DOI:
10.1039/C6QI00457A
(Research Article)
Inorg. Chem. Front., 2017,
4, 160-164
Instantaneous formation of polyoxometalate-based cerium vanadium oxide gels†
Received
27th October 2016
, Accepted 24th November 2016
First published on 25th November 2016
Abstract
The instantaneous formation of mechanically stable cerium vanadium oxide gels starting from soluble polyoxovanadates is reported together with initial application studies. Upon addition of phosphoric acid to solutions containing a vanadium oxide source (e.g. (nBu4N)4[V4O12]) and Ce3+, instantaneous formation (reaction time <1 s) of a vanadium oxide gel is observed. The gel shows unique mechanical and thermal stabilities (up to ∼180 °C). High permeability of the gel is observed, allowing its use for long-term acid delivery into aqueous media or for the adsorption of organic aromatic dye pollutants from solution. A range of spectroscopy and electron microscopy techniques provide insight into the gel formation and the gel composition: an intertwined 3D matrix of nanowires (d ∼ 10 nm) containing cerium, vanadium oxide and phosphate is identified as an inorganic matrix which enables the formation of the mechanically stable gel.
Introduction
Polyoxometalates (POMs) are molecular analogues of solid-state metal oxides.1 They have attracted vast interest in academia and industry due to their widespread application in (photo)-catalysis,2,3 energy storage,4,5 molecular electronics6 and nanomaterials science.7 POMs can be employed under homogeneous or heterogeneous conditions. While the use of POMs under homogeneous conditions provides detailed insight into reactivity and solution behaviour, many technological applications rely on heterogeneous systems, e.g. for facile separation and recycling.8 The need to embed POMs in heterogeneous systems has been recognized and various routes for POM heterogenization have been reported.9 One promising approach is the use of POMs as precursors for the formation of metal oxide gels. This approach allows the tuning of POM precursor properties on the molecular level; further fine-tuning of the materials’ properties is possible by incorporation of additional reactive components. POM-based gels with unique optical,10 electrochemical11,12 and thermo-/photoresponsive behaviour13 have been developed. Polyoxovanadates are particularly attractive precursors for gel formation as their conversion into nanostructured vanadium oxide gels is well-known.14 Vanadium oxide gels are technologically highly relevant materials15 and their use in solar energy conversion,16,17 (bio)sensors,18,19 porous materials20,21 and battery electrodes22,23 has been reported.
Results and discussion
Gel synthesis
Here, we report a novel synthetic route which enables the instantaneous formation of a cerium vanadium-oxide based organogel. The gel is formed spontaneously when a basic organic solution containing a vanadate precursor (e.g. (nBu4N)3[H3V10O28]), nBu4NOH and Ce3+ is acidified using aqueous phosphoric acid (Fig. 1). Gelation occurs instantaneously (tgelation < 1 s) and a mechanically stable gel which can be cast into a desired shape (disk, cylinder, etc.) and manipulated using tweezers is formed. The high mechanical stability of the gel is shown, as the gel is self-supporting and can be cast or cut into the required shape (Fig. 1).
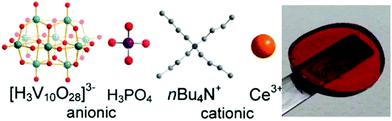 |
| Fig. 1 Left: Components required for gel formation: a vanadium oxide source, typically a polyoxovanadate e.g. (nBu4N)3[H3V10O28] is dissolved in basic (nBu4NOH-containing) organic solution (e.g. DMSO) and acidified using phosphoric acid in the presence of Ce3+ cations. Instantaneous gelation is observed. Right: photograph of a typical free-standing gel sample. | |
The formation of the gel was discovered by serendipity when we investigated the synthesis of lanthanide-functionalized polyoxovanadate clusters in organic solution.24,25 It was observed that when a basic DMSO solution containing nBu4NOH, (nBu4N)3[H3V10O28] and Ce(NO3)3·6H2O is acidified with aqueous phosphoric acid, the solution viscosity instantaneously increases and a mechanically stable gel is obtained. Systematic analysis of the reaction parameters showed that both Ce3+ and phosphoric acid are required for gel formation: attempts to replace Ce3+ with a wide range of other metal cations, including lanthanides, alkali and alkali earth metals did not result in the formation of a gel. Gelation is therefore specific to Ce3+. While the exact role of Ce3+ is still unclear, we suggest that Ce3+ acts as an ionic cross-linker between the building blocks of the gel.26 Attempts to replace phosphoric acid with other mineral or organic acids (HClaq, HNO3, H2SO4, acetic acid, benzoic acid) did not result in gelation, indicating that phosphate anions are also critical for the gel formation. In contrast, the vanadium oxide source can be varied and gel formation is possible with a range of soluble vanadium oxide precursors such as (nBu4N)4[V4O12], (nBu4N)3[H3V10O28] or hydrolysed V2O5. As the synthesis is carried out under basic conditions (i.e. in the presence of nBu4NOH), the compounds are converted into metavanadate-type precursors,27–29 thus explaining their similar gel-forming reactivities upon acidification. For reasons of brevity, this report is focused on gels based on (nBu4N)3[H3V10O28].‡
It was further demonstrated that gel formation is achieved in a range of polar organic solvents e.g. dimethyl sulfoxide (DMSO), N,N′-dimethyl formamide (DMF) and water, giving access to vanadium oxide organogels and hydrogels. However, lower mechanical stability was observed for the hydrogel compared with the respective organogels, therefore this report is focused on DMSO-based gels.‡
Gel characterization
51V-NMR-spectroscopy.
