DOI:
10.1039/C6QM00014B
(Research Article)
Mater. Chem. Front., 2017,
1, 91-99
Blue pyrene-based AIEgens: inhibited intermolecular π–π stacking through the introduction of substituents with controllable intramolecular conjugation, and high external quantum efficiencies up to 3.46% in non-doped OLEDs†
Received
13th April 2016
, Accepted 16th May 2016
First published on 29th July 2016
Abstract
Six blue AIE luminogens, Py-2pTPE, Py-2mTPE, Py-2TP, Py-2TF, Py-2NTF and Py-2F, have been successfully synthesized, which exhibit sky blue (484 nm) to deep blue (444 nm) emissions in accordance with the different introduced aromatic substituents on the pyrene core and the different linkage modes. Furthermore, Py-2pTPE and Py-2mTPE show interesting mechanochromism effects, inherited from TPE-pBr and TPE-mBr, respectively. When fabricated as emitters in OLEDs, all of the six AIEgens demonstrate blue emissions and good EL performances, among which, Py-2TP shows the best device performance with an ηEQE,max up to 3.46% at a CIE coordinate of (0.15, 0.09).
1 Introduction
Organic light-emitting diodes (OLEDs) have attracted great interest due to their promise as devices for full-color display applications.1 However, so far, unlike their green and red congeners, efficient and stable blue materials, especially the deep blue ones with Commission Internationale de l'Éclairage (CIE) coordinates (x ≤ 0.15, y < 0.1) are still scarce as a result of their intrinsic wide bandgap.2 In order to meet the demands of full-color displays, great efforts have been made to develop efficient deep blue luminogens (Chart S1, ESI†),3 among which, pyrene and its derivatives have received the spotlight in the OLED field on account of their deep blue emissions, high quantum efficiencies, carrier mobility, and thermal and chemical stabilities.4 However, their tendency to form excimers via π–π stacking in the aggregation state constitutes severe impediments such as large red-shifted emission and aggregation-caused quenching (ACQ) effect. In order to suppress the unwanted π–π stacking of pyrene and realize good performances for OLEDs, especially the deep blue emissions derived from pyrene itself, great efforts have been made (Chart S2, ESI†).5 For example, Liu et al. successfully suppressed the intermolecular π-stacking of pyrene by the introduction of 9,9-di(triarylamino)fluorenes to obtain DTDPF with ηC,max and ηEQE,max values of 1.31 cd A−1 and 1.83% at a CIE coordinate of (0.15, 0.16).5a Then, Lee et al. replaced the 9,9-di(triarylamino)fluorene moieties with 9,9-bis-(3-(9-phenyl-carbazoyl)) groups to give DCDPF, which suppressed the aggregation and achieved a much higher efficiency of 4.4 cd A−1 with a pure blue CIE coordinate of (0.15, 0.15).5c Chow et al. also reported a series of sterically congested tetraarylpyrenes exhibiting no π-stacking behavior, and among them, Py-4Ph3 gave the best performance of 2.7 cd A−1 (ηC,max) and 3.26% (ηEQE,max) at a CIE coordinate of (0.14, 0.09).5e However, there is still much room for further improvement of the OLED performance while retaining the deep blue emission with CIE coordinates of (x ≤ 0.15, y < 0.1).
On the other hand, from 2001, first reported by Ben Zhong Tang et al., the research on organic luminogens exhibiting an aggregation-induced emission (AIE) effect has become a hot topic.6 Zhao and Tang et al. have successfully created a highly efficient solid emitter, TTPEPy, by decorating the pyrene core with four AIE-active tetraphenylethene (TPE) peripheries, its ηC,max, and ηEQE,max could be up to 12.3 cd A−1 and 4.95%, respectively.5h However, regardless of the effectively suppressed π–π stacking, it is a pity that the EL emission peak of TTPEPy red-shifts to 488 nm, nearly out of the blue region owing to the much extended intramolecular conjugation upon the introduction of four TPE moieties. Thus, a balance should be achieved between the restricted π–π stacking and controlled conjugation, namely high efficiency and deep blue emission. In the past several years, our group has successfully developed a series of excellent blue AIE materials based on TPE by modifying the linkage mode, utilizing the crystallization-induced blue-shifted emission, making twisted conformation, and interrupting the conjugation.7 However, unfortunately, no deep blue AIEgens with CIE coordinates of (x ≤ 0.15, y < 0.1) were achieved by the intrinsic conjugation of TPE. Then, excitedly, after replacing TPE with pentaphenylbenzene moieties possessing a relatively weak conjugation effect, some deep blue AIEgens with the characteristic of aggregation enhanced emission (AEE) were achieved with ηC,max and ηEQE,max values of 2.0 cd A−1 and 2.3% at a CIE coordinate of (0.15, 0.08).8 Thus, this might be another effective way to develop deep blue AIEgens through introducing some other AIE/AEE building blocks with weak conjugation to a core.9 However, another question then arose, what kind of AIE building blocks would be better for realizing deep blue emission as well as retaining excellent EL performance? We might more likely select some AIE building blocks with weak intrinsic conjugation in order to guarantee the deep blue emission. Then, mainly two types of groups could be considered: one was that with a highly twisted conformation, while another was that with a short conjugation length.10 Generally, the much twisted configuration will impede the carrier mobility, possibly leading to inferior EL performance, while the relatively small aromatic ones with short conjugation lengths could not restrain the intermolecular π–π stacking effectively, particularly for the cores with a relatively planar conformation. Is it possible to find an appropriate AIE building block restraining the intermolecular π–π stacking exactly without sacrificing the carrier mobility to a large degree to realize the deep blue emission with good device performances? In order to answer this question, four AIEgens Py-2TP, Py-2TF, Py-2NTF and Py-2F (Fig. 1 and Fig. S1, ESI†) were designed, in which as a typical example, the pyrene group, a frequently used constructing block for blue luminogens as mentioned above, acted as the core, and different AIE building blocks were introduced as peripheral groups from the small m-terphenyl group to the larger 9,9′-diarylfluorenyl one. For comparison, two TPE-based AIEgens, Py-2pTPE and Py-2mTPE, were also prepared with different linkage modes, para and meta. All the six compounds exhibit apparent AIE characteristics and good performance in their corresponding LED devices with blue emissions. Excitedly, Py-2TP shows the best OLED performance with the maximum current efficiency and external quantum efficiency up to 2.94 cd A−1 and 3.46%, respectively at a CIE coordinate of (0.15, 0.09), disclosing that the introduced m-terphenyl groups with suitable bulk to the pyrene core in Py-2TP meet the above requirement as appropriate AIE building blocks perfectly. Herein, we present in detail the syntheses, characterizations, theoretical calculations, and light-emitting properties of Py-2pTPE, Py-2mTPE, Py-2TP, Py-2TF, Py-2NTF and Py-2F.
