DOI:
10.1039/C7PY00091J
(Paper)
Polym. Chem., 2017,
8, 2773-2784
Triple-stimuli-responsive ferrocene-containing homopolymers by RAFT polymerization†
Received
17th January 2017
, Accepted 5th April 2017
First published on 6th April 2017
Abstract
Well-defined ferrocene-containing homopolymers were synthesized by RAFT homopolymerization of an acrylate monomer bearing a ferrocene (Fc) unit and an N,N-diethylamino ethyl (DEAE) group, i.e. 2-(3-(N-(2-(diethylamino)ethyl)-acrylamido)propanoyloxy)ethyl ferrocenecarboxylate (Fc-DEAE-AM). As an ideal redox-responsive group, hydrophobic Fc can be easily oxidized into hydrophilic Fc+ by certain oxidants, providing tremendous opportunities to produce various Fc-containing redox-responsive materials. On the other hand, the pH/CO2-responsive reversible nature of the DEAE group makes it possible to construct a smart system to adapt to the complex environment in practical application. The stimuli-responsive aggregation behavior of the well-defined poly(Fc-DEAE-AM) homopolymer is examined by the combination of a fluorescent probe, UV/vis transmittance, zeta potential, transmission electron microscopy (TEM), and dynamic light scattering (DLS). In fact, due to the redox and pH/CO2-responsive character provided by the Fc and DEAE groups, the poly(Fc-DEAE-AM) homopolymer exhibits distinct phase transition in aqueous solution. In addition, such a homopolymer could form typical spherical particles in acid aqueous solution, and the redox agent could lead to changes in the size and morphology of the aggregates. Thus, we provide a new and efficient way to prepare triple-stimuli-responsive Fc-containing homopolymers, which might be used as interesting building block for the fabrication of multiple stimuli-responsive functional materials.
Introduction
Polymeric stimuli-responsive materials have attracted significant attention in many areas of chemistry, as these intelligent polymers with sharp polarity change upon stimulation show impressive potential for a variety of interesting applications.1–6 Most related research focused on smart polymer-based materials using just a single stimulus. However, the practical applications of stimuli-responsive polymers are usually in complex environments. In some cases, multiple stimuli are particularly appealing for the fabrication of smart materials with much more precise and sensitive stimuli-responsiveness.7,8
As redox reactions are widely and constantly present in the body metabolism, redox-responsive polymers have recently gained considerable attention because of their potential in controllable transport and release in physiological environments.9–20 As is well-known, redox-responsive polymers mainly contain sulfur,9 selenium,10,11 ferrocene (Fc),12–23 and other functionalities. Compared with sulfur and selenium, the hydrophobic Fc group can be quickly oxidized to yield the hydrophilic ferrocenium cation (Fc+), which can be reversibly recovered by a reductant. The reversible change in hydrophilicity with a rapid response is realized by only a gain and loss of electrons without a change in molecular structure, which is especially of interest to scientists for developing new redox-responsive polymers.12–14 For example, Zhang et al. have discovered a kind of multi-compartment nanoassembly via a poly(2-(dimethylamino)ethyl methacrylate)-block-poly(benzyl methacrylate)-block-poly(4-vinylbenzyl ferrocenecarboxylate) (PDMAEMA-b-PBzMA-b-PVFC) triblock copolymer prepared by seeded RAFT polymerization.14 The multi-compartment vesicles could be expected to be smart hosts to load and release guests, as the membrane pores could be reversibly on–off switched through redox triggering. Gallei et al. synthesized a diblock copolymer consisting of poly(vinylferrocene) and poly(N,N-diethylacrylamide) via a combination of anionic and RAFT polymerization, which showed both thermo- and redox-responsive character.20 Wurm et al. prepared poly(ferrocenyl glycidyl ether)-co-poly(ethylene glycol) (PfcGE-co-PEG) random copolymers by anionic ring-opening polymerization of a ferrocene-containing epoxide monomer of ferrocenyl glycidyl ether with ethylene oxide, and this kind of copolymer also exhibited both thermo- and redox-responsiveness.22 After introducing amine functionalities into PfcGE-co-PEG by a post-polymerization functionalization strategy, the obtained copolymer was able to demonstrate temperature, pH and redox triple-stimuli-responsiveness both in solution and on the surface.23 Although chain extension and post-polymerization modification strategies could be employed for the preparation of Fc-based copolymers with multiple stimuli-responsiveness, the tedious purification process of the chain extension strategy and the ill-defined distribution of functional groups along the backbone of the final polymers prepared by the post-polymerization approach would be the obstacles for preparation of well-defined Fc-based multiple stimuli-responsive polymers.
Recently, our group developed an efficient synthetic route for the preparation of multi-responsive homopolymers functionalized with different reactive groups.24–26 Motivated by the excellent redox-responsive abilities attributed to Fc-containing polymers, herein, we attempted to incorporate redox responsiveness into a new dual-responsive homopolymer so as to realize the triple-trigger-controlled dynamic association and dissociation of the polymer chain. Owing to the key step of the aza-Michael addition reaction, the Fc unit and the N,N-diethylamino ethyl (DEAE) group with both pH and CO2 responsiveness27–31 could be easily incorporated into one single monomer (Scheme 1), providing a new acrylate monomer, 2-(3-(N-(2-(diethylamino)ethyl)acrylamido)propanoyloxy)ethyl ferrocenecarboxylate (Fc-DEAE-AM). Subsequently, RAFT homopolymerization of Fc-DEAE-AM was utilized to afford the target well-defined homopolymer with controlled molecular weight and narrow molecular weight distribution. It was observed that variation in acid/alkali reagents, bubbling N2/CO2, and redox reagents could all induce reversible phase transition in aqueous solution. To the best of our knowledge, pH/CO2- and redox-responsive homopolymers have not been reported yet, which is crucial to exploit new trigger modes as close to physiological conditions as possible. The presented triple-stimuli-responsive system has many advantages such as facile sample preparation and the possibility to produce multifunctional materials, which may bring great opportunities to enlarge the potential applications of this class of homopolymers.
