DOI:
10.1039/C6QM00284F
(Research Article)
Mater. Chem. Front., 2017,
1, 928-932
Luminescence properties of Na2SiF6:Mn4+ red phosphors for high colour-rendering white LED applications synthesized via a simple exothermic reduction reaction
Received
2nd November 2016
, Accepted 16th November 2016
First published on 9th December 2016
Abstract
Na2SiF6:Mn4+ red phosphor is reported in this paper. Na2SiF6:Mn4+ was synthesized via a simple exothermic reduction reaction approach using Na2SiF6 and NaMnO4 as raw materials. H2O2 was dropped into 0.02 mol L−1 of NaMnO4 in HF solution to reduce Mn7+ to Mn4+ at room temperature. The phosphors can produce sharp red emissions in a broad absorption range (from 500 nm to 700 nm). The white LEDs were fabricated with blue GaN chips merged with a mixture of Na2SiF6:Mn4+ and commercial YAG:Ce yellow phosphors for white light.
Introduction
Solid-state white light-emitting diodes (LEDs) are new generation lighting sources to replace traditional fluorescent lamps and incandescent light because of their various advantages such as low power consumption, long service life, fast response, high efficiency, small size, safety, and so on.1–13 At present, the most mature method for the preparation of white LEDs is a blue chip combined with a Y3Al5O12:Ce3+ (YAG:Ce3+) yellow phosphor. However, due to the lack of a red light component in the emission spectrum of YAG:Ce3+, it is hard to obtain white LEDs with a high color-rendering index (CRI, Ra > 80) and low color temperature (CC < 4000 K) for application in indoor lighting.14–16 During this year, great efforts have been made to explore novel rare-earth doped red phosphors to solve these problems. Of these, Eu2+-doped alkaline silicates, typically including Sr2SiO4:Eu2+ and Sr3SiO5:Eu2+, show a very broad excitation band and an effective absorption covering the blue spectra in the range of 450–470 nm.17 But these phosphors still have several drawbacks, such as hygroscopicity and bad thermostability.18 Now, Eu2+-doped (oxy)nitrite compounds, for example LiSr4B3O(9−3x/2)Nx:Eu2+, Sr2Si5N8:Eu2+, CaAlSiN3:Eu2+, are the most successful red phosphors for white LEDs.19–21 These phosphors were researched and have wide applications, but they are difficult to prepare under the rigorous synthesis conditions required for these compounds. To avoid the above drawbacks, an ideal red phosphor should possess relatively narrow absorption and emission bands, low-production costs and easy synthesis.
Recently, narrow-band red-emitting fluoride phosphors doped with Mn4+ have drawn increasing attention due to their good thermal stability and potential applications in white LED devices. Mn4+ is a transition metal ion with an outer 3d3 electron configuration. A spectral feature of Mn4+ is that it exhibits both broadband excitation and a sharp emission band due to its distinct electronic structure. Its optical properties are strongly influenced by local crystal field environments. Zhu et al. synthesized the K2TiF6:Mn4+ red phosphor, which exhibited the most intense excitation band at ∼468 nm with a bandwidth of ∼50 nm.22 Particularly, they achieved a photoluminescence quantum yield as high as 98% for K2TiF6:Mn4+, which is perfect for blue-chip excitation. Many researchers prepared all sorts of red fluoride phosphors, such as K2SiF6:Mn4+, Na2SiF6:Mn4+, K2GeF6:Mn4+, BaSiF6:Mn4+, ZnSiF6:Mn4+ and so on, which are synthesized via wet chemical etching of silicon wafers in aqueous HF solution with the addition of oxidizing agents NaMnO4 or KMnO4 at room temperature.23–28 The red phosphors have broadband excitation and a highly intense sharp emission band, which offset the lack of a red light component for white LEDs.
In this report, a simple reduction reaction was presented to synthesize red phosphor Na2SiF6:Mn4+ with sharp emission bands. The phosphors could be used for commercial applications, and exhibited intense emission, a short reaction time, and simple post-processing, as well as controllability of reactant size and other advantages. The crystal structure of the host, concentrations of HF and NaMnO4, and photoluminescence properties are discussed. The prepared fluoride red phosphors are potential candidates, and present a substantial advance for white LED applications.