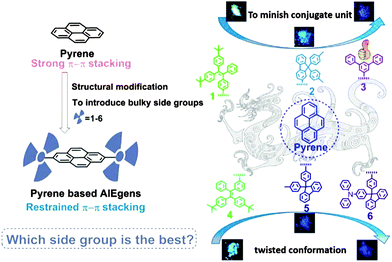 |
| Fig. 1 A diagram of pyrene restraining the π–π stacking through introducing bulky side groups and the corresponding photographs of the pyrene based AIEgens. | |
2 Results and discussion
2.1 Synthesis
The synthetic routes to Py-2pTPE, Py-2mTPE, Py-2TP, Py-2TF, Py-2NTF and Py-2F are illustrated in Scheme 1. The key intermediate, compound 1 was synthesized under the catalysis of [Ir(μ-OMe)cod]2. TPE-pBr and TPE-mBr were both obtained through the reaction of diphenylmethane derivatives and benzophenone derivatives. The other four intermediates, Ar–Br, were synthesized according to the literature.11 The target compounds, Py-2pTPE, Py-2mTPE, Py-2TP, Py-2TF, Py-2NTF and Py-2F, were conveniently synthesized through the Suzuki coupling reaction, and purified by column chromatography on silica gel following by recrystallization. They were all characterized by 1H, 13C NMR, mass spectrometry and elemental analysis.
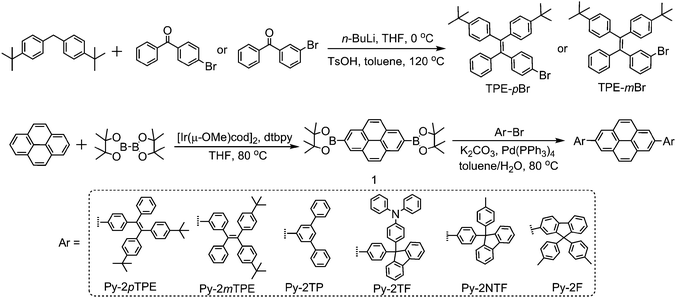 |
| Scheme 1 Synthetic routes for Py-2pTPE, Py-2mTPE, Py-2TP, Py-2TF, Py-2NTF and Py-2F. | |
2.2 Thermal properties
Thermogravimetric analysis (TGA) and differential scanning calorimetry (DSC) were used to measure the thermal properties of these AIEgens (Table 1). As shown in Fig. S2 (ESI†), they were all thermally stable, and their thermal decomposition temperatures (Td, corresponding to 5% weight loss) were all about 500 °C owing to the rigid pyrene and the bulky tetraphenylethene, 9,9′-diphenylfluorenyl and m-terphenyl groups. Their glass transition temperatures (Tg) were also very high, ranging from 148 to 191 °C (Fig. S3, ESI†). However, no obvious Tg value was observed in the DSC curve of Py-2NTF. Good thermal stabilities would contribute much to their stabilities and long lifetimes when used in OLED devices.
Table 1 The thermal, electrochemical and photophysical data of Py-2pTPE, Py-2mTPE, Py-2TP, Py-2TF, Py-2NTF and Py-2F
|
T
d
(°C) |
T
g (°C) |
E
g
(eV) |
E
HOMO
/LUMO
(eV) |
λ
abs
(nm) |
PL λmax(solution)e (nm) |
Φ
F(solution) (%) |
PL λmax(aggregation)f (nm) |
Φ
F(powder) (%) |
PL λmax(film) (nm) |
α
AIE
|
5% weight loss temperature measured by TGA under N2.
Band gap estimated from the optical absorption band edge of the solution.
Calculated from the onset oxidation potentials of the compounds.
Estimated using the empirical equation ELUMO = EHOMO + Eg.
Observed from absorption spectra in dilute THF solution.
Determined in THF : H2O = 1 : 99 solution.
α
AIE = ΦF(powder)/ΦF(solution).
|
Py-2pTPE |
498 |
146 |
3.15 |
−5.46/−2.31 |
342 |
— |
0.1 |
494 |
55.2 |
486 |
552.0 |
Py-2mTPE |
464 |
160 |
3.28 |
−5.48/−2.20 |
342 |
— |
0.1 |
477 |
21.5 |
468 |
215.0 |
Py-2TP |
500 |
191 |
3.53 |
−5.68/−2.15 |
342 |
416, 437 |
3.1 |
427, 448 |
15.9 |
427, 446 |
5.1 |
Py-2TF |
527 |
182 |
3.54 |
−5.22/−1.68 |
341 |
417, 438 |
1.5 |
424, 444 |
10.4 |
423, 441 |
6.9 |
Py-2NTF |
506 |
— |
3.55 |
−5.67/−2.12 |
341 |
417, 438 |
3.5 |
426, 446 |
26.9 |
425, 443 |
7.7 |
Py-2F |
517 |
186 |
3.37 |
−5.62/−2.25 |
341 |
424, 446 |
11.4 |
437, 451 |
18.3 |
437, 449 |
1.6 |
2.3 Optical properties
Fig. S4 (ESI†) shows the UV-vis spectra of Py-2pTPE, Py-2mTPE, Py-2TP, Py-2TF, Py-2NTF and Py-2F in THF solution. Py-2pTPE had the most red-shifted absorption spectrum owing to the good conjugation between para-tetraphenylethene and pyrene, while a blue-shift was observed for Py-2mTPE due to the meta-linkage mode with a much weaker conjugation effect as proved in our previous cases.12 This linkage mode effect not only existed in the para and meta-positions of tetraphenylethene, but also at the different reactive sites of 9,9′-diphenylfluorene. Just as shown in Fig. S4 (ESI†), Py-2NTF exhibited a blue-shifted absorption compared to Py-2F, owing to the weak conjugation of 9,9′-positions in fluorene as well as the much twisted conformation of this linkage mode. Then, triphenylamine groups were introduced at 9,9′-positions of fluorene and Py-2TF was yielded. There was nearly no difference between Py-2NTF and Py-2TF in the UV absorption spectra, due to the unconjugated 9,9′-positions of fluorene.
In order to investigate the AIE effect of these new compounds, we studied their fluorescence behaviours, by choosing THF as a good solvent and water as a poor one. As shown in Fig. 2 and Fig. S5 (ESI†), all of them exhibited AIE or AEE (aggregation-enhanced emission) effects. In detail, for Py-2pTPE and Py-2mTPE, which consisted of typical AIE units of tetraphenylethene, they showed typical AIE characteristics (nearly non-emissive in pure THF solution, however very strong after the addition of a large amount of water). Interestingly, for Py-2pTPE (Fig. 2A), as the water fraction ranged from 30% to 50%, it showed a deep blue emission which peaked at about 450 nm and the luminescence intensity increased gradually, and then reached the maximum at 50% water fraction. However, when the water fraction increased to 60%, it gave a very weak luminescence and its emission peak red-shifted to about 494 nm. This abnormal phenomenon might originate from the transition from the crystalline state to the amorphous one, as well-proven and explained in previous reported cases.13 The same phenomenon also appeared in Py-2mTPE, well certifying the universality of crystallization-induced blue-shifted emission and crystallization-induced enhanced emission in AIEgens.