Investigation of the gelation processes is non-trivial, particularly if multi-component systems are present. We have used 51V-NMR spectroscopy to investigate the gelation process. To this end samples of the precursor solution containing (nBu4N)3[H3V10O28] in DMSO/nBu4NOH and solutions/gels formed at increasing H3PO4 concentration (i.e. acidic pH) were examined. The data (Fig. 2) show significant changes of the chemical shifts upon gelation: 51V-NMR data show that the basic precursor solution ((nBu4N)3[H3V10O28] in DMSO/nBu4NOH) contains several small vanadate species as observed by four distinct signals in the range of δ = −500 to −600 ppm, corresponding to mononuclear orthovanadate species [VO4]3− (δ = −542 ppm), [HVO4]2− (δ = −564 ppm) and [H2VO4]− (δ = −571 ppm) as well as oligomeric “metavanadate”-related species, e.g. [V3O10]5− (δ = −514 ppm).30
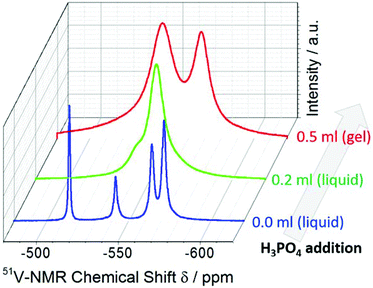 |
| Fig. 2
51V-NMR data showing the changes in chemical shifts observed for the vanadium centers during gel formation. Conditions: solvent: DMSO; precursor: (nBu4N)3[H3V10O28] hydrolyzed by addition of nBu4NOH; acid: H3PO4 (9 M). | |
Upon H3PO4 addition (but still in the non-gelled form), one major signal arises at δ = −554 ppm (assigned to dinuclear [V2O7]4− species),30 while the resonance at δ = −542 ppm can be assigned to residual [VO4]3−.30 The data therefore suggest that the initial steps of acid-induced vanadium oxide poly-condensation are observed. Upon gelation, two distinct signals are observed by 51V-NMR spectroscopy at δ = −568 ppm and δ = −544 ppm, indicating that two vanadate coordination environments are present within the gel structure. The chemical shifts observed are in line with tetrahedrally coordinated vanadate centres in an all-oxo environment.30 Note that other possible vanadium coordination geometries would be observed at significantly different 51V-NMR chemical shifts: square pyramidal [VO5] units in solid-state vanadium oxide gels are observed at δ ca. 280 ppm,15 while octahedral [VO6] units are observed at δ ca. 400–500 ppm (e.g. in [V10O28]6−).3031P-NMR data show that in the gel, phosphate-based building blocks are observed, see the ESI.† The data therefore suggest that two distinct tetrahedral vanadate building blocks are present in the nanofibers together with phosphate groups, and future work will use high-resolution TEM, X-ray photoelectron spectroscopy and theoretical modelling to gain further insight into the detailed chemical structure.
Electron microscopy.