Experimental
Synthesis
Red phosphor Na2SiF6:Mn4+ was synthesized using a directly mixed method as shown in Fig. 1. The raw materials consisted of a 10 ml HF (wt. 40%) solution, a Na2SiF6 (A. R.), H2O2 (wt. 35%) solution, and NaMnO4 (A. R.) powders. The NaMnO4 and HF (wt. 40%) solutions were mixed thoroughly by magnetically stirring in a Teflon-cup. The HF solution thoroughly dissolves NaMnO4 powders to obtain solution A at room temperature, and then solution B was formed by adding Na2SiF6 to the resulting solution A under continuous stirring for 20 min, meanwhile, this releases a lot of gas and gives off heat in solution B. Thereafter, 2 ml H2O2 was added to the deep purple solution, which rapidly turned milky orange. After the reaction, the resulting products were filtered, washed with ethanol, and dried at 70 °C to room temperature. The sample shows that it emits brilliant red light under blue light (365 nm) excitation (Fig. 1a), and shows a pale pink tint under natural light illumination (Fig. 1b).
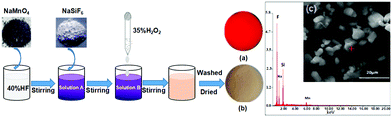 |
| Fig. 1 Synthesis of the Na2SiF6:Mn4+ phosphor, and samples of Na2SiF6:Mn4+: (a) UV light (λex = 365 nm), (b) room light, and (c) EDX spectrum and SEM image. | |
Characterization
The crystalline phases of the samples were analyzed using an X-ray diffractometer (XRD, TD-3500, Dandong, China) with Cu Kα radiation in the range of 2θ = 10°–70° with a step angle of 0.06°, a tube voltage of 30 kV and a tube current of 20 mA. The photoluminescent (PL) and PL excitation (PLE) spectra were measured by using a fluorescence spectrophotometer (F-7000, Hitachi, Japan) with a Xe lamp as the excitation light source. The excitation and emission spectra were scanned in the range of 330–500 nm and 550–700 nm, respectively. The surface morphology was observed using a scanning electron microscope (SEM, Quanta 250, FEI, USA).
The Mn4+ doping percentage in Na2SiF6 was estimated from the EDX results and the SEM image of the sample before and after the reaction indicates that the particle size is primarily in the range of 5–10 μm (Fig. 1c). All energy peaks correspond to the elements in Na2SiF6:Mn4+, except for the peaks at 0.25 and 0.5 keV, which correspond to organic matter from the preparation process. ICP-OES analyses show that the Mn4+ concentrations of the samples are 0.5–4.0 at%, which are increased by increasing the initial NaMnO4 concentration.
Results and discussion
Composition and structure
Fig. 2 shows the XRD patterns of the red phosphors of the Na2SiF6:Mn4+ samples. It can be seen that all these compounds correspond to Na2SiF6 (JCPDS No. 33-1280) with a hexagonal structure (space group – P321, the lattice parameter values are a = 0.8859 nm and c = 0.5038 nm). There are no other special obvious diffraction peaks. Therefore, the doped Mn4+ ion does not change the main phase of the crystal structure.
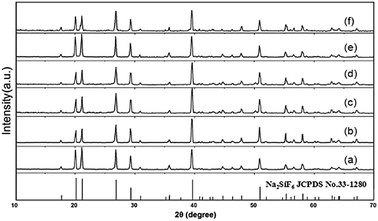 |
| Fig. 2 XRD patterns of the Na2SiF6:Mn4+ doped with various Mn4+ contents: (a) 0.005, (b) 0.01, (c) 0.015, (d) 0.02, (e) 0.03 and (f) 0.04 M. | |
The crystal structure and cation polyhedra arrangements of Na2SiF6 are shown in Fig. 3a. The crystal structure of the Na2SiF6 unit cell demonstrates a hexagonal structure. The center atom Si4+ is consistent of the type of octahedron, which is coordinated by six fluorine atoms with a Si–F distance as marked in Fig. 3b. Each Si atom is surrounded by six fluorine atoms resulting in only one kind of SiF62− octahedral structure. Therefore, when doped with Mn4+ atoms, the Si4+ atoms of the octahedron were replaced by Mn4+ atoms to obtain the MnF62− octahedral structure in Fig. 3c. The crystal field strength of the octahedral structure is changed with the ingress of Mn4+, because the radius of Mn4+ (0.053 nm) is larger than Si4+ (0.04 nm), which leads to the lattice expansion in the MnF62− octahedra, and neighboring lattice contraction. These effects cause unsymmetrical vibrations of the crystal field of the octahedral structure, and the electronic transition of 4A2g → 4T1g and 4A2g → 4T2g, and finally results in red light emission.