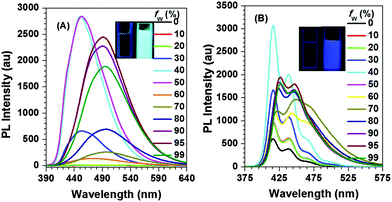 |
| Fig. 2 PL spectra in THF/H2O mixtures with different water fractions: (A) Py-2pTPE, concentration (µM): 12.0; excitation wavelength 330 nm; (B) Py-2TP, concentration (µM): 14.0; excitation wavelength 300 nm. Inset: Photos of Py-2pTPE and Py-2TP in THF/H2O mixtures (fw = 0 and 99%) taken under the illumination of a 365 nm UV lamp. | |
In pure THF solution, their quantum yields were just about 0.1% by using 9,10-diphenylanthracene as the standard (Fig. 3). When the water fraction increased to 99%, the yields were 29.2% and 24.7% for Py-2pTPE and Py-2mTPE, respectively, more than 200 times increased in comparison with those in pure THF solution (Table 1). However, as for the other four compounds, Py-2TP, Py-2TF, Py-2NTF and Py-2F, they only exhibited AEE properties (in pure THF solution, they could give weak emissions, and the emissions became stronger upon the addition of water). Their αAIE values (Φ(powder)/Φ(solution)) were calculated to be 5.1, 6.9, 7.7 and 1.6 for Py-2TP, Py-2TF, Py-2NTF and Py-2F, respectively. For Py-2pTPE, Py-2mTPE and Py-2TP bearing typical free rotators of phenyl rings, the AIE effects should mainly originate from the restriction of intramolecular rotation (RIR) effect. However, for Py-2TF, Py-2NTF and Py-2F without apparent free rotators, their AEE effects might be derived from the synergistic effect of restriction of intramolecular rotation (RIR) and restriction of intramolecular vibration (RIV).14
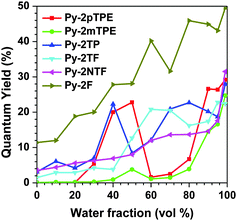 |
| Fig. 3 Plots of fluorescence quantum yields of Py-2pTPE, Py-2mTPE, Py-2TP, Py-2TF, Py-2NTF and Py-2F determined in THF/H2O solutions by using 9,10-diphenylanthracene (Φ = 90% in cyclohexane) as a standard versus water fractions. | |
We further investigated the fluorescence properties of the AIEgens in the solid state, since they are often fabricated as thin solid films in practical applications. As shown in Fig. 4 and Fig. S6 (ESI†), the emission peak of Py-2pTPE in the film located at 486 nm between the emission of the crystalline state (450 nm) and amorphous state (494 nm) but more close to amorphous state, indicating that it mainly existed in the amorphous state similar to Py-2mTPE. As for the other four compounds, Py-2TP, Py-2TF, Py-2NTF, and Py-2F, they just demonstrated slight red-shifts from solutions to films, ranging from 5 to 13 nm, totally different from pyrene itself which has a large red-shift of more than 70 nm from solutions to films. The ignorable red-shifts of these four AIEgens manifested that the introduction of m-terphenyl and 9,9′-diarylfluorenyl groups could suppress the π–π stacking effectively, almost realizing the feasibility of our design strategy.
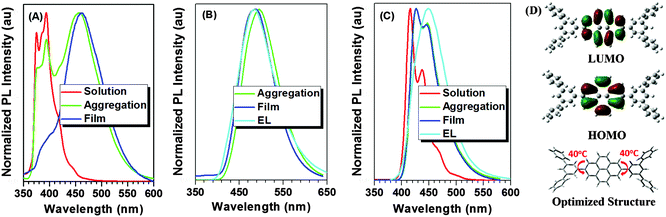 |
| Fig. 4 (A) The normalized solution, aggregation and film spectra for pyrene; (B) the normalized aggregation, film and EL spectra for Py-2pTPE; (C) the normalized solution, aggregation, film and EL spectra for Py-2TP; (D) calculated molecular orbital amplitude plots of LUMO and HOMO levels and the optimized molecular structure of Py-2TP. | |
2.4 Theoretical calculations
To further verify the structure–property relationship at the molecular level, density functional theory (DFT) calculations (B3LYP/6-31g*) were carried out to obtain the optimized structures and orbital distributions of HOMO and LUMO energy levels of Py-2pTPE, Py-2mTPE, Py-2TP, Py-2TF, Py-2NTF and Py-2F. As revealed in Fig. 4D and Fig. S7 (ESI†), for Py-2TP, Py-2NTF and Py-2F, their HOMOs and LUMOs were all located on the pyrene core, showing local electronic cloud distributions. As for Py-2pTPE, Py-2mTPE and Py-2TF, their HOMOs were mainly distributed on the tetraphenylethene or triphenylamine group due to their good hole transporting ability, while their LUMOs were also on the pyrene core. Further inspection of the optimized molecular structures was carried out. As disclosed from their optimized structures (Fig. 4D and Fig. S7, ESI†), all the torsion angles between the pyrene core and peripheral substituent groups were as large as about 40°, and the twisted conformations could suppress the π–π stacking effectively and contribute much to the AIE or AEE effect.
2.5 Mechanochromism properties
In TPE-pBr and TPE-mBr, tert-butyl groups were introduced to improve the solubility as the initial goal. Unexpectedly, the tert-butyl groups were found to endow TPE-pBr and TPE-mBr with the novel mechanochromism effect. As shown in Fig. 5A, the as-prepared TPE-pBr gave a deep blue emission at 424 nm. After being simply ground using a mortar and pestle, its emission red-shifted to sky blue at 463 nm, showing a high contrast as large as 39 nm. After the ground samples were fumed for a few minutes with THF solution, the emission color almost returned to its original appearance. The reversible switching by mechanical stimuli might be derived from the transition from the crystalline state to the amorphous one. The changes in solid state of TPE-pBr were further confirmed by X-ray diffraction (XRD) patterns (Fig. S8, ESI†). There were many sharp peaks for the as-prepared TPE-pBr, suggesting its existence mainly in the crystalline state. After being ground, it turned to the amorphous state and displayed low-intensity reflections along with some peaks missing. Then, the peaks of the reflections recovered after being fumed. A similar phenomenon was also observed in TPE-mBr (Fig. S9, ESI†). When pyrene was decorated with TPE-pBr and TPE-mBr to yield Py-2pTPE and Py-2mTPE, the mechanochromism effect was inherited (Fig. 5B and Fig. S10, ESI†). Among them, Py-2pTPE showed a better performance with a different emission peak as large as 33 nm. As shown in Fig. 5B, when the Py-2pTPE solid was ground, the PL emission red-shifted to green at about 499 nm, while it gave sky blue emission (466 nm) after being fumed showing a high color contrast.