The micro- and nanostructure of the gel was examined using scanning electron microscopy (SEM), transmission electron microscopy (TEM) and high-angle annular dark field-scanning transmission electron microscopy (HAADF-STEM). SEM imaging of the semi-dried gel shows a smooth surface (Fig. 3a and b). More detailed TEM (Fig. 3c and d) and HAADF-STEM analysis (Fig. S7†) revealed that the gel was stabilized by a vast network of intertwined fibrillary structures. The fibres observed feature large aspect ratios and typical diameters of <10 nm (Fig. 3c and d). Based on previous findings,15,31 these structures are assigned as vanadium oxide fibres which is further supported by the 51V-NMR data and also FT-IR data which show distinct V–O vibrational modes within the gel (Fig. S1†). EDS analysis showed that these fibrillar features contain large amounts of vanadium together with smaller amounts of phosphate and cerium (Fig. S8†). The presence of vanadium was detected by their characteristic X-ray emission signals at 4.95 and 0.51 eV (Kα and Lα, respectively). The Ce Lα signal was observed at 4.84 eV (overlaid with the V signal at 4.95 eV), while the M emission can be detected at 0.88 eV (a purely Ce-based signal, see the ESI, Fig. S8b†). The data highlight that vanadate, phosphate and cerium components are present within these inorganic nanofibers, however, further work is underway to gain more detailed structural insight into the specific role of each component.
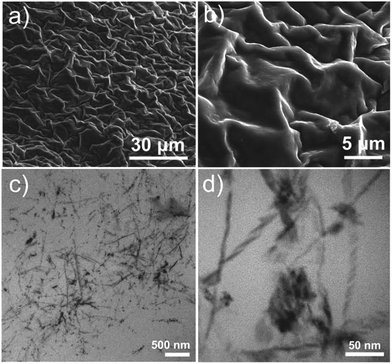 |
| Fig. 3 Electron microscopic characterization of the gel. Top (a, b): Scanning electron microscopy (SEM) images showing the smooth gel surface. Bottom (c, d): Transmission electron microscopy (TEM) images showing the internal network of cerium vanadium oxide/phosphate fibres stabilizing the gel matrix. | |
Gel properties and applications
Thermal stability.
Initial observations showed that the gels possess a high thermal stability and do not feature a de-gelation temperature where the system obtains enough thermal energy to overcome the gel-forming interactions. This corroborates our observation that the gel is stabilized by an internal network of intertwined vanadium oxide fibres which are thermally stable and do not allow reversible de-gelation by temperature increase. When the gel reaches the boiling-point of the corresponding solvent (e.g. DMSO: Tevaporation = 189 °C), irreversible thermal decomposition of the gel is observed due to solvent-loss. Below the given decomposition temperature, the gel is not irreversibly changed: for the DMSO-based gel, a loss of solvent (<5 wt%) is observed upon prolonged heating (24 h) to 100 °C. The solvent loss leads to a reversible shrinking of the gel: immersion of the de-solvated gel into DMSO results in a re-uptake of the solvent to reconstitute the original gel shape.
Chemical stability.
The stability of the gel against high ionic strength solutions (aqueous, 1 M NaCl solution) was investigated by immersing pre-formed gel cylinders (l = 1.0 cm, d = 0.85 cm) into the salt solution for 24 h. No degradation or loss of shape or mechanical stability of the gel was observed, highlighting the stable internal support structure. Similar experiments were conducted using a range of organic low-polarity solvents such as hexane, diethyl ether, ethyl acetate, toluene and no gel degradation was observed, further illustrating the stable network present within the gel.
Pollutant removal.
It was hypothesized that the accessible internal structure of the gels might make them useful as solid adsorbents for the uptake of organic pollutants from aqueous solution. As a model pollutant the azo-dye basic blue 41 (BB41) was used.32 In a typical experiment, a cylindrical piece of gel (diameter 0.85 cm, height 1.0 cm, surface area: 3.8 cm2, volume: 0.56 cm3) was exposed to an aqueous solution of BB41 ([BB41] = 12 mM) and the solution was left to equilibrate. The dye uptake kinetics were followed UV-Vis-spectrometrically and pseudo first-order uptake kinetics were observed and dye absorption reached a steady state after ca. 96 h (Fig. 4) with a maximum dye removal from solution of ∼87%. The dye-loaded gel was still mechanically stable and was removed from the solution using tweezers.
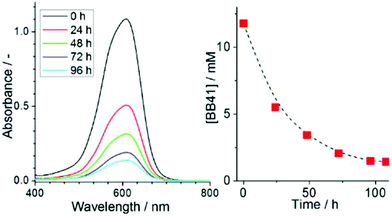 |
| Fig. 4 Removal of the organic model pollutant BB41 from aqueous solution by using the vanadium oxide gel. Conditions: V = 3.0 ml, [BB41]0 = 12 mM; gel surface area: 3.5 cm2, gel volume: 0.5 cm3. | |
Acid release.