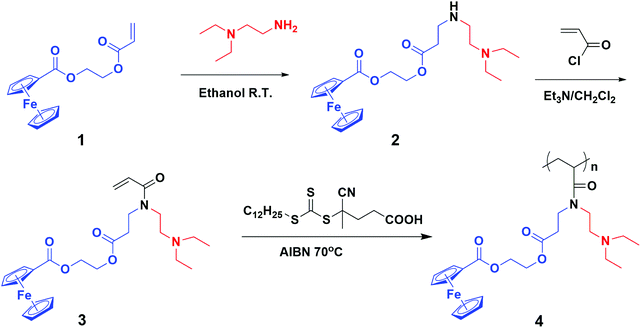 |
| Scheme 1 Synthetic route of poly(2-(3-(N-(2-(diethylamino)ethyl)acrylamido)-propanoyloxy)ethyl ferrocenecarboxylate) homopolymer. | |
Experimental
Materials
2,2′-Azobis(isobutyronitrile) (AIBN, Aldrich, 98%) was recrystallized twice from anhydrous ethanol. Toluene and dichloromethane were dried over CaH2 and distilled under reduced pressure prior to use. Triethylamine (Et3N, Aldrich, 99.5%) was dried over KOH and distilled over CaH2 under N2 prior to use. N-Phenyl-1-naphthylamine (PNA, Alfa Aesar, 97%) was purified by recrystallization in ethanol three times. Ferrocenecarboxylic acid (TCI, 98%), oxalyl chloride (Sinopharm, 98%), N,N-diethylethylenediamine (J&K, 99%), 2-hydroxyethyl acrylate (HEA, Aldrich, 97%), and acryloyl chloride (Aldrich, 97%) were used as received. 4-Cyano-4-(dodecylsulfanylthiocarbonylsulfanyl)pentanoic acid was synthesized according to previous literature.32
Measurements
All 1H and 13C NMR analyses were performed on a JEOL resonance ECZ 400S (400 MHz) in CDCl3 and D2O, tetramethylsilicone (1H NMR) and CDCl3 (13C NMR) were used as internal standards. FT-IR spectra were recorded on a Nicolet AVATAR-360 spectrophotometer with a 4 cm−1 resolution. Electrospray ionization mass spectrometry (ESI-MS) and high resolution mass spectrometry (HR-MS) were performed by using an Agilent LC/MSD SL system and a Thermo Fisher Scientific LTQ FT Ultra system, respectively. Relative molecular weights and molecular weight distributions were measured by a conventional gel permeation chromatography (GPC) system equipped with a Waters 515 Isocratic HPLC pump, a Waters 2414 refractive index detector, and a set of Waters Styragel columns (HR3 (500–30
000), HR4 (5000–600
000), and HR5 (50
000–4
000
000), 7.8 × 300 mm, particle size: 5 μm). GPC measurements were carried out at 35 °C using THF as the eluent with a flow rate of 1.0 mL min−1. The system was calibrated with linear poly(methyl methacrylate) standards. The phase transition of the homopolymer was measured by UV/vis spectroscopy using a Hitachi U-2910 spectrophotometer. Transmission electron spectroscopy (TEM) images were obtained by a JEOL JEM-1230 instrument operated at 80 kV. Zeta-potential was determined in KCl aqueous media (5.0 mM) at 25 °C by a Malvern Nano-ZS90 Zetasizer. The hydrodynamic diameter (Dh) was measured using dynamic light scattering (DLS), with a Malvern Nano-ZS90 Zetasizer. The samples were allowed to equilibrate for 2 min at 25 °C prior to measurement. Steady-state fluorescence spectra were measured at 20 °C on a Hitachi F-2700 fluorescence spectrophotometer with a bandwidth of 5 nm for excitation and emission, and the emission intensity at 418 nm was recorded to determine the critical aggregation concentration (CAC), where the excitation wavelength (λex) was 340 nm.
Preparation of ferrocenecarbonyl chloride
Oxalyl chloride (50 mL) was added dropwise to a solution of ferrocene carboxylic acid (23.0 g, 0.1 mol) in anhydrous CH2Cl2 (300 mL) at 0 °C. The reaction mixture was stirred at 0 °C for 30 min and then at room temperature for another 6 h. Both the solvent and excess oxalyl chloride were removed by distillation under reduced pressure. The resulting mixture was triturated with hot n-hexane followed by filtration, and the filtrate was concentrated to give 20.6 g of red crystal with a yield of 82.9%.
Preparation of 2-acryloxyethyl ferrocenecarboxylate
Ferrocenecarbonyl chloride (20.6 g, 82.9 mmol) was dissolved in 250 mL of anhydrous CH2Cl2, and the solution was cooled to 0 °C by using an ice bath. After mixing HEA (11.55 g, 99.48 mmol), Et3N (23.1 mL, 165.8 mmol), and anhydrous CH2Cl2 (50 mL) in the constant pressure liquid funnel, the mixture was added dropwise to the stirring solution within 10 min. The reactor was slowly warmed up to room temperature. After 12 h, the precipitated salt was removed by filtration. The filtrate was evaporated under reduced pressure, and the residue was purified by flash column chromatography on a silica gel column eluting with EtOAc/hexane (v
:
v = 1
:
8), to give 25.3 g (93.0%) of orange crystal, 2-acryloxyethyl ferrocenecarboxylate 1. 1H NMR (CDCl3): δ (ppm): 4.19 (5H, C5H5), 4.40 (2H, CCH2C2H2), 4.47 (4H, OCH2CH2O), 4.81 (2H, CCH2C2H2), 5.86, 6.17, 6.50 (3H, CH
CH2). 13C NMR (CDCl3): δ (ppm): 61.87 (OCH2CH2O), 62.47 (OCH2CH2O), 69.82 (C5H5), 70.22, 70.53, 71.48 (C5H4), 128.00 (CH
CH2), 131.45 (CH
CH2), 165.91 (OCOCH
CH2), 171.52 (OCOC5H4). FT-IR: ν (cm−1): 3090, 2954, 2924, 2852, 1712, 1635, 1458, 1407, 1376, 1272, 1185, 1132, 982, 930, 806, 769, 430, 413. DART-MS: calculated C16H16O454Fe for 326.0439; found 326.0437.
Preparation of 2-(3-(2-(diethylamino)ethylamino)propanoyloxy)ethyl ferrocenecarboxylate
2-Acryloxyethyl ferrocenecarboxylate 1 (20.5 g, 62.5 mmol) was dissolved in 300 mL of anhydrous EtOH. While stirring vigorously, N,N-diethylethylenediamine (60 mmol, 6.973 g) was added and then the mixture was stirred for 12 h at ambient temperature. The volatiles were evaporated under reduced pressure, and the residue was purified by flash chromatography on a silica gel column, eluting with EtOAc/hexane (v
:
v = 1
:
1, with Et3N), to give 10.4 g (37.5%) of sticky orange liquid, 2-(3-(2-(diethylamino)-ethylamino)propanoyloxy)ethyl ferrocenecarboxylate 2. 1H NMR (CDCl3): δ (ppm): 1.00 (6H, N(CH2CH3)2), 2.15 (1H, NH), 2.56 (8H, COCH2CH2 and CH2N(CH2CH3)2), 2.68 (2H, NHCH2CH2N), 2.93 (2H, COCH2CH2NH), 4.21 (5H, C5H5), 4.41 (6H, CCH2C2H2, OCH2CH2O), 4.81 (2H, CCH2C2H2). 13C NMR (CDCl3): δ (ppm): 11.18 (N(CH2CH3)2), 29.67 (COCH2CH2NH), 34.11 (NHCH2CH2N), 44.84 (COCH2CH2NH), 46.93 (N(CH2CH3)2), 71.50 (NHCH2CH2N), 61.78 (OCH2CH2O), 62.59 (OCH2CH2O), 69.81 (C5H5), 70.20, 70.44, 71.50 (C5H4), 172.39 (OCOC2H4), 171.54 (OCOC5H4). FT-IR: ν (cm−1): 3320, 3090, 2964, 2926, 2816, 1738, 1717, 1461, 1377, 1276, 1182, 1139, 1063, 1025, 821, 772, 484, 458, 412. DART-MS: calculated C22H33N2O454Fe for 443.1836; found 443.1827 [M + H]+.