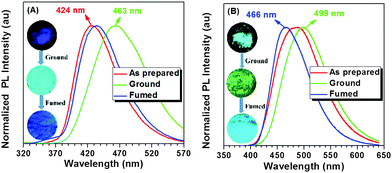 |
| Fig. 5 (A) Their emission spectra of the as-prepared, ground and fumed TPE-pBr solids, inset: their photographs taken under UV illumination (365 nm); (B) the emission spectra of the as-prepared, ground and fumed Py-2pTPE solids, inset: their photographs taken under UV illumination (365 nm). | |
2.6 Electrochemical properties
Cyclic voltammetry (CV) was carried out to investigate the electrochemical behavior of these six AIEgens (Fig. S11, ESI†). The HOMO energy levels were estimated from the onset oxidation potentials according to the equation EHOMO = −(4.8 − E(Fc/Fc+) + Eox) eV, in which E(Fc/Fc+) is 0.48 eV and the LUMO energy levels were obtained from the optical band gap energies (estimated from the onset wavelengths of the UV absorptions) and the HOMO values. The highest HOMO value of −5.22 eV was achieved in Py-2TF, which was slightly higher than that of NPB (−5.30 eV), suggesting that it might possess good hole-injection ability due to the introduction of triphenylamine groups. The HOMOs of Py-2pTPE and Py-2mTPE were −5.46 and −5.48 eV, respectively, which were also very close to that of NPB. This would be much beneficial for the hole-injection and high efficiencies when they were used in OLED devices. As for Py-2TP, Py-2NTF and Py-2F, they had the similar HOMO values, namely −5.68, −5.67, and −5.62 eV, respectively. The corresponding LUMO energy levels were calculated to be −2.31, −2.22, −2.15, −1.68, −2.12 and −2.25 eV for Py-2pTPE, Py-2mTPE, Py-2TP, Py-2TF, Py-2NTF, and Py-2F, respectively. The slight differences in their LUMO energy levels suggested that they should have a similar electron-injection ability.
2.7 Electroluminescence
Considering their good thermal stabilities and efficient light emissions in the solid state, we fabricated non-doped OLED devices using these AIEgens as emitters with a configuration of ITO (indium tin oxide)/PEDOT:PSS (polyethylenedioxythiophene–polystyrene sulfonic acid)/NPB (1,4-bis(1-naphthylphenylamino)biphenyl, 40 nm)/EML (emissive layer, 10–20 nm)/TPBI (1,3,5-tris(N-phenylbenzimidizol-2-yl)-benzene, 35 nm)/Ca:Ag, in which NPB and TPBI served as the hole-transporting and electron-transporting layers, respectively, PEDOT:PSS was used to smooth the ITO surface and decrease the hole injection barrier, and the six AIEgens served as emitters. Fig. 6 and Fig. S12 (ESI†) show the voltage–current density and voltage–brightness characteristics, current efficiency versus current density curves, external quantum efficiency versus current density curves and power efficiency versus current density curves.
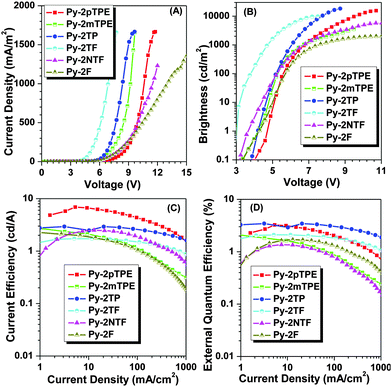 |
| Fig. 6 Changes in (A) current density with the applied voltage, (B) brightness with the applied voltage, (C) current efficiency with the current density, (D) external quantum efficiency with the current density. Device configuration: ITO/PEDOT:PSS/NPB (40 nm)/Py-2pTPE, Py-2mTPE, Py-2TP, Py-2TF, Py-2NTF and Py-2F (10–20 nm)/TPBI (35 nm)/Ca:Ag. | |
As listed in Table 2, Py-2TP exhibited the best performance with the maximum brightness, current efficiency and external quantum efficiency up to 18
287 cd m−2, 2.94 cd A−1 and 3.46% at a CIE coordinate of (0.15, 0.09), owing to the excellent carrier mobility of pyrene and short conjugation of m-terphenyl groups. Notably, it possessed very high stabilities of EL efficiencies and spectra. As listed in Table S1 (ESI†), the device based on Py-2TP reached its highest efficiency at 5.2 V with a practical brightness of about 100 cd m−2. Accompanied by the increase of the brightness to about 1000 cd m−2, the current and external quantum efficiency were 2.83 cd A−1 and 3.34%, respectively, with a roll-off value of below 4%. Then, when the brightness increased to about 5000 cd A−1, it retained a current efficiency of 2.3 cd A−1 with a roll-off value of 21.8%. At a high brightness of about 10
000 cd A−1, it showed a current efficiency of 1.95 cd A−1, with a roll-off value of 33.7%. Even at the highest brightness of 18
287 cd m−2, the current and external quantum efficiencies were still about 50% of the maximum values. Furthermore, the device exhibited excellent spectrum stability (Fig. S13, ESI†). With the increased driving voltage from 6 to 10 V, the EL spectra remained almost unchanged, and the CIE coordinate values showed negligible variation, suggesting a remarkable voltage-independent EL emission. The excellent color stability should be attributed to the bulky structure of m-terphenyl that could suppress the π–π stacking in the solid state and then improve the morphological stability to resist morphological transition-induced deterioration during the device operation. The bluest emission was achieved in Py-2TF with the EL emission peak of 444 nm at a CIE coordinate of (0.15, 0.08), mainly due to the weak conjugation of 9,9′-positions in the fluorene moiety and the introduction of triphenylamine units with the induced highly twisted conformation and effectively suppressed π–π stacking.
Table 2 EL performances of Py-2pTPE, Py-2mTPE, Py-2TP, Py-2TF, Py-2NTF and TPE-2F
|
λ
EL (nm) |
V
on (V) |
L
max (cd m−2) |
η
P,max (lm W−1) |
η
C,max (cd A−1) |
η
ext,max (%) |
CIE (x, y) |
Abbreviations: Von = turn-on voltage at 1 cd m−2, Lmax = maximum luminance, ηP,max, ηC,max and ηext,max = maximum power, current and external efficiencies, respectively. CIE = Commission International de l'Éclairage coordinates. |
Py-2pTPE |
484 |
4.9 |
15 546 |
3.55 |
6.91 |
3.25 |
(0.20, 0.29) |
Py-2mTPE |
460 |
4.3 |
3514 |
1.82 |
2.85 |
2.19 |
(0.16, 0.17) |
Py-2TP |
448 |
4.2 |
18 287 |
1.79 |
2.94 |
3.46 |
(0.15, 0.09) |
Py-2TF |
444 |
3.1 |
9754 |
1.52 |
1.72 |
2.10 |
(0.15, 0.08) |
Py-2NTF |
456 |
3.9 |
6081 |
1.37 |
2.50 |
1.37 |
(0.17, 0.18) |
Py-2F |
452 |
4.3 |
1996 |
1.37 |
2.27 |
1.70 |
(0.16, 0.11) |
All the devices turned on at a low voltage from 3.1 to 4.9 V, disclosing small injection barriers from the transporting layer to the emitter in the OLED devices. Among them, Py-2TF possessed the lowest turn-on voltage at 3.1 V owing to the introduction of triphenylamine units, which could improve the hole-injection ability and enhance the power efficiency (1.52 lm W−1). When the triphenylamine groups were taken away in Py-2NTF, the turn-on voltage increased to 3.9 V, and the power efficiency dropped to 1.37 lm W−1. Good device performances were obtained in Py-2pTPE and Py-2mTPE, with Lmax, ηC,max and ηEQE,max values of 15
547 cd m−2, 6.91 cd A−1, 3.25% at a CIE coordinate of (0.20, 0.29) and 3514 cd m−2, 2.85 cd A−1 and 2.19% at a CIE coordinate of (0.16, 0.17), respectively. The EL emission blue-shifted from sky blue (484 nm) for Py-2pTPE to pure blue (460 nm) for Py-2mTPE, confirming the powerful control of intramolecular conjugation by simply changing the linkage mode again. In Py-2TP, when m-tetraphenylethene groups were replaced by m-terphenyl units, its EL emission further blue-shifted to deep blue (448 nm), as a result of the reduced conjugation of the unit and the meta-linkage mode of m-terphenyl. Moreover, its current efficiency and external quantum efficiency improved to 2.94 cd A−1 and 3.46%, respectively. As for Py-2F, it also had good device performance with ηC,max and ηEQE,max values of 2.27 cd A−1, 1.70% at a CIE coordinate of (0.16, 0.11), respectively, owing to the high carrier mobility of pyrene and twisted conformation of 9,9′-diphenylfluorenyl.
