflammatory gene networks in the brain, liver, and gut and promote behavioral deficits in mice, Brain, Behav., Immun., 2017, 59, 158–172 CrossRef CAS PubMed.
- D. B. West, C. N. Boozer, D. L. Moody and R. L. Atkinson, Dietary obesity in nine inbred mouse strains, Am. J. Physiol., 1992, 262, R1025–R1032 CAS.
- B. Ojo, G. D. El-Rassi, M. E. Payton, P. Perkins-Veazie, S. Clarke, B. J. Smith and E. A. Lucas, Mango Supplementation Modulates Gut Microbial Dysbiosis and Short-Chain Fatty Acid Production Independent of Body Weight Reduction in C57BL/6 Mice Fed a High-Fat Diet, J. Nutr., 2016, 146, 1483–1491 CrossRef CAS PubMed.
- J. B. Holm, A. Ronnevik, H. S. Tastesen, E. Fjaere, K. R. Fauske, U. Liisberg, L. Madsen, K. Kristiansen and B. Liaset, Diet-induced obesity, energy metabolism and gut microbiota in C57BL/6J mice fed Western diets based on lean seafood or lean meat mixtures, J. Nutr. Biochem., 2016, 31, 127–136 CrossRef CAS PubMed.
- P. S. Daltro, P. S. Alves, M. F. Castro, C. M. Azevedo, J. F. Vasconcelos, K. J. Allahdadi, L. A. de Freitas, B. S. de Freitas Souza, R. R. Dos Santos, M. B. Soares and S. G. Macambira, Administration of granulocyte-colony stimulating factor accompanied with a balanced diet improves cardiac function alterations induced by high fat diet in mice, BMC Cardiovasc. Disord., 2015, 15, 162 CrossRef PubMed.
- L. W. Engstrom, L. Bober, S. C. Chen, J. S. Fine, Y. Li, M. C. Stanton, D. Kinsley, L. Cui, J. V. Jackson, A. Rojas-Triana, D. Lundell, M. Laverty, E. L. Gustafson, C. H. Jenh, T. J. Kowalski and D. J. Manfra, Kinetic assessment and therapeutic modulation of metabolic and inflammatory profiles in mice on a high-fat and cholesterol diet, PPAR Res., 2010, 2010, 970164 CrossRef PubMed.
- T. H. Ehrich, J. P. Kenney, T. T. Vaughn, L. S. Pletscher and J. M. Cheverud, Diet, obesity, and hyperglycemia in LG/J and SM/J mice, Obes. Res., 2003, 11, 1400–1410 CrossRef CAS PubMed.
- H. Luck, S. Tsai, J. Chung, X. Clemente-Casares, M. Ghazarian, X. S. Revelo, H. Lei, C. T. Luk, S. Y. Shi, A. Surendra, J. K. Copeland, J. Ahn, D. Prescott, B. A. Rasmussen, M. H. Chng, E. G. Engleman, S. E. Girardin, T. K. Lam, K. Croitoru, S. Dunn, D. J. Philpott, D. S. Guttman, M. Woo, S. Winer and D. A. Winer, Regulation of obesity-related insulin resistance with gut anti-inflammatory agents, Cell Metab., 2015, 21, 527–542 CrossRef CAS PubMed.
- S. Nagafuchi, M. Totsuka, S. Hachimura, M. Goto, T. Takahashi, T. Yajima, T. Kuwata and S. Kaminogawa, Dietary nucleotides increase the proportion of a TCR gammadelta+ subset of intraepithelial lymphocytes (IEL) and IL-7 production by intestinal epithelial cells (IEC); implications for modification of cellular and molecular cross-talk between IEL and IEC by dietary nucleotides, Biosci., Biotechnol., Biochem., 2000, 64, 1459–1465 CrossRef CAS PubMed.
- M. Ogata, Y. Ota, M. Nanno, R. Suzuki and T. Itoh, Autocrine DNA fragmentation of intra-epithelial lymphocytes (IELs) in mouse small intestine, Cell Tissue Res., 2015, 361, 799–810 CrossRef CAS PubMed.
- S. Muller, M. Buhler-Jungo and C. Mueller, Intestinal intraepithelial lymphocytes exert potent protective cytotoxic activity during an acute virus infection, J. Immunol., 2000, 164, 1986–1994 CrossRef CAS.
- Z. Liu, R. S. Brooks, E. D. Ciappio, S. J. Kim, J. W. Crott, G. Bennett, A. S. Greenberg and J. B. Mason, Diet-induced obesity elevates colonic TNF-alpha in mice and is accompanied by an activation of Wnt signaling: a mechanism for obesity-associated colorectal cancer, J. Nutr. Biochem., 2012, 23, 1207–1213 CrossRef CAS PubMed.
- X. Luo, S. Luo, Y. Zheng, R. Wen, X. Deng and L. Zhou, Intestinal dysbacteriosis promotes intestinal intraepithelial T lymphocyte activation and proinflammatory cytokine secretion in mice, Xibao Yu Fenzi Mianyixue Zazhi, 2016, 32, 1031–1035 Search PubMed.
- S. Yamamoto, F. Russ, H. C. Teixeira, P. Conradt and S. H. Kaufmann, Listeria monocytogenes-induced gamma interferon secretion by intestinal intraepithelial gamma/delta T lymphocytes, Infect. Immun., 1993, 61, 2154–2161 CAS.
- M. Swamy, L. Abeler-Dorner, J. Chettle, T. Mahlakoiv, D. Goubau, P. Chakravarty, G. Ramsay, C. Reis e Sousa, P. Staeheli, B. A. Blacklaws, J. L. Heeney and A. C. Hayday, Intestinal intraepithelial lymphocyte activation promotes innate antiviral resistance, Nat. Commun., 2015, 6, 7090 CrossRef CAS PubMed.
- A. M. Fahrer, Y. Konigshofer, E. M. Kerr, G. Ghandour, D. H. Mack, M. M. Davis and Y. H. Chien, Attributes of gammadelta intraepithelial lymphocytes as suggested by their transcriptional profile, Proc. Natl. Acad. Sci. U. S. A., 2001, 98, 10261–10266 CrossRef CAS PubMed.
|
This journal is © The Royal Society of Chemistry 2017 |
Click here to see how this site uses Cookies. View our privacy policy here.