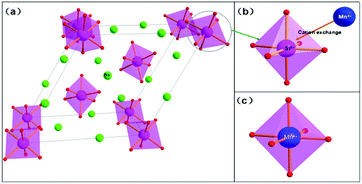 |
| Fig. 3 Crystal structure and cation polyhedron arrangements of the Na2SiF6:Mn4+ phosphor host. | |
Photoluminescence properties
The PLE and PL spectra of the Na2SiF6:Mn4+ (nMn = 0.02 mol l−1) red phosphors are shown in Fig. 4. The left curve corresponds to the PL excitation spectrum recorded in the range of 330 to 500 nm with monitoring at 631 nm emission. The PL excitation spectrum exhibits two broad bands with a weak peak at 360 nm and a strong peak at 460 nm, which are ascribed to the spin-allowed transitions of 4A2g → 4T1g and 4A2g → 4T2g respectively. Under 460 nm excitation, the right curve shows the sharp red emission of Na2SiF6:Mn4+ from 500 to 700 nm originating from the spin-forbidden 2Eg/4A2g transitions.22,29 There are seven peaks at ∼596, 607, 611, 620, 629, 632, and 645 nm, which are related to the transitions of the ν3(t1u), ν4(t1u), ν6(t2u), zero phonon line (ZPL), ν6(t2u), ν4(t1u), and ν3(t1u) vibronic modes, respectively.23–28
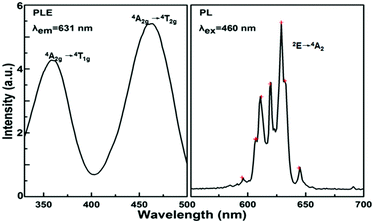 |
| Fig. 4 Excitation and emission spectra, where the excitation spectrum is monitored at λem = 631 nm and the emission spectra excited by 460 nm. | |
Fig. 5 shows the PLE and PL spectra of the Na2SiF6:Mn4+ phosphors synthesized with different concentrations of NaMnO4. The inset shows the emission intensity, measured at λem = 631 nm with excitation at λex = 460 nm, versus NaMnO4 concentration. The concentration of NaMnO4 has a pronounced effect on the luminescence intensities of Na2SiF6:Mn4+. We observed that the luminescence intensities of Na2SiF6:Mn4+ increased gradually when the NaMnO4 concentration increased from 0.005 to 0.02 mol l−1. The highest luminescence intensity was found with the optimized NaMnO4 concentration of 0.02 mol l−1.
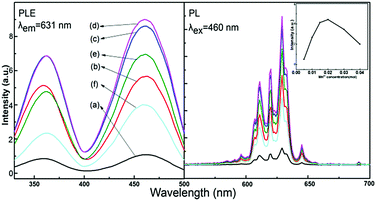 |
| Fig. 5 Excitation and emission spectra with a NaMnO4 concentration of (a) 0.005, (b) 0.01, (c) 0.015, (d) 0.02, (e) 0.03, and (f) 0.04 mol l−1; the inset curve shows the maximum PL intensities. | |
The effects of HF concentration on the luminescence of Na2SiF6:Mn4+ are shown in Fig. 6. With the increase in HF concentration, the excitation and emission intensities of Na2SiF6:Mn4+ increased gradually from 10 to 40 wt%. Hence, we can determine the optimized luminescence intensity by the solution with HF concentration at 40 wt%. According to the luminous theory, the results indicated that high HF concentrations might make Mn4+ easy to introduce into the host.
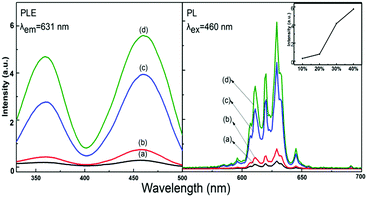 |
| Fig. 6 Excitation and emission spectra of the Na2SiF6:Mn4+ phosphor with HF concentrations of (a) 10, (b) 20, (c) 30, and (d) 40 wt%. | |
The colorimetric coordinates (x, y) for the Na2SiF6:Mn4+ phosphor series are calculated using an equidistant wavelength method and are summarized in Table 1. They are depicted in the CIE chromaticity diagram shown in Fig. 7. The colorimetric coordinate of the sample with (nMn4+) = 0.005 mol l−1 locates in the orange-red region with the value being (0.6364, 0.3566). The colorimetric coordinate shifts to the crimson region and the value fluctuates in a small area for different Mn doping amounts (0.01–0.04 mol l−1). However, the position of the colorimetric coordinates depends on the form of the emission spectrum above.