All the six AIEgens shared the same pyrene core, while different bulky side groups were introduced to be mainly responsible for their diverse EL performances. Analyzing the EL data carefully, we could find that accompanied by the AIE building blocks becoming more and more twisted, large blue-shifted emission could be achieved just as the transition from Py-2mTPE (460 nm) to Py-2NTF (456 nm) and to Py-2TF (444 nm). However, the highly twisted configuration will impede the carrier mobility, leading to inferior EL performance. Thus, Py-2TF just gave a current efficiency of 1.72 cd A−1 at a CIE coordinate of (0.15, 0.08). On the other hand, reducing the conjugation of AIE building block units might be an effective way for realizing deep blue emission as well as retaining excellent EL performance. Large blue-shifted emission was also observed through reducing the conjugation of the units from Py-2pTPE (484 nm) to Py-2F (452 nm) then to Py-2TP (448 nm). Excitedly, Py-2TP gave an excellent performance with ηC,max and ηEQE,max values up to 2.94 cd A−1 and 3.46% at a CIE coordinate of (0.15, 0.09) because of the short conjugation length of the m-terphenyl group and good carrier mobility of pyrene. However, if the side groups were too small to prevent the π–π stacking, aggregation caused quenching or red-shifted emission might be brought about, resulting in the possible inferior EL performance. Thus, a balance should be achieved for the selection of appropriate AIE building blocks, which could restrain the intermolecular π–π stacking exactly without sacrificing the carrier mobility to a large degree. The preliminary results confirmed that through optimizing the AIE building blocks, bluer and efficient AIEgens could be further developed.
3 Conclusions
In summary, six AIEgens including Py-2pTPE, Py-2mTPE, Py-2TP, Py-2TF, Py-2NTF and Py-2F, were designed, with excellent thermal stabilities and good AIE properties. By modifying the bulk size of the introduced AIE building blocks and changing the linkage modes, their emissions could be tuned from sky blue (484 nm) to deep blue (444 nm). When fabricated as emitters in OLEDs, all of the six AIEgens exhibited blue emissions and good EL performances, and Py-2TP demonstrated the best device performance with ηC,max and ηEQE,max values up to 2.94 cd A−1 and 3.46% in the deep blue region at a CIE coordinate of (0.15, 0.09), thanks to the suitable bulk and short conjugation of the m-terphenyl moiety. It is anticipated that m-terphenyl could be utilized to construct more high efficient deep blue AIEgens due to its facile synthesis and outstanding opto-electronic properties.
4 Experimental section
4.1 Characterizations
1H and 13C NMR spectra were recorded on a Mercury vx300 or Bruker Advance III (400 MHz) spectrometer. Mass spectra were measured on a MALDI-TOF mass spectrometer. Elemental analyses of carbon and hydrogen were performed on a Carlo-Erba-1106 microanalyzer. UV/Vis absorption spectra were recorded on a Shimadzu UV-2500 recording spectrophotometer. Photoluminescence spectra were recorded on a Hitachi F-4600 fluorescence spectrophotometer. Differential scanning calorimetry (DSC) was performed on a NETZSCH DSC 200 PC instrument from room temperature to 250 °C at a heating rate of 10 °C min−1 under argon. Thermogravimetric analysis (TGA) was performed on a NETZSCH STA 449C instrument. The thermal stabilities of the samples under a nitrogen atmosphere were determined by measuring their weight loss while heating from 25 to 670 °C at a rate of 10 °C min−1. Cyclic voltammetry (CV) was carried out on a CHI voltammetric analyzer in a three-electrode cell with a platinum counter electrode, an Ag/AgCl reference electrode, and a glassy carbon working electrode at a scan rate of 100 mV s−1 in anhydrous dichloromethane solution with 0.1 M tetrabutylammonium perchlorate (purchased from Alfa Aesar) as the supporting electrolyte and purged with nitrogen. The potentials obtained in reference to the Ag/Ag+ electrode were converted into values versus the saturated calomel electrode (SCE) by means of an internal ferrocenium/ferrocene (Fc+/Fc) standard. The powder X-ray diffraction patterns were recorded using a Rigaku MiniFlex 600 with an X-ray source of Cu Kα (λ = 1.5418 Å) at 25 °C at 40 kV and 15 mA at a scan rate of 10° (2θ) per min (scan range: 2–50°). The fluorescence quantum yields of powders were determined using a Hamamatsu C11347 Quantaurus-QY absolute fluorescence quantum yield spectrometer.
4.2 Computational details
The geometrical and electronic properties were optimized at the B3LYP/6-31g* level using the Gaussian 09 program. The molecular orbitals were obtained at the same level of theory.
4.3 OLED device fabrication and measurement
NPB and TPBI were purchased from Aldrich Chemical Co. and were purified by thermal sublimation. In a general procedure, indium-tin oxide (ITO)-coated glass substrates were etched, patterned, and washed with detergent, deionized water, acetone, and ethanol in turn. A typical multilayer device ITO/polyethylenedioxythiophene-polystyrene sulfonic acid (PEDOT:PSS, 30 nm)/NPB (40 nm)/EML (10–20 nm)/TPBi (35 nm)/Ca:Ag (100 nm) was fabricated, where NPB and TPBI served as the hole-transporting and the hole-blocking layer, respectively. PEDOT:PSS was used to smooth the ITO surface and to decrease the hole injection barrier, and it is important to improve the device efficiency. The emitting layer thickness was varied from 10 to 20 nm. All the organic layers were successively deposited by means of conventional vacuum deposition onto the ITO/glass substrate at a pressure of 8 × 10−5 Pa. The active area of device was 6 mm2. A quartz crystal oscillator placed near the substrate was used to monitor the thickness of each layer, which was calibrated ex situ using an Ambios Technology XP-2 surface profilometer. EL spectra and chromaticity coordinates were measured using a SpectraScan PR 655 photometer. All device tests were carried out under an ambient atmosphere at room temperature. Current density–voltage–luminance (J–V–L) measurements were conducted simultaneously using a Keithley 4200 semiconductor parameter analyzer combined with a Newport multifunction 2835-C optical meter, with luminance being measured in the forward direction.