Due to the synthetic procedure it was noted that the POM-gels are acidic in nature and it was suggested that the gels could be used for the slow release of H3O+ into solution. This was exemplified by exposing a piece of the DMSO-based gel cylinder (diameter 0.85 cm, height 1.0 cm, surface area: 3.8 cm2, volume: 0.56 cm3) to an aqueous solution (V = 3.0 ml, initial pH upon immersion of the gel cylinder: pH 3.5, due to the presence of H3PO4 on the gel surface, Fig. 5). The solution pH was reduced in an exponential fashion and equilibrium was reached after 90 min, resulting in a final solution pH of 2.4. After the H3O+ release, the gel remains unchanged and can be separated from the solution using tweezers. The gel can be re-charged with acid by immersion in a DMSO-solution containing H3PO4 and can be re-used for a second acid release run in water (ESI†). It was further demonstrated that the H3O+ release can be modulated by varying the accessible surface area of the gel. To this end, the original gel cylinders were cut into halves or quarters using a scalpel, resulting in increased surface areas. Acid release into solution increased with increasing surface area, see Fig. 5. The results show that the gel can be used as a solid source of protons which are released into solution with over a prolonged period of time, making it an interesting candidate for the study of slow, proton-dependent reactions.
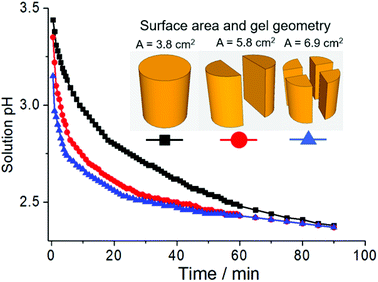 |
| Fig. 5 Surface-area dependent acid release of gel samples into aqueous solution (V = 3.0 ml), highlighting that the gel can be used for continuous long-term acid release. The release rate can be controlled by the surface area of the gel. Gel geometries and surface areas A used for release are shown as the inset. | |
Conclusions
In summary, we report a facile route which allows the instantaneous formation of mechanically stable cerium vanadium oxide gels. Material characterization provides insight into the gel structure and it is shown that the gel is stabilized by intertwined vanadium oxide nanofibers (d ∼ 10 nm). Initial reactivity studies show that the gel features unprecedented thermal stability, does not de-gelate upon heating, can deliver protons into aqueous solution and shows promising pollutant adsorption properties in aqueous solution. Future studies will further explore the molecular-level structure of the gel and aim at further analysis of the adsorbent properties of the gel.
Acknowledgements
Financial support by the Deutscher Akademischer Austauschdienst (DAAD) EU COST Action CM1203, Ulm University, Friedrich-Alexander-Universität Erlangen-Nürnberg and Fundación General CSIC (Programa ComFuturo) is gratefully acknowledged. The authors would like to acknowledge the use of The Advanced Microscopy Laboratory (LMA-INA).
Notes and references
- L. Cronin, A. Müller and (guest eds.), Chem. Soc. Rev., 2012, 41, 7325–7648.
- C. L. Hill, J. Mol. Catal. A: Chem., 2007, 262, 2–6 CrossRef CAS.
- C. Streb, Dalton Trans., 2012, 41, 1651 RSC.
- A. Sartorel, M. Bonchio, S. Campagna and F. Scandola, Chem. Soc. Rev., 2013, 42, 2262–2280 RSC.
- Y. C. Ji, L. J. Huang, J. Hu, C. Streb and Y.-F. Song, Energy Environ. Sci., 2015, 8, 776–789 CAS.
- J. Lehmann, A. Gaita-Arino, E. Coronado and D. Loss, Nat. Nanotechnol., 2007, 2, 312–317 CrossRef CAS PubMed.
- D. L. Long, R. Tsunashima and L. Cronin, Angew. Chem., Int. Ed., 2010, 49, 1736–1758 CrossRef CAS PubMed.
- Y. Leng, J. Wang, D. Zhu, X. Ren, H. Ge and L. Shen, Angew. Chem., 2009, 121, 174–177 CrossRef.
-
New and Future Developments in Catalysis: Hybrid materials, Composites and Organocatalysts, ed. S. Suib, Elsevier, Amsterdam, 2013 Search PubMed.