Table 1 Wavelength and colorimetric coordinates (x, y) with different Mn4+ concentrations in the Na2SiF6:Mn4+ phosphor series
n (Mn4+) |
Peaks |
CIE coordinates (x, y) |
x
|
y
|
0.005 |
629 |
0.6364 |
0.3566 |
0.010 |
630 |
0.6708 |
0.3274 |
0.015 |
630 |
0.6769 |
0.3222 |
0.020 |
631 |
0.6770 |
0.3219 |
0.030 |
631 |
0.6762 |
0.3224 |
0.040 |
631 |
0.6709 |
0.3268 |
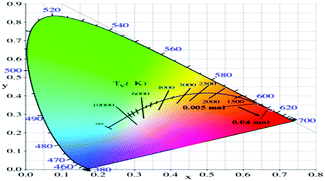 |
| Fig. 7 Coordinate location points of Na2SiF6:Mn4+ (0.005 to 0.04 mol l−1) in the CIE chromaticity diagram. | |
Fabrication of white LEDs using InGaN-based blue LEDs with Na2SiF6:Mn4+ red phosphors
The electroluminescence spectra of the white LEDs under different drive currents are shown in Fig. 8a. We have fabricated white LEDs using blue InGaN chips, commercial YAG:Ce3+ yellow phosphors and Na2SiF6:Mn4+ red phosphors as shown in Fig. 8b, and one white LED was illuminated under 120 mA drive current as shown in Fig. 8c, with the luminous efficacy of 101.5 lm W−1 and CRI of 86.5. Table 2 shows different relevant photoelectric parameters, such as the CCT, CRI and chromaticity coordinate for the fabricated white LEDs. The results indicate that the Na2SiF6:Mn4+ red phosphors are potential candidates current mature white LED applications.
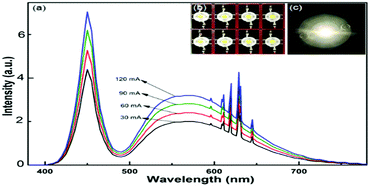 |
| Fig. 8 Luminescence spectra based on the Na2SiF6:Mn4+ phosphor under various drive currents (a); white LEDs (b) and bright white-light (c). | |
Table 2 Photoelectric parameters of typical white LEDs fabricated with different phosphors
White LEDs |
Phosphor composition |
Y3Al5O12:Ce3+ |
Y3Al5O12:Ce3+ and Na2SiF6:Mn4+ |
CCT (K) |
5290 |
4986 |
R
a
|
75.8 |
86.5 |
R
9
|
8.8 |
79.1 |
Chromaticity coordinate |
x = 0.342; y = 0.326 |
x = 0.351; y = 0.321 |
Current (mA) |
120 |
120 |
Efficacy (lm W−1) |
106.9 |
101.5 |
Conclusions
In summary, Na2SiF6:Mn4+ red fluoride phosphors were synthesized by using a simple exothermic reduction reaction method. Na2SiF6:Mn4+ had a micrometer-sized hexagonal structure, and the particle size can be controlled from the raw materials used. The prepared phosphors had strong blue-light absorption and intense narrow-band red emission. The optimal concentration of NaMnO4 in the reaction system for the synthesis of Na2SiF6:Mn4+ is 0.02 mol l−1, which is much lower than those in the previously reported studies, and the spectra of the Na2SiF6:Mn4+ phosphor were affected with disparate HF concentrations. A significant improvement in the CRI of the white LEDs fabricated using the Na2SiF6:Mn4+ red phosphor was observed. The obtained Na2SiF6:Mn4+ phosphors, with efficient red luminescence under blue light excitation, a short reaction time, high yield, and simple post-processing, are a potential candidate for improving the color reproducibility of white LEDs.
This work was financially supported by the National Natural Science Foundation of China (Grant No. 51402032), the China Postdoctoral Science Foundation funded project (Grant No. 2014M562297), the Chongqing Youth Science and Technology talent cultivation project (Grant No. cstc2014kjrc-qnrc40006) and the Chongqing Education Commission funded project (Grant No. KJ1401102).
Notes and references
- S. Nakamura, T. Mukai and M. Senoh, Appl. Phys. Lett., 1994, 64, 1687–1689 CrossRef CAS.
- C. J. Humphreys, MRS Bull., 2008, 33, 459–470 CrossRef.
- E. F. Schubert and J. K. Kim, Science, 2005, 308, 1274–1278 CrossRef CAS PubMed.
- Q. Su, H. Wu, Y. X. Pan, J. Xu, C. F. Guo, X. M. Zhang, J. H. Zhang, J. Wang and M. J. Zhang, J. Rare Earths, 2005, 23, 513–517 CAS.
- H. Daicho, T. Iwasaki, K. Enomoto, Y. Sasaki, Y. Maeno, Y. Shinomiya, S. Aoyagi, E. Nishibori, M. Sakata, H. Sawa, S. Matsuishi and H. Hosono, Nat. Commun., 2012, 3, 1132 CrossRef PubMed.