4.4 Preparation of the compounds
All other chemicals and reagents were obtained from commercial sources and used as received. Solvents for chemical synthesis were purified according to standard procedures. The Ar–Br intermediates were synthesized according to literature procedures.
Synthesis of compound 1.
Under an atmosphere of nitrogen, [Ir(m-OMe)cod]2 (0.060 g, 0.09 mmol), 4,4′-di-tert-butyl-2,2′-bipyridine (dtbpy, 0.048 g, 0.18 mmol), and bis(pinacolato)diboron (0.1 g, 0.39 mmol) were dissolved in THF (5 mL). The mixture was added to a Schlenk tube containing pyrene (1.80 g, 8.90 mmol) and bis(pinacolato)diboron (4.86 g, 19.1 mmol). After the addition of THF (10 mL), the reaction mixture was stirred at 80 °C for 24 h under N2. Then, the mixture was extracted with dichloromethane, the combined organic extracts were dried over anhydrous Na2SO4 and concentrated by rotary evaporation. The crude product was first purified by column chromatography on silica gel using dichloromethane/petroleum ether (1
:
1 v/v) as the eluent, and then recrystallized from petroleum ether. A white solid was finally obtained in a yield of 40% (1.616 g). 1H NMR (300 MHz, CDCl3, δ): 8.62 (s, 4H), 8.08 (s, 4H), 1.46 (s, 24H).
Synthesis of TPE-pBr.
A solution of 4,4′-di-tert-butyldiphenylmethane (8.41 g, 30 mmol) in anhydrous THF (50 mL) was stirred at 0 °C for 15 minutes under an argon atmosphere. Then, a solution of n-BuLi in hexane (24 mmol, 11 mL, 2.2 M) was added dropwise. After being stirred for 1 h at that temperature, the solution of 4-bromobenzophenone (5.22 g, 20 mmol) dissolved in THF (30 mL) was added. 2 hours later, the mixture was slowly warmed to room temperature. Then, the reaction was quenched with a saturated aqueous solution of ammonium chloride. The mixture was extracted with dichloromethane, and the organic layer was evaporated after drying with anhydrous sodium sulfate. The crude product was dissolved in toluene (100 mL), then p-toluenesulfonic acid (0.76 g, 4 mmol) was added and the mixture was refluxed overnight. After being cooled to room temperature, the mixture was evaporated, and the crude product was purified by silica gel column chromatography using petroleum ether as eluent to yield a white powder (3.6 g, 34.6%). 1H NMR (300 MHz, CDCl3, δ): 7.18–7.21 (d, 2H), 7.07–7.13 (m, 7H), 6.86–6.98 (m, 8H), 1.24–1.26 (d, 18H). 13C NMR (75 Hz, CDCl3, δ): 149.5, 149.3, 143.6, 143.1, 141.5, 140.3, 140.2, 138.6, 133.0, 131.3, 130.8, 130.6, 127.5, 126.2, 124.5, 124.3, 120.0, 34.4, 34.3, 31.2. Anal. calcd for C34H35Br: C, 78.00; H, 6.74. Found: C, 78.42; H, 6.83.
Synthesis of TPE-mBr.
A solution of 4,4′-di-tert-butyldiphenylmethane (8.41 g, 30 mmol) in THF (50 mL) was stirred at 0 °C for 15 minutes under an argon atmosphere. Then, a solution of n-BuLi in hexane (24 mmol, 11 mL, 2.2 M) was added dropwise. After being stirred for 1 h at that temperature, the solution of 3-bromobenzophenone (5.22 g, 20 mmol) in THF (30 mL) was added. 2 hours later, the mixture was slowly warmed to room temperature. Then, the reaction was quenched with an aqueous solution of ammonium chloride, and the resultant mixture was extracted with dichloromethane. The organic layer was evaporated after drying with anhydrous sodium sulfate, and the crude product was dissolved in toluene (100 mL). Then, p-toluenesulfonic acid (0.76 g, 4 mmol) was added, and the mixture was refluxed overnight. After being cooled to room temperature, the mixture was evaporated and the crude product was purified by silica gel column chromatography using petroleum ether as eluent to yield a white powder (4.68 g, 45%). 1H NMR (300 MHz, CDCl3, δ): 7.17–7.19 (d, 1H), 7.07–7.14 (m, 8H), 6.91–7.00 (m, 8H), 1.24–1.26 (d, 18H). 13C NMR (75 Hz, CDCl3, δ): 149.6, 149.4, 146.2, 143.3, 142.0, 140.1, 138.4, 134.1, 131.2, 130.8, 129.9, 129.0, 128.9, 127.6, 126.3, 124.4, 124.3, 121.5, 34.4, 34.3, 31.2. Anal. calcd for C34H35Br: C, 78.00; H, 6.74. Found: C, 78.17; H, 6.70.
General procedure for the synthesis of Py-2pTPE, Py-2mTPE, Py-2TP, Py-2TF, Py-2NTF, Py-2F.
Py-2pTPE.
Compound 1 (181 mg, 0.4 mmol), TPE-pBr (419 mg, 0.8 mmol), Pd(PPh3)4 (0.10 g, 0.08 mmol) and potassium carbonate (276 mg, 2 mmol) in toluene (20 mL) and distilled water (7 mL) were added into a 200 mL Schlenk tube. The resultant mixture was refluxed for 2 days under argon, and then extracted with dichloromethane. The combined organic extracts were dried over anhydrous Na2SO4 and concentrated by rotary evaporation. The crude product was purified by column chromatography on silica gel using chloroform/petroleum ether (v/v = 1/5) as eluent, and further purified by recrystallization in petroleum ether. A yellow solid was finally produced in a yield of 35% (152 mg). 1H NMR (300 MHz, CDCl3, δ): 8.35 (s, 4H), 8.09 (s, 4H), 7.62–7.64 (d, 4H), 7.12–7.20 (m, 22H), 7.03–7.05 (d, 4H), 6.96–6.99 (d, 4H), 1.26 (s, 36H). 13C NMR (100 MHz, CDCl3, δ): 149.5, 149.4, 144.4, 143.7, 141.4, 140.9, 139.7, 138.9, 138.7, 132.3, 131.7, 131.6, 131.2, 128.0, 127.8, 127.1, 126.4, 124.7, 124.5, 123.8, 123.7, 34.6, 31.5. MS (MALDI TOF), m/z: 1086.5333 ([M+], calcd for C84H78, 1086.61). Anal. calcd for C84H78: C, 92.77; H, 7.23. Found: C, 93.08; H, 7.18.
Py-2mTPE.
Light yellow solid (yield: 32%). 1H NMR (300 MHz, CDCl3, δ): 7.97–8.00 (d, 8H), 7.59–7.62 (d, 4H), 7.24–7.32 (m, 6H), 6.99–7.13 (m, 24H), 1.25–1.27 (d, 36H). 13C NMR (100 MHz, CDCl3, δ): 149.5, 149.4, 144.9, 144.0, 141.6, 141.3, 140.7, 140.2, 139.0, 132.1, 131.6, 131.4, 131.1, 128.4, 127.9, 127.8, 126.4, 125.8, 124.8, 124.6, 123.8, 34.7, 34.6, 31.5. MS (MALDI TOF), m/z: 1086.4846 ([M+], calcd for C84H78, 1086.61). Anal. calcd for C84H78: C, 92.77; H, 7.23. Found: C, 93.29; H, 7.15.