- S. Favette, B. Hasenknopf, J. Vaissermann, P. Gouzerh and C. Roux, Chem. Commun., 2003, 2664–2665 RSC.
- X. Tong and V. Thangadurai, J. Phys. Chem. C, 2015, 119, 7621–7630 CAS.
- R. Tsunashima, C. Richmond and L. Cronin, Chem. Sci., 2012, 3, 343 RSC.
- P. He, B. Xu, H. Liu, S. He, F. Saleem and X. Wang, Sci. Rep., 2013, 3, 1833 Search PubMed.
- J. Livage, Coord. Chem. Rev., 1998, 178–180(P), 999–1018 CrossRef CAS.
- J. Livage, Chem. Mater., 1991, 3, 578–593 CrossRef CAS.
- O. Dvorak and M. K. De Armond, Chem. Mater., 1992, 4, 1074–1077 CrossRef CAS.
- K. Zilberberg, S. Trost, J. Meyer, A. Kahn, A. Behrendt, D. Lützenkirchen-Hecht, R. Frahm and T. Riedl, Adv. Funct. Mater., 2011, 21, 4776–4783 CrossRef CAS.
- J. Liu, X. Wang, Q. Peng and Y. Li, Adv. Mater., 2005, 17, 764–767 CrossRef CAS.
- V. Glezer and O. Lev, J. Am. Chem. Soc., 1993, 115, 2533–2534 CrossRef CAS.
- G. T. Chandrappa, N. Steunou and J. Livage, Nature, 2002, 416, 702 CrossRef CAS PubMed.
- O. Durupthy, M. Jaber, N. Steunou, J. Maquet, G. T. Chandrappa and J. Livage, Chem. Mater., 2005, 17, 6395–6402 CrossRef CAS.
- N. A. Chernova, M. Roppolo, A. C. Dillon and M. S. Whittingham, J. Mater. Chem., 2009, 19, 2526–2552 RSC.
- G. Li, S. Pang, L. Jiang, Z. Guo and Z. Zhang, J. Phys. Chem. B, 2006, 110, 9383–9386 CrossRef CAS PubMed.
- A. Seliverstov and C. Streb, Chem. – Eur. J., 2014, 20, 9733–9738 CrossRef CAS PubMed.
- A. Seliverstov and C. Streb, Chem. Commun., 2014, 50, 1827–1829 RSC.
- Y. Zhang, N. Zhou, S. Akella, Y. Kuang, D. Kim, A. Schwartz, M. Bezpalko, B. M. Foxman, S. Fraden, I. R. Epstein and B. Xu, Angew. Chem., Int. Ed., 2013, 52, 11494–11498 CrossRef CAS PubMed.
- J. Tucher, L. C. Nye, I. Ivanovic-Burmazovic, A. Notarnicola and C. Streb, Chem. – Eur. J., 2012, 18, 10949–10953 CrossRef CAS PubMed.
- J. Forster, B. Rösner, R. H. Fink, L. C. Nye, I. Ivanovic-Burmazovic, K. Kastner, J. Tucher and C. Streb, Chem. Sci., 2013, 4, 418–424 RSC.
- K. Kastner, J. T. Margraf, T. Clark and C. Streb, Chem. – Eur. J., 2014, 20, 12269–12273 CrossRef CAS PubMed.
- D. Rehder, Bull. Magn. Reson., 1982, 4, 33–83 CAS.
- C. Streb, R. Tsunashima, D. A. MacLaren, T. McGlone, T. Akutagawa, T. Nakamura, A. Scandurra, B. Pignataro, N. Gadegaard and L. Cronin, Angew. Chem., Int. Ed., 2009, 48, 6490–6493 CrossRef CAS PubMed.
- M. Dave and C. Streb, Dalton Trans., 2015, 44, 18919–18922 RSC.
Footnotes |
† Electronic supplementary information (ESI) available: Synthetic and analytical details. See DOI: 10.1039/c6qi00457a |
‡ The gels employed for the application studies were prepared as follows: to a solution of (nBu4N)3[H3V10O28] (0.06 mmol) and CeCl3·7H2O (0.11 mmol) in DMSO (5 ml) was added a methanolic solution of TBAOH (1.5 M, 0.3 ml) at 80 °C. The solution was stirred for 5 min and H3PO4 (9 M, 0.45 ml) was added, resulting in the instantaneous formation of the gel which could be mechanically removed from the reaction vessel and cut into the desired shape using a scalpel. |
|
This journal is © the Partner Organisations 2017 |