Preparation of 2-(3-(N-(2-(diethylamino)ethyl)acrylamido)propanoyloxy)ethyl ferrocenecarboxylate
2-(3-(2-(Diethylamino)ethylamino)propanoyloxy)ethyl ferrocenecarboxylate 2 (8.3 g, 18.7 mmol) and Et3N (6.3 mL, 44.8 mmol) were dissolved in 150 mL of anhydrous CH2Cl2. The solution was cooled to 0 °C followed by addition of acryloyl chloride (2.0 g, 22.4 mmol) dropwise within 15 min. The mixture was slowly warmed up to room temperature and stirred at room temperature for 12 h. The volatiles were evaporated under reduced pressure, and the residue was purified by flash chromatography on a silica gel column eluting with EtOAc/hexane (v
:
v = 1
:
5, with Et3N), to give 3.7 g (39.7%) of sticky orange liquid, 2-(3-(N-(2-(diethylamino)ethyl)acrylamido)-propanoyloxy)ethyl ferrocenecarboxylate (Fc-DEAE-AM) 3. 1H NMR (CDCl3): δ (ppm): 0.95 (6H, N(CH2CH3)2), 2.51 (8H, COCH2CH2 and CH2N(CH2CH3)2), 3.40 (2H, CONCH2CH2N), 3.66 (2H, COCH2CH2NCO), 4.13 (5H, C5H5), 4.34 (6H, CCH2C2H2, OCH2CH2O), 4.74 (2H, CCH2C2H2), 5.61, 6.29, 6.46 (3H, CH
CH2). 13C NMR (CDCl3): δ (ppm): 11.92 (N(CH2CH3)2), 32.62 (COCH2CH2NCO), 34.17 (CONCH2CH2N), 43.57 (COCH2CH2NCO), 47.39 (N(CH2CH3)2), 52.42 (CONCH2CH2N), 61.74 (OCH2CH2O), 62.54 (OCH2CH2O), 69.76 (C5H5), 70.15, 70.43, 71.42 (C5H4), 127.52 (CH
CH2), 127.89 (CH
CH2), 166.33 (NCO), 170.75 (OCOC2H4), 171.89 (OCOC5H4). FT-IR: ν (cm−1): 3090, 2962, 2926, 1737, 1716, 1650, 1613, 1460, 1376, 1274, 1136, 1068, 1025, 822, 794, 772, 438, 421. DART-MS: calculated C25H35N2O554Fe for 497.1942; found 497.1930 [M + H]+.
RAFT homopolymerization of Fc-DEAE-AM
In a typical procedure, Fc-DEAE-AM 3 (0.58 g, 1.16 mmol), AIBN (2.8 mg, 0.017 mmol), and 4-cyano-4-(dodecylsulfanylthiocarbonylsulfanyl)pentanoic acid (21.0 mg, 0.052 mmol) were first added to a 25 mL Schlenk flask (flame-dried under vacuum prior to use) sealed with a rubber septum for degassing and kept under N2. Next, anhydrous toluene (0.6 mL) was charged via a gastight syringe. The flask was degassed by three cycles of freezing–pumping–thawing followed by immersing the flask into an oil bath set at 70 °C. The polymerization lasted 19 h and it was terminated by placing the flask into liquid N2. The reaction mixture was precipitated into cold n-hexane. The crude product was purified by repeated dissolution in THF and precipitation in cold n-hexane followed by drying in vacuo overnight to give a yellow oil of poly(Fc-DEAE-AM) 4a homopolymer. GPC: Mn = 3700 g mol−1, Mw/Mn = 1.21. 1H NMR (CDCl3): δ (ppm): 0.98 (6H, N(CH2CH3)2), 1.26 (18H, CH3C9H18C2H4), 1.73 (2H, CHCOCH2CHCO), 2.33 (H, CH2CHCO), 2.49–2.65 (8H, COCH2CH2, CH2N(CH2CH3)2), 3.33 (2H, CONCH2CH2N), 3.59 (2H, COCH2CH2CON), 4.19 (5H, C5H5), 4.39 (6H, CCH2C2H2, OCH2CH2O), 4.80 (2H, CCH2C2H2). FT-IR: ν (cm−1): 3090, 2962, 2926, 1740, 1716, 1641, 1460, 1377, 1275, 1138, 1060, 1020, 825, 773, 486, 459.
Micellar morphology
A THF solution of a poly(Fc-DEAE-AM) 4 homopolymer (10.0 mg mL−1) was added dropwise to acidic water under vigorous stirring and bubbling of N2 until the concentration of the homopolymer reached 0.5 mg mL−1. THF was evaporated by moderate stirring overnight at room temperature. For TEM studies, 5 μL of micelle solution was deposited on to an electron microscopy copper grid coated with carbon film, and the water was evaporated at room temperature.
Titration experiment
A 50 mL three-neck round-bottom flask equipped with Thermo Scientific™ Orion™ ROSS Ultra™ Refillable pH/ATC Triode™ Combination Electrodes and a Lei-Ci DJS-1C Conductance Electrode was used to conduct the potentiometric titration. 20 mL of poly(Fc-DEAE-AM) 4 homopolymer (2.0 mg mL−1) in aqueous salt solution ([NaCl] = 10 mM, pH = 2.84) was titrated by standard NaOH solution (1.0 M) under constant stirring and bubbling of N2.
Transmittance experiment
A poly(Fc-DEAE-AM) 4 homopolymer was uniformly dispersed in re-distilled water ultrasonically until the concentration of the homopolymer reached 2.0 mg mL−1. The gas-based transmittance changes of the aqueous solution were measured by a Hitachi U-2910 spectrophotometer at a constant wavelength of 800 nm.
Determination of critical aggregation concentration
PNA was used as a fluorescent probe to measure the critical aggregation concentration (CAC) of a poly(Fc-DEAE-AM) 4 homopolymer in aqueous media. An acetone solution of PNA ([PNA] = 2 mM) was added to a large amount of water until the concentration of PNA reached 0.002 mM. The solutions for fluorescence measurement were obtained by adding different amounts of THF solutions of poly(Fc-DEAE-AM) 4 homopolymer (1, 0.1, 0.01, 0.001, or 0.0001 mg mL−1) to water containing PNA ([PNA] = 0.002 mM). THF was evaporated by constant stirring and bubbling of N2 overnight at room temperature. The solution was sealed in closed vials before measurement to avoid the influence of CO2 in the air.
Results and discussion
Design and synthesis of Fc-DEAE-AM trifunctional acrylate monomers
Given the well-studied redox-responsive property of ferrocene12–23 and the pH/CO2-responsive behavior of PDEAEMA,27–31 we attempted to incorporate the redox-responsive Fc unit and the pH/CO2-sensitive DEAE group into a single monomer, and utilize RAFT polymerization to construct triple-stimuli-responsive homopolymers. The key in the preparation of the target monomer is to incorporate a polymerizable group, ferrocene unit, and DEAE group within a single monomer. Our group has developed an effective strategy to connect two different groups and an acrylamide group.24–26 Inspired by our previous results, we used a similar strategy in the present work. We employed the aza-Michael reaction as a key way to produce the secondary amine target intermediate which can be utilized in a further reaction to afford a polymerizable acrylamide.