Py-2TP.
White solid (yield: 73%). 1H NMR (300 MHz, CDCl3, δ): 8.53 (s, 4H), 8.21 (s, 4H), 8.10 (s, 4H), 7.90 (s, 2H), 7.79–7.82 (d, 8H), 7.52–7.57 (t, 8H), 7.42–7.46 (t, 4H). 13C NMR (75 MHz, CDCl3, δ): 142.5, 141.1, 131.6, 128.8, 128.0, 127.5, 127.4, 126.0, 125.4, 124.0. MS (MALDI TOF), m/z: 658.1686 ([M+], calcd for C52H34, 658.27). Anal. calcd for C52H34: C, 94.8; H, 5.20. Found: C, 94.31; H, 4.99.
Py-2TF.
White solid (yield: 74%). 1H NMR (300 MHz, CDCl3, δ): 8.32 (s, 4H), 8.08 (s, 4H), 7.93–7.82 (d, 4H), 7.18–7.44 (d, 4H), 7.50–7.53 (d, 4H), 7.30–7.40 (m, 12H), 7.20–7.23 (m, 8H), 7.07–7.15 (m, 12H), 6.93–7.01 (m, 8H). 13C NMR (75 MHz, CDCl3, δ): 151.3, 147.6, 146.2, 145.3, 140.1, 139.6, 139.3, 131.3, 129.1, 128.9, 128.7, 127.8, 127.7, 127.4, 126.2, 124.3, 123.6, 123.1, 122.7, 120.1, 64.6. MS (MALDI TOF), m/z: 1168.3335 ([M+], calcd for C90H60N2, 1168.48). Anal. calcd for C90H60N2: C, 92.43; H, 5.17; N, 2.40. Found: C, 92.15; H, 4.95, N, 2.45.
Py-2NTF.
White solid (yield: 58%). 1H NMR (300 MHz, CDCl3, δ): 8.32 (s, 4H), 8.09 (s, 4H), 7.79–7.82 (d, 4H), 7.71–7.74 (d, 4H), 7.48–7.51 (d, 4H), 7.31–7.39 (m, 12H), 7.16–7.19 (d, 4H), 7.06–7.09 (d, 4H), 2.32 (s, 6H). 13C NMR (75 MHz, CDCl3, δ): 151.3, 145.3, 142.7, 140.1, 139.6, 138.4, 136.3, 131.4, 128.9, 128.7, 128.0, 127.8, 127.7, 127.4, 126.1, 123.6, 120.1, 64.93, 20.90. MS (MALDI TOF), m/z: 862.2351 ([M+], calcd for C68H46, 862.36). Anal. calcd for C68H46: C, 94.63; H, 5.37. Found: C, 94.18; H, 5.56.
Py-2F.
White solid (yield: 62%). 1H NMR (300 MHz, CDCl3, δ): 8.34 (s, 4H), 8.11 (s, 4H), 7.82–7.94 (m, 8H), 7.21–7.45 (m, 14H), 7.06–7.09 (d, 8H), 2.30 (s, 12H). 13C NMR (100 MHz, CDCl3, δ): 152.5, 151.9, 143.1, 141.0, 139.7, 139.5, 139.0, 136.3, 131.4, 129.1, 128.2, 127.9, 127.5, 126.2, 125.7, 123.8, 123.7, 120.7, 120.3, 65.1, 21.0. MS (MALDI TOF), m/z: 890.2684 ([M+], calcd for C70H50, 890.39). Anal. calcd for C70H50: C, 94.34; H, 5.66. Found: C, 93.95; H, 5.82.
Acknowledgements
We are grateful to the National Fundamental Key Research Program (2013CB834701) and the National Science Foundation of China (No. 21325416, 61106017, and 51573140) for financial support.
Notes and references
-
(a) M. A. Baldo, M. E. Thompson and S. R. Forrest, Nature, 2000, 403, 750 CrossRef CAS PubMed;
(b) B. W. D'Andrade and S. R. Forrest, Adv. Mater., 2004, 16, 1585 CrossRef.
-
(a) T. Arakane, M. Funahashi, H. Kuma, K. Fukuoka, K. Ikeda, H. Yamamoto, F. Moriwaki and C. Hosokawa, Soc. Inf. Disp. Int. Symp. Dig. Tech. Pap., 2006, 37, 37 CrossRef;
(b) K. T. Kamtekar, A. P. Monkman and M. R. Bryce, Adv. Mater., 2010, 22, 572 CrossRef CAS PubMed.
-
(a) M. Zhu and C. Yang, Chem. Soc. Rev., 2013, 42, 4963 RSC;
(b) X. Yang, X. Xu and G. Zhou, J. Mater. Chem. C, 2015, 3, 913 RSC;
(c) S. K. Kim, B. Yang, Y. Ma, J. H. Lee and J. W. Park, J. Mater. Chem., 2008, 18, 3376 RSC;
(d) C. H. Wu, C. H. Chien, F. M. Hsu, P. I. Shih and C. F. Shu, J. Mater. Chem., 2009, 19, 146 Search PubMed;
(e) P. Shih, C. Chuang, C. Chien, E. Diau and C. Shu, Adv. Funct. Mater., 2007, 17, 3141 CrossRef CAS;
(f) B. Kim, Y. Park, J. Lee, D. Yokoyama, J. Lee, J. Kido and J. Park, J. Mater. Chem. C, 2013, 1, 432 RSC;
(g) Y. Zhang, G. Cheng, Y. Zhao, J. Hou, S. Liu, S. Tang and Y. Ma, Appl. Phys. Lett., 2005, 87, 241112 CrossRef;
(h) S. Tang, M. Liu, P. Lu, G. Cheng, M. Zeng, Z. Xie, H. Xu, H. Wang, B. Yang and Y. Ma, and D. Yan, Org. Electron., 2008, 9, 241–252 CrossRef CAS;
(i) Y. Yuan, J. Chen, F. Lu, Q. Tong, Q. Yang, H. Mo, T. Ng, F. Wong, Z. Guo, J. Ye, Z. Chen, X. Zhang and C. Lee, Chem. Mater., 2013, 25, 4957 CrossRef CAS;
(j) L. Yao, B. Yang and Y. Ma, Sci. China: Chem., 2014, 57, 335 CrossRef CAS.
- T. Duarte and K. Müllen, Chem. Rev., 2011, 111, 7260 CrossRef PubMed.