- C. Y. Sun, X. L. Wang, X. Zhang, C. Qin, P. Li, Z. M. Su, D. X. Zhu, G. G. Shan, K. Z. Shao, H. Wu and J. Li, Nat. Commun., 2013, 4, 2717 Search PubMed.
- W. B. Im, N. George, J. Kurzman, J. Kurzman, S. Brinkley, A. Mikhailovsky, J. Hu, B. F. Chmelka, S. P. DenBaars and R. Seshadri, Adv. Mater., 2011, 23, 2300–2305 CrossRef CAS PubMed.
- W. T. Chen, H. S. Sheu, R. S. Liu and J. P. Attfield, J. Am. Chem. Soc., 2012, 134, 8022–8025 CrossRef CAS PubMed.
- Q. Dai, M. E. Foley, C. J. Breshike, A. Lita and G. F. Strouse, J. Am. Chem. Soc., 2011, 133, 15475–15486 CrossRef CAS PubMed.
- C. J. Duan, W. M. Otten, A. C. A. Delsing and H. T. Hintzen, J. Alloys Compd., 2008, 461, 454–458 CrossRef CAS.
- W. B. Park, S. P. Singh, C. Yoon and K. S. Sohn, J. Mater. Chem. C, 2013, 1, 1832–1839 RSC.
- W. B. Park, S. P. Singh and K. S. Sohn, J. Am. Chem. Soc., 2014, 136, 2363–2373 CrossRef CAS PubMed.
- C. W. Yeh, W. T. Chen, R. S. Liu, S. F. Hu, H. S. Sheu, J. M. Chen and H. T. Hintzen, J. Am. Chem. Soc., 2012, 134, 14108–14117 CrossRef CAS PubMed.
- P. Pust, V. Weiler, C. Hecht, A. Tucks, A. S. Wochnik, A. K. Hen, D. Wiechert, C. Scheu, P. J. Schmidt and W. Schnick, Nat. Mater., 2014, 13, 891–896 CrossRef CAS PubMed.
- M. R. Krames, O. B. Shchekin, R. Mueller-Mach, G. O. Mueller, L. Zhou, G. Harbers and M. G. Craford, J. Disp. Technol., 2007, 3, 160–175 CrossRef CAS.
- P. F. Smet, A. B. Parmentier and D. Poelman, J. Electrochem. Soc., 2011, 158, R37–R54 CrossRef CAS.
- H. S. Jang and D. Y. Jeon, Appl. Phys. Lett., 2007, 90, 041906 CrossRef.
- P. L. Li, Y. Wang and Q. L. Guo, Sci. China Press, 2011, 56, 488–490 Search PubMed.
- R. J Xie, N. Hirosaki, Y. Li and T. Takeda, Materials, 2010, 3, 3777–3793 CrossRef.
- T. Suehiro, R. J. Xie and N. Hirosaki, Ind. Eng. Chem. Res., 2013, 52, 7453–7456 CrossRef CAS.
- H. Yu, D. Deng, S. Xu, C. P. Yu, H. Y. Yin and Q. L. Nie, J. Lumin., 2012, 132, 2553–2556 CrossRef CAS.
- H. M. Zhu, C. C. Lin, W. Q. Luo, S. T. Shu, Z. G. Liu, Y. S. Liu, J. T. Kong, E. Ma, Y. G. Cao, R. S. Liu and X. Y. Chen, Nat. Commun., 2014, 8, 1–10 CrossRef.
- T. Takahashi and S. Adachi, J. Electrochem. Soc., 2008, 155, E183–E188 CrossRef CAS.
- S. Adachi, H. Abe, R. Kasa and T. Arai, J. Electrochem. Soc., 2012, 159, J34–J37 CrossRef CAS.
- R. Kasa and S. Adachi, J. Appl. Phys., 2012, 112, 013506 CrossRef.
- Y. K. Xu and S. Adachi, J. Electrochem. Soc., 2011, 158, J58–J65 CrossRef CAS.
- D. Sekiguchi, J. Nara and S. Adachi, J. Appl. Phys., 2013, 113, 183516 CrossRef.
- R. Hoshino and S. Adachi, J. Appl. Phys., 2013, 114, 213502 CrossRef.
- H. D. Nguyen, C. C. Lin, M. H. Fang and R. S. Liu, J. Mater. Chem. C, 2014, 2, 10268–10272 RSC.
|
This journal is © the Partner Organisations 2017 |
Click here to see how this site uses Cookies. View our privacy policy here.