The 2-acryloxyethyl ferrocenecarboxylate (AEFC) 1 precursor was first prepared using commercially available ferrocenecarboxylic acid, oxalyl chloride, and 2-hydroxyethyl acrylate as starting materials (Scheme S1†). Fig. S1A and S1B† show the 1H and 13C NMR spectra of the AEFC 1 intermediate, exhibiting the expected proton resonance signals of the target compound. The key intermediate of 2-(3-(2-(diethylamino)ethylamino)propanoyloxy)ethyl ferrocenecarboxylate (Fc-DEAE) 2 was then prepared through aza-Michael addition reaction using AEFC 1 and N,N-diethylethylenediamine. 1H (Fig. S2A†) and 13C (Fig. S2B†) NMR spectra of Fc-DEAE 2 confirmed the chemical structure of the target intermediate. Subsequently, Fc-DEAE 2 was treated with acryloyl chloride to afford the target Fc-DEAE-AM 3 monomer. The Fc-DEAE-AM 3 monomer was characterized by 1H NMR, 13C NMR, FT-IR, and MS. Fig. 1A shows the 1H NMR spectrum of the monomer, which displays the typical proton resonance signals of the double bond at 6.52, 6.28, and 5.61 ppm (peaks “a”, “b”, and “c”), the Fc moiety at 4.75, 4.34, and 4.14 ppm (peaks “l”, “m”, and “n”), and the DEAE group at 0.95 ppm (peak “g”). The 13C NMR spectrum shown in Fig. 1B demonstrates the anticipated carbon resonance signals of carbonyl (peaks “m”, “j”, and “c”) and double bond (peaks “a” and “b”). The structure of the Fc-DEAE-AM 3 monomer is also confirmed by FT-IR spectroscopy (Fig. S3†). In addition, the ESI-MS (Fig. S4†) and HR-MS (497.1930, Fig. S5†) results are in good agreement with the theoretical value (C25H35N2O554Fe+, 497.1942). All these results clearly confirm the structure of the target monomer 3.
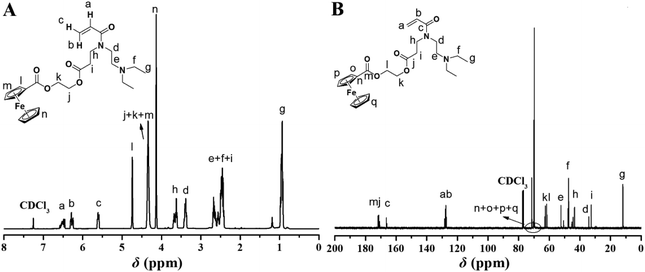 |
| Fig. 1
1H (A) and 13C (B) NMR spectra of Fc-DEAE-AM 3 monomer in CDCl3. | |
Synthesis of poly(Fc-DEAE-AM) homopolymers
RAFT polymerization32–37 is one of the most powerful and versatile reversible-deactivation radical polymerization (RDRP) processes, which enables precise control over molecular weight and molecular weight distribution. RAFT polymerization can be conducted under mild reaction conditions and is tolerant of most functional groups. In particular, RAFT polymerization of the acrylate monomer has been extensively investigated, and 4-cyano-4-(dodecylsulfanylthiocarbonylsulfanyl)-pentanoic acid (CDSTSP) has been frequently selected as the chain transfer agent (CTA) in recent decades.32 Considering the good solubility of the Fc moiety, toluene was employed as the solvent for RAFT polymerization of the Fc-DEAE-AM 3 monomer. Two well-defined poly(Fc-DEAE-AM) 4 homopolymers with narrow molecular weight distributions (Mw/Mn ≤ 1.22) were synthesized by varying the feeding ratio of Fc-DEAE-AM 3 monomer to CDSTSP as listed in Table 1.
Table 1 Synthesis of poly(Fc-DEAE-AM) homopolymer by RAFT polymerizationa
Entry |
[3] : [CTA] |
Time (h) |
Conv.b (%) |
M
n,theo c (g mol−1) |
M
n,NMR b (g mol−1) |
M
n,GPC d (g mol−1) |
M
w/Mn d |
[AIBN] : [CTA] = 1 : 3, solvent : toluene, polymerization temperature: 80 °C.
Obtained from 1H NMR.
M
n,theo = Mn,3 × ([3] : [CTA]) × Conv. + Mn,CTA.
Measured by GPC in THF at 35 °C.
|
4a
|
20 : 1 |
19 |
57.0 |
6100 |
6900 |
3700 |
1.21 |
4b
|
35 : 1 |
24 |
57.3 |
10 400 |
10 200 |
5300 |
1.22 |
The chemical structure of the poly(Fc-DEAE-AM) 4 homopolymer was examined using 1H NMR and FT-IR. A typical 1H NMR spectrum (Fig. 2A) shows the disappearance of double bond peaks and the continuing of the signals originating from the Fc moiety (peak “a”, “b”, and “c”) and the DEAE group (peak “k”). The RAFT mechanism was evidenced by the minor peak “l” located at 1.26 ppm corresponding to the protons of the –C12H25 moiety in CDSTSP. These observations distinctly illustrated the structure of the obtained poly(Fc-DEAE-AM) 4 homopolymer. Moreover, based on the integration area ratio of peak “a” to peak “l”, the absolute molecular weight of the poly(Fc-DEAE-AM) 4 homopolymer could be estimated using the following equation, in which Sa and Sl are the integration area of peak “a” and “l”, respectively, and Mmonomer and 403.67 are the molecular weights of the Fc-DEAE-AM 3 monomer and CDSTSP, respectively. Indeed, it can be seen from Table 1 that Mn,NMR is very close to the theoretical value (Mn,theo), which indicates a good control over molecular weight via RAFT polymerization.
| Mn,NMR = 9(Sa/Sl)Mmonomer + 403.67 | (1) |
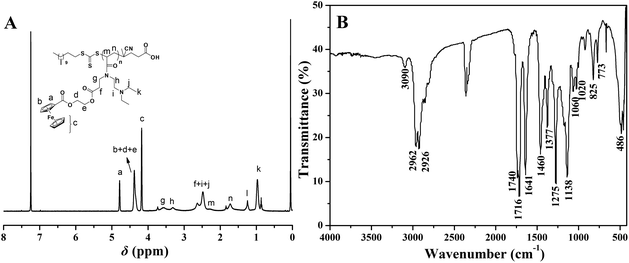 |
| Fig. 2
1H NMR (A) and FT-IR (B) spectra of poly(Fc-DEAE-AM) 4 homopolymer. | |
The FT-IR spectrum of the poly(Fc-DEAE-AM) 4 homopolymer is shown in Fig. 2B. The stretching vibrations of C–H in the ferrocene groups, C–H in-plane, and C–H bending vibrations appear at 3090 cm−1, 1020–1060 cm−1, and 773–825 cm−1, respectively. The significant peak at 486 cm−1 is attributed to the Fe–Cp stretching mode and the stretching vibrations of the ester and amide groups appear at 1716–1740 cm−1 and 1641 cm−1, respectively. All these results clearly confirm the chemical properties of the poly(Fc-DEAE-AM) 4 homopolymer.
The polymerization kinetics is further investigated and the linear dependence of ln([M]0/[M]t) on the time and molecular weight on the monomer conversion are observed in Fig. S6A and S6B,† respectively. These observations reflect the “living/controlled” nature of the RAFT polymerization of the Fc-DEAE-AM 3 monomer.
pH/CO2-Responsive behavior of poly(Fc-DEAE-AM) in aqueous media
Although PDEAEMA is a weak polybase and the pKa of its conjugated acid is about 7.3,38–42 the structural difference between PDEAEMA and poly(Fc-DEAE-AM) might result in different pH-stimulus responsiveness. Therefore, we firstly conducted a titration experiment by adding an NaOH aqueous solution (1.0 M) dropwise to the acidic solution of the poly(Fc-DEAE-AM) 4a homopolymer, for measuring the relationship between the protonation degree of the DEAE group and the pH of the aqueous solution.43 As shown in Fig. 3A, there are two transition points existing in the titration process (point ‘a’, pH ∼ 4.27 and point ‘b’, pH ∼ 8.15) according to the changing trends of the pH value and conductivity. Since the mobility of H+ (λH+ = 350 S cm2 mol−1) is much higher than that of Na+ (λNa+ = 50.5 S cm2 mol−1), the conductivity of the polymer-containing solution decreased in region 1 due to the decrease of the concentration of H+ though the concentration of Na+ increased. In region 2, the conductivity increased slowly because the initial portion of the NH+(C2H5)2 macro-ions were deprotonated. The rapid ascending of conductivity in region 3 indicated the increase in the concentration of Na+. As a result, region 2 from point ‘a’ to point ‘b’ corresponded to the deprotonation process of the NH+(C2H5)2 moiety. The pH value was plotted against the protonation degree (α) to further demonstrate the relationship between the dissociation of the polyelectrolyte and the changing of pH in the presence of salt (Fig. 3B). The magnitude of α was determined by the moles of titrated polyelectrolyte and the titrant added, where α ranged from 0 at the un-ionized state of the amine group (pH ∼ 8.15) to 1.0 at the fully protonated state (pH ∼ 4.27). On the other hand, the zeta-potential of the aggregates formed by the poly(Fc-DEAE-AM) 4a homopolymer increased from 38.2 mV to 45.4 mV after the pH was adjusted from 9.0 to 3.0, which indicated that the DEAE group was protonated to the NH+(C2H5)2 group with a positive charge.
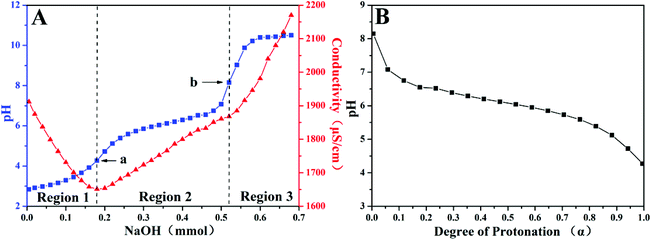 |
| Fig. 3 (A) Titration curves of poly(Fc-DEAE-AM) 4a homopolymer (2.0 mg mL−1) at 25 °C in aqueous media with 10 mM NaCl; (B) profile of dependence of protonation on pH. | |
Either the immiscibility of one of the blocks in a given solvent or their mutual incompatibility is reported to be the driving force for the self-assembly of the block copolymer.44 Unlike the copolymer, the main driving force for the self-assembly of the homopolymer depends on intramolecular phase separation.45,46 When the pH of the solution is decreased to 3.0, the DEAE group would be in the protonation state (DEAE+) completely while the Fc in the reduction state is known to be a kind of hydrophobic moiety. As a result, the poly(Fc-DEAE-AM) homopolymer would be endowed with the amphiphilic property in acidic aqueous solution. This characteristic provides an opportunity to investigate self-assembly behavior in aqueous media.
The critical aggregation concentration (CAC) of the poly(Fc-DEAE-AM) 4a homopolymer in aqueous solution was determined by fluorescence spectroscopy using PNA as the fluorescent probe at three different pH values (3.0, 7.0, and 10.0) and a constant temperature of 25 °C. It was reported that the fluorescence intensity of PNA was sensitive to the environment and the polarity of its surroundings, and it could be very easily quenched by polar solvents such as water.47 Once the aggregates were formed in aqueous solution, the hydrophobic PNA could be encapsulated into the hydrophobic core so as to dramatically increase the value of I/I0 (I and I0 are the fluorescence intensities of PNA at 418 nm with and without poly(Fc-DEAE-AM) 4a homopolymer in the solution, respectively) of its emission spectrum.47 The fluorescence intensity of PNA at pH = 3.0, at which the amine groups are completely protonated, showed a clear increase when the concentration of homopolymer 4a exceeded a certain value (Fig. 4A). Thus, the CAC of the poly(Fc-DEAE-AM) 4a homopolymer was determined to be the intersection of two straight lines with a value of 1.95 × 10−5 g mL−1 as shown in Fig. 4B. At the same time, the fluorescence emission spectra of PNA in neutral (pH = 7.0) and basic (pH = 10.0) aqueous solutions of the poly(Fc-DEAE-AM) 4a homopolymer showed no obvious CAC with the increase of concentration of the homopolymer (Fig. S7†).
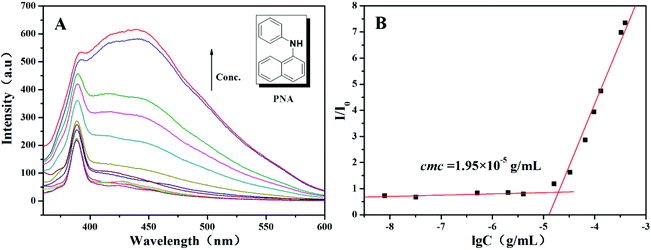 |
| Fig. 4 (A) Fluorescence emission spectra of PNA in aqueous solution of poly(Fc-DEAE-AM) 4a homopolymer ([PNA] = 2 × 10−6 mol L−1); (B) dependence of fluorescence intensity ratio of PNA emission band at 418 nm on the concentration of poly(Fc-DEAE-AM) 4a homopolymer at 25 °C in an acidic environment (pH = 3.0). | |
It is well known that the pH value of saturated CO2 aqueous solution is about 3.9 at ambient temperature and atmosphere pressure due to the chemical equilibrium with weak carbonic acid.48 Unlike commonly used acids for adjusting the pH of solutions, dissolved CO2 gas can be easily removed from the solution by bubbling of inert gas such as N2 or Ar. The titration experiment showed that the protonation of the DEAE moiety also has the expected pH responsiveness. In order to test if the homopolymer is able to exhibit CO2-responsive behavior in aqueous solution, we monitored the transmittance change of the poly(Fc-DEAE-AM) 4a homopolymer in aqueous solution at 25 °C by alternating bubbling with CO2/N2 (Fig. 5). It is found that the solution appears turbid when prepared by bubbling with N2 for 10 min and then sonication for 10 min at 25 °C with transmittance of 0.6%, whereas the solution turns clear immediately with a sharp increase in transmittance from 0.6% to 97.5% in 10 s after the treatment with CO2. Although the solution turns turbid again with the decrease in transmittance from 97.5% to 0.6% after the treatment with N2, it takes about 60 min for the decrease of transmittance from 97.5% to 0.6%. We speculate that the difference in response time might result from a higher affinity of the DEAE moieties toward CO2, in comparison with N2. In addition, we found that the zeta-potential increased from 38.2 mV to 45.5 mV after the treatment with CO2. The increase in zeta-potential also indicated the protonation of the tertiary amine group. Subsequently, after passing N2 through the solution to remove CO2, a sharp drop in transmittance was observed due to the deprotonation of the tertiary amine group. Repeatable cycles under an alternating CO2/N2 stimulation as shown in Fig. 5 indicated the gas-responsive reversibility of the poly(Fc-DEAE-AM) 4a homopolymer.
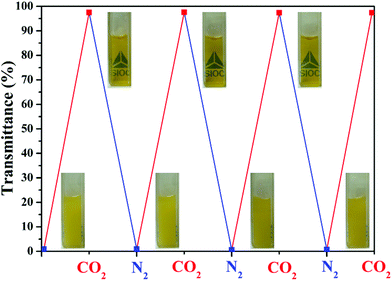 |
| Fig. 5 Optical appearances and transmittance changes of aqueous solution of poly(Fc-DEAE-AM) 4a homopolymer (2.0 mg mL−1) with CO2/N2 gas triggers. | |
Redox-responsive behavior of poly(Fc-DEAE-AM) in aqueous media
The inter-conversion between reduced and oxidized forms can lead to the transition from hydrophobicity to hydrophilicity of the hydrophobic part of Fc-containing polymers.12–23 We then examined the effect of oxidation on the self-assembly behavior of a poly(Fc-DEAE-AM) 4a homopolymer under both basic and acidic conditions (Fig. 6A). Upon treating the solution with an equivalent amount of FeCl3 oxidant, the transparency of the basic solution (pH = 9.0) was improved immediately (<10 s), accompanied by a distinct color change from orange to green, which is similar to the observations in a previous report.12 The change in color of the solution after treatment with FeCl3 might result from the formation of Fe2+ by the oxidation of Fc to Fc+ with Fe3+ on the basis of a previous report.12 After the addition of vitamin C (VC), the solution turned dark yellow immediately (<10 s) along with the formation of precipitate because all DEAE groups were not protonated in a basic environment according to the dependence of protonation on pH (Fig. 3B). One can notice that the color of the solution (dark yellow) after treatment with FeCl3 and VC is different from that of the original solution (yellow), though all of the Fc+ should be reduced to Fc after the treatment with VC according to previous reports.12–23 We speculate that the presence of additional Fe3+ and Fe2+ after the addition of FeCl3 and VC might lead to the difference between the color of the final solution and that of the original solution. Similarly, the color of the transparent acidic solution (pH = 3.0) also changed from orange to green upon the addition of FeCl3, and turned dark yellow with the addition of VC. The zeta potential of the aqueous solution of the poly(Fc-DEAE-AM) 4a homopolymer greatly increased from 38.2 mV to 49.7 mV after the FeCl3 was injected, which demonstrated that the Fc group was oxidized to Fc+. After the addition of VC, the zeta potential decreased to 39.5 mV, indicative of the reduction of Fc+ to Fc again. However, a stable colloidal solution was formed without the formation of any precipitate due to the presence of hydrophilic NH+(C2H5)2 moieties at pH = 3.0. To further probe the reversible redox behavior of the poly(Fc-DEAE-AM) 4a homopolymer, UV/vis spectra were recorded in acidic solution as shown in Fig. 6B. The original homopolymer gave rise to an absorbance at 446 nm, and upon the addition of FeCl3 (oxidant), the signal at 446 nm decreased while a new signal originating from the formation of Fc+ state was observed at 636 nm. The signal at 446 nm re-appeared after the reduction of the ferrocenium moiety by adding VC (reductant), which proved that this redox process is reversible.
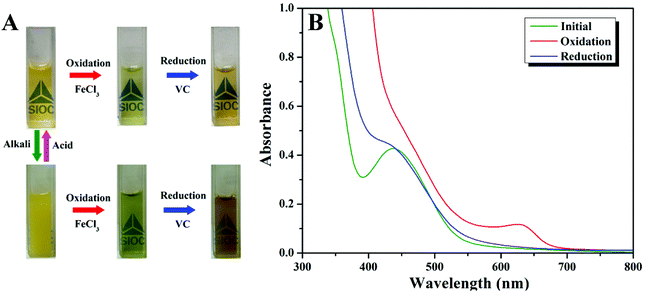 |
| Fig. 6 (A) Photographs of aqueous solution of poly(Fc-DEAE-AM) 4a homopolymer after treating with multi-stimuli; (B) UV/vis absorption spectra of acidic aqueous solution of poly(Fc-DEAE-AM) 4a homopolymer (2.0 mg mL−1) before and after the treatment of redox agent. | |
The TEM image (Fig. 7A) showed that initial spherical aggregates with hydrophobic Fc domains and hydrophilic protonated DEAE segments were formed when the pH of the solution was 3.0 with an average diameter of 219 nm measured by DLS (Fig. 8A). As an equivalent amount of oxidant (FeCl3) was added to the solution (1.1 mM), the size of aggregates in the solution decreased significantly to 160 nm (Fig. 7B), which was consistent with the result of DLS (162 nm) in Fig. 8A. It is worth noting that vesicles were formed after the addition of FeCl3 and one can clearly notice a dark rim in Fig. 7B, which was attributed to the membrane of the vesicle. After an equivalent amount of reductant (VC) was added (1.1 mM), the aggregates transformed into typical spheres with a size of 190 nm (Fig. 7C and 8A). One might argue that the addition of FeCl3 also led to the increase of the concentration of salt, which could affect the self-assembly behavior of amphiphilic block copolymer and micellar morphologies according to previous reports.49–51 In order to make this issue clear, we added different amounts of NaCl into the aqueous solution of the poly(Fc-DEAE-AM) 4a homopolymer instead of FeCl3. Noticeable spherical aggregates were observed from the TEM and DLS analysis as the concentration of NaCl was 1.7 and 17 mM, respectively, while large aggregates were formed as the concentration of NaCl increased to 170 mM. These observations indicated that the formation of vesicles after the addition of FeCl3 predominantly resulted from the formation of Fc+, which changed the hydrophilic–hydrophobic balance of the poly(Fc-DEAE-AM) homopolymer, although we cannot completely exclude the marginal effect of increasing the ion concentration.
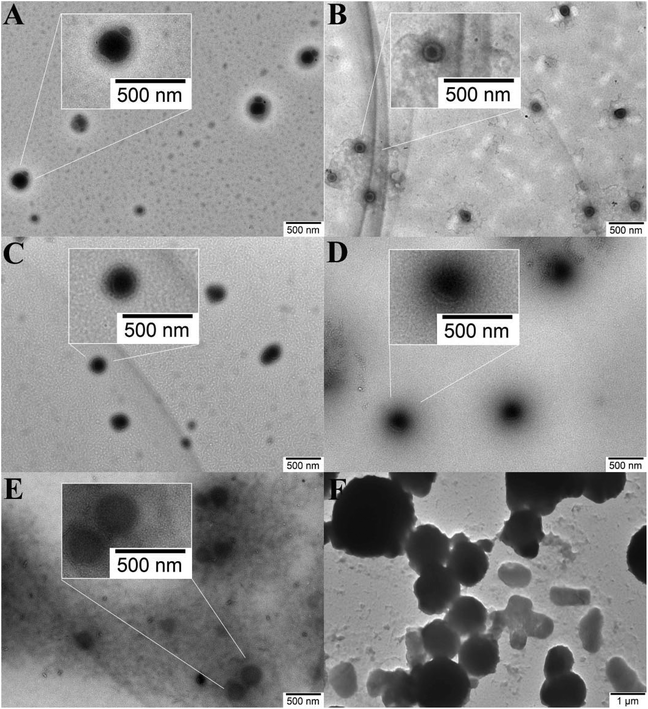 |
| Fig. 7 TEM images of aggregated formed by poly(Fc-DEAE-AM) 4a homopolymer at 25 °C in an acidic environment (0.5 mg mL−1 and pH = 3.0): before (A) and after (B) oxidation by FeCl3; reduction (C) by vitamin C; in 1.7 mM (D), 17 mM (E), and 170 mM (F) NaCl aqueous solution. | |
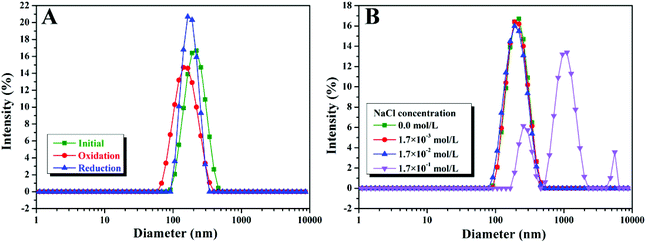 |
| Fig. 8 Size distributions of poly(Fc-DEAE-AM) 4a homopolymer at 25 °C in an acidic environment (pH = 3.0): aggregates (A) before and after treatment of redox agent; (B) at different concentrations of NaCl. | |
Conclusion
In summary, we synthesized a new type of acrylamide monomer by combining a pH/CO2-responsive DEAE moiety, a redox-responsive Fc group, and a polymerizable double bond into a single monomer via aza-Michael addition and amidation reaction. A well-defined triple-stimuli-responsive poly(Fc-DEAE-AM) homopolymer was then obtained by RAFT polymerization. The aggregation behavior of the homopolymer in aqueous media was investigated by the combination of a fluorescent probe, UV/vis transmittance, and zeta potential. It is found that the poly(Fc-DEAE-AM) homopolymer shows pH/CO2 and redox responsiveness as demonstrated by the phase transition in aqueous solution. In addition, TEM and DLS measurements indicate that such a homopolymer could form typical spherical particles in acidic aqueous solution, and a redox agent could result in changes in the size and morphology of the aggregates. Compared with the multi-stimuli responsive Fc-containing polymers reported before,20,22,23 we offer a new and efficient method for obtaining triple-stimuli-responsive Fc-containing homopolymers. The novel Fc-DEAE-AM monomer broadens the field of Fc-containing monomers and polymers toward polyacrylamide. The end CTA group in the poly(Fc-DEAE-AM) homopolymer might provide the opportunity for post-modification by introducing additional functional chains, which further broadens the potential application of these kinds of polymers.
Acknowledgements
The authors are grateful for the financial support from the National Basic Research Program of China (2015CB931900), National Natural Science Foundation of China (21474127), Strategic Priority Research Program of the Chinese Academy of Sciences (XDB20000000), Youth Innovation Promotion Association of Chinese Academy of Sciences (2016233), and Shanghai Scientific and Technological Innovation Project (16JC1402500, 14520720100, and 16520710300).
References
- T. Thambi, J. H. Park and D. S. Lee, Stimuli-Responsive Polymersomes for Cancer Therapy, Biomater. Sci., 2016, 4, 55–69 RSC.
- M. Wei, Y. Gao, X. Li and M. J. Serpe, Stimuli-Responsive Polymers and Their Applications, Polym. Chem., 2017, 8, 127–143 RSC.
- G. Kocak, C. Tuncer and V. Butun, pH-Responsive Polymers, Polym. Chem., 2017, 8, 144–176 RSC.
- E. Cabane, V. Malinova, S. Menon, C. G. Palivan and W. Meier, Photoresponsive Polymersomes as Smart, Triggerable Nanocarriers, Soft Matter, 2011, 7, 9167–9176 RSC.
- A. Topete, S. Barbosa and P. Taboada, Intelligent Micellar Polymeric Nanocarriers for Therapeutics and Diagnosis, J. Appl. Polym. Sci., 2015, 132, 42650 CrossRef.
- A. C. Hunter and S. M. Moghimi, Smart Polymers in Drug Delivery: A Biological Perspective, Polym. Chem., 2017, 8, 41–51 RSC.
- J. Zhuang, M. R. Gordon, J. Ventura, L. Li and S. Thayumanavan, Multi-Stimuli Responsive Macromolecules and Their Assemblies, Chem. Soc. Rev., 2013, 42, 7421–7435 RSC.
- S. Guragain, B. P. Bastakoti, V. Malgras, K. Nakashima and Y. Yamauchi, Multi- Stimuli-Responsive Polymeric Materials, Chem. – Eur. J., 2015, 21, 13164–13174 CrossRef CAS PubMed.
- A. Napoli, M. J. Boerakker, N. Tirelli, R. J. M. Nolte, N. A. J. M. Sommerdijk and J. A. Hubbell, Glucose-oxidase Based Self-Destructing Polymeric Vesicles, Langmuir, 2004, 20, 3487 CrossRef CAS PubMed.
- N. Ma, Y. Li, H. P. Xu, Z. Wang and X. Zhang, Dual Redox Responsive Assemblies Formed from Diselenide Block Copolymers, J. Am. Chem. Soc., 2010, 132, 442–443 CrossRef CAS PubMed.
- H. Ren, Y. Wu, N. Ma, H. Xu and X. Zhang, Side-chain Selenium-Containing Amphiphilic Block Copolymers: Redox-Controlled Self-Assembly and Disassembly, Soft Matter, 2012, 8, 1460–1466 RSC.
- B. Adhikari, R. Afrasiabi and H.-B. Kraatz, Ferrocene-Tryptophan Conjugate: An Example of a Redox-Controlled Reversible Supramolecular Nanofiber Network, Organometallics, 2013, 32, 5899–5905 CrossRef CAS.
- X. Chang, R. Dong, B. Ren, Z. Cheng, J. Peng and Z. Tong, Novel Ferrocenyl-Terminated Linear-Dendritic Amphiphilic Block Copolymers: Synthesis, Redox-Controlled Reversible Self-Assembly, and Oxidation-Controlled Release, Langmuir, 2014, 30, 8707–8716 CrossRef CAS PubMed.
- P. Shi, Y. Qu, C. Liu, H. Khan, P. Sun and W. Q. Zhang, Redox-Responsive Multicompartment Vesicles of Ferrocene-Containing Triblock Terpolymer Exhibiting On-Off Switchable Pores, ACS Macro Lett., 2016, 5, 88–93 CrossRef CAS.
- Q. Yan, J. Y. Yuan, Z. Cai, Y. Xin, Y. Kang and J. Y. Yin, Voltage-Responsive Vesicles Based on Orthogonal Assembly of Two Homopolymers, J. Am. Chem. Soc., 2010, 132, 9268–9270 CrossRef CAS PubMed.
- Q. Yan, A. Feng, H. Zhang, Y. Yin and J. Y. Yuan, Redox-Switchable Supramolecular Polymers for Responsive Self-Healing Nanofibers in Water, Polym. Chem., 2013, 4, 1216–1220 RSC.
- L. Peng, H. Zhang, A. Feng, M. Huo, Z. Wang, J. Hu, W. Gao and J. Y. Yuan, Electrochemical Redox Responsive Supramolecular Self-Healing Hydrogels Based on Host-Guest Interaction, Polym. Chem., 2015, 6, 3652–3659 RSC.
- X. Chang, Z. Cheng, B. Ren, R. Dong, J. Peng, S. Fu and Z. Tong, Voltage-Responsive Reversible Self-Assembly
and Controlled Drug Release of Ferrocene-Containing Polymeric Superamphiphiles, Soft Matter, 2015, 11, 7494–7501 RSC.
- R. L. N. Hailes, A. M. Oliver, J. Gwyther, G. R. Whittell and I. Manners, Polyferrocenylsilanes: Synthesis, Properties, and Applications, Chem. Soc. Rev., 2016, 45, 5358–5407 RSC.
- B. V. K. J. Schmidt, J. Elbert, C. Barner-Kowollik and M. Gallei, Individually Addressable Thermo- and Redox-Responsive Block Copolymers by Combining Anionic Polymerization and RAFT Protocols, Macromol. Rapid Commun., 2014, 35, 708–714 CrossRef CAS PubMed.
- A. Alkan and F. R. Wurm, Water-Soluble Metallocene-Containing Polymers, Macromol. Rapid Commun., 2016, 37, 1482–1493 CrossRef CAS PubMed.
- C. Tonhauser, A. Alkan, M. Schömer, C. Dingels, S. Ritz, V. Mailänder, H. Frey and F. R. Wurm, Ferrocenyl Glycidyl Ether: A Versatile Ferrocene Monomer for Copolymerization with Ethylene Oxide to Water-Soluble, Thermoresponsive Copolymers, Macromolecules, 2013, 46, 647–655 CrossRef CAS.
- A. Alkan, C. Steinmetz, K. Landfester and F. R. Wurm, Triple-Stimuli-Responsive Ferrocene-Containing PEGs in Water and on the Surface, ACS Appl. Mater. Interfaces, 2015, 7, 26137–26144 CAS.
- X. Jiang, C. Feng, G. L. Lu and X. Y. Huang, Thermoresponsive Homopolymer Tunable by pH and CO2, ACS Macro Lett., 2014, 3, 1121–1125 CrossRef CAS.
- X. Jiang, C. Feng, G. L. Lu and X. Y. Huang, Synthesis of Temperature and pH/CO2 Responsive Homopolymer Bearing Oligo(ethylene glycol) Unit and N,N-diethylamino Ethyl Group and Its Solution Property, Polymer, 2015, 64, 268–276 CrossRef CAS.
- X. Jiang, C. Feng, G. L. Lu and X. Y. Huang, Oxygen and Carbon Dioxide Dual Gas-Responsive Homopolymers and Diblock Copolymers Synthesized via RAFT Polymerization, Polym. Chem., 2017, 8, 1163–1176 RSC.
- P. G. Jessop, L. Kozycz, Z. G. Rahami, D. Schoenmakers, A. R. Boyd, D. Wechsler and A. M. Holland, Tertiary Amine Solvents Having Switchable Hydrophilicity, Green Chem., 2011, 13, 619–623 RSC.
- D. Han, X. Tong, O. Boissiere and Y. Zhao, General Strategy for Making CO2-Switchable Polymers, ACS Macro Lett., 2012, 1, 57–61 CrossRef CAS.
- D. Han, O. Boissiere, S. Kumar, X. Tong, L. Tremblay and Y. Zhao, Two-Way CO2-Switchable Triblock Copolymer Hydrogels, Macromolecules, 2012, 45, 7440–7445 CrossRef CAS.
- B. Yan, D. Han, O. Boissiere, P. Ayotte and Y. Zhao, Manipulation of Block Copolymer Vesicles Using CO2: Dissociation or “Breathing”, Soft Matter, 2013, 9, 2011–2016 RSC.
- Q. Yan and Y. Zhao, CO2-Stimulated Diversiform Deformations of Polymer Assemblies, J. Am. Chem. Soc., 2013, 135, 16300–16303 CrossRef CAS PubMed.
- K. Ponnusamy, R. P. Babu and R. Dhamodharan, Synthesis of Block and Graft Copolymers of Styrene by RAFT Polymerization, Using Dodecyl-Based Trithiocarbonates as Initiators and Chain Transfer Agents, J. Polym. Sci., Part A: Polym. Chem., 2013, 51, 1066–1078 CrossRef CAS.
- J. Chiefari, Y. K. Chong, F. Ercole, J. Krstina, J. Jeffery, P. T. Le Tam, R. Mayadunne, G. F. Meijs, C. L. Moad, G. Moad, E. Rizzardo and S. H. Thang, Living Free-Radical Polymerization by Reversible Addition-Fragmentation Chain Transfer:The RAFT Process, Macromolecules, 1998, 31, 5559–5562 CrossRef CAS.
- C. L. McCormick and A. B. Lowe, Aqueous RAFT Polymerization: Recent Developments in Synthesis of Functional Water-Soluble (Co)polymers with Controlled Structures, Acc. Chem. Res., 2004, 37, 312–325 CrossRef CAS PubMed.
- G. Moad, E. Rizzardo and S. H. Thang, Living Radical Polymerization
by the RAFT Process, Aust. J. Chem., 2005, 58, 379–410 CrossRef CAS.
- G. Moad, E. Rizzardo and S. H. Thang, Living Radical Polymerization by the RAFT Process-A First Update, Aust. J. Chem., 2006, 59, 669–692 CrossRef CAS.
- G. Moad, E. Rizzardo and S. H. Thang, Living Radical Polymerization by the RAFT Process-A Second Update, Aust. J. Chem., 2009, 62, 1402–1472 CrossRef CAS.
- A. S. Lee, A. P. Gast, V. Butun and S. P. Armes, Characterizing the Structure of pH Dependent Polyelectrolyte Block Copolymer Micelles, Macromolecules, 1999, 32, 4302–4310 CrossRef CAS.
- S. Y. Liu, N. C. Billingham and S. P. Armes, A Schizophrenic Water-Soluble Diblock Copolymer, Angew. Chem., Int. Ed., 2001, 40, 2328–2331 CrossRef CAS PubMed.
- E. J. Lobb, I. Ma, N. C. Billingham and S. P. Armes, Facile Synthesis of Well- Defined, Biocompatible Phosphorylcholine-Based Methacrylate Copolymers via Atom Transfer Radical Polymerization at 20 °C, J. Am. Chem. Soc., 2001, 123, 7913–7914 CrossRef CAS PubMed.
- H. Zhang, P. Ni, J. He and C. Liu, Novel Fluoroalkyl End-Capped Amphiphilic Diblock Copolymers with pH/Temperature Response and Self-Assembly Behavior, Langmuir, 2008, 24, 4647–4654 CrossRef CAS PubMed.
- X. Chen, D. P. Randall, C. Perruchot, J. F. Watts, T. E. Patten, T. Werne and S. P. Armes, Synthesis and Aqueous Solution Properties of Polyelectrolyte-Grafted Silica Particles Prepared by Surface-Initiated Atom Transfer Radical Polymerization, J. Colloid Interface Sci., 2003, 257, 56–64 CrossRef CAS PubMed.
- E. He, P. Ravi and K. C. Tam, Synthesis and