-
(a) S. Jiao, Y. Liao, X. Xu, L. Wang, G. Yu, L. Wang, Z. Su, S. Ye and Y. Liu, Adv. Funct. Mater., 2008, 18, 2335 CrossRef CAS;
(b) A. Thangthong, N. Prachumrak, R. Tarsang, T. Keawin, S. Jungsuttiwong, T. Sudyoadsuk and V. Promarak, J. Mater. Chem., 2012, 22, 6869 RSC;
(c) S. Tao, Y. Zhou, C. Lee, X. Zhang and S. Lee, Chem. Mater., 2010, 22, 2138 CrossRef CAS;
(d) J. Lee and J. Park, Org. Lett., 2015, 17, 3960 CrossRef CAS PubMed;
(e) J. Moorthy, P. Natarajan, P. Venkatakrishnan, D. Huang and T. J. Chow, Org. Lett., 2007, 25, 5215 CrossRef PubMed;
(f) P. Kotchapradist, N. Prachumrak, R. Tarsang, S. Jungsuttiwong, T. Keawin, T. Sudyoadsuk and V. Promarak, J. Mater. Chem. C, 2013, 1, 4916 RSC;
(g) J. Gu, G. Xie, L. Zhang, S. Chen, Z. Lin, Z. Zhang, J. Zhao, L. Xie, C. Tang, Y. Zhao, S. Liu and W. Huang, J. Phys. Chem. Lett., 2010, 1, 2849 CrossRef CAS;
(h) Z. Zhao, S. Chen, J. W. Y. Lam, P. Lu, Y. Zhong, K. S. Wong, H. S. Kwok and B. Z. Tang, Chem. Commun., 2010, 46, 2221 RSC;
(i) J. Yang, J. Huang, N. Sun, Q. Peng, Q. Li, D. Ma and Z. Li, Chem. – Eur. J., 2015, 21, 6862 CrossRef CAS PubMed.
-
(a) J. Luo, Z. Xie, J. W. Y. Lam, L. Cheng, H. Chen, C. Qiu, H. S. Kwok, X. Zhan, Y. Liu, D. Zhu and B. Z. Tang, Chem. Commun., 2001, 1740 RSC;
(b) Y. Hong, J. W. Y. Lam and B. Z. Tang, Chem. Soc. Rev., 2011, 40, 5361 RSC;
(c) J. Mei, N. Leung, R. Kwok, J. Lam and B. Z. Tang, Chem. Rev., 2015, 115, 11718 CrossRef CAS PubMed;
(d) X. Zhang, X. Zhang, B. Yang and Y. Wei, Chin. J. Polym. Sci., 2014, 32, 871 CrossRef CAS;
(e) X. Zhang, X. Zhang, B. Yang and Y. Wei, Chin. J. Polym. Sci., 2014, 32, 1479 CrossRef CAS;
(f) X. Ji, P. Wang, H. Wang and F. Huang, Chin. J. Polym. Sci., 2015, 33, 890 CrossRef CAS;
(g) Q. Li and Z. Li, Sci. China: Chem., 2015, 58, 1800 CrossRef CAS;
(h) Z. Li, Sci. China: Chem., 2015, 58, 969 CrossRef CAS;
(i) W. Wu, R. Tang, Q. Li and Z. Li, Chem. Soc. Rev., 2015, 44, 3997 RSC;
(j) C. Wang, B. Xu, M. Li, Z. Chi, Y. Xie, Q. Li and Z. Li, Mater. Horiz., 2016, 3, 220–225 RSC.
-
(a) J. Huang, N. Sun, P. Chen, R. Tang, Q. Li, D. Ma and Z. Li, Chem. Commun., 2014, 50, 2136 RSC;
(b) J. Huang, N. Sun, Y. Dong, R. Tang, P. Lu, P. Cai, Q. Li, D. Ma, J. Qin and Z. Li, Adv. Funct. Mater., 2013, 23, 2329 CrossRef CAS;
(c) J. Yang, J. Huang, Q. Li and Z. Li, J. Mater. Chem. C, 2016, 4, 2663 RSC;
(d) J. Huang, M. Yang, J. Yang, R. Tang, S. Ye, Q. Li and Z. Li, Org. Chem. Front., 2015, 2, 1608 RSC.
- X. Zhan, N. Sun, Z. Wu, J. Tu, L. Yuan, X. Tang, Y. Xie, Q. Peng, Y. Dong, Q. Li, D. Ma and Z. Li, Chem. Mater., 2015, 27, 1847 CrossRef CAS.
-
(a) W. Qin, Z. Yang, Y. Jiang, J. W. Y. Lam, G. Liang, H. S. Kwok and B. Z. Tang, Chem. Mater., 2015, 27, 3892 CrossRef CAS;
(b) Q. Zeng, Z. Li, Y. Dong, C. Di, A. Qin, Y. Hong, L. Ji, Z. Zhu, C. Jim, G. Yu, Q. Li, Z. Li, Y. Liu, J. Qin and B. Z. Tang, Chem. Commun., 2007, 70 RSC;
(c) L. Li, M. Chen, H. Zhang, H. Nie, J. Sun, A. Qin and B. Z. Tang, Chem. Commun., 2015, 51, 4830 RSC.
-
(a) J. Huang, J. Su and H. Tian, J. Mater. Chem., 2012, 22, 10977 RSC;
(b) S. Kim, B. Kim, J. Lee, H. Shin, Y. Park and J. Park, Mater. Sci. Eng., R, 2016, 99, 1 CrossRef.
-
(a) J. Huang, R. Tang, T. Zhang, Q. Li, G. Yu, S. Xie, Y. Liu, S. Ye, J. Qin and Z. Li, Chem. – Eur. J., 2014, 20, 5317 CrossRef CAS PubMed;
(b) J. Zou, H. Wu, C. Lam, C. Wang, J. Zhu, C. Zhong, S. Hu, C. Ho, G. Zhou, H. Wu, W. Choy, J. Peng, Y. Cao and W. Wong, Adv. Mater., 2011, 23, 2976 CrossRef CAS PubMed.
-
(a) J. Huang, Y. Jiang, J. Yang, R. Tang, N. Xie, Q. Li, H. S. Kwok, B. Z. Tang and Z. Li, J. Mater. Chem. C, 2014, 2, 2028 RSC;
(b) J. Yang, N. Sun, J. Huang, Q. Li, Q. Peng, X. Tang, Y. Dong, D. Ma and Z. Li, J. Mater. Chem. C, 2015, 3, 2624 RSC;
(c) J. Huang, N. Sun, J. Yang, R. Tang, Q. Li, D. Ma and Z. Li, Adv. Funct. Mater., 2014, 24, 7645 CrossRef CAS.
-
(a) Y. Dong, J. Lam, A. Qin, Z. Li, J. Sun, H. Sung, I. Williams and B. Z. Tang, Chem. Commun., 2007, 40 RSC;
(b) X. Luo, J. Li, C. Li, L. Heng, Y. Don, Z. Liu, Z. Bo and B. Z. Tang, Adv. Mater., 2011, 23, 3261 CrossRef CAS PubMed.
- J. Mei, Y. Hong, J. Lam, A. Qin, Y. Tang and B. Z. Tang, Adv. Mater., 2014, 26, 5429 CrossRef CAS PubMed.
Footnotes |
† Electronic supplementary information (ESI) available: Structures of some good blue materials, DSC curves, TGA curves, UV-vis spectra, PL spectra and photographs, XRD data, HOMOs and LUMOs, optimized structures, CV plots and EL curves for part or whole of the six blue AIE luminogens, Py-2pTPE, Py-2mTPE, Py-2TP, Py-2TF, Py-2NTF and Py-2F. See DOI: 10.1039/c6qm00014b |
‡ These authors contributed equally to this work. |
|
This journal is © the Partner Organisations 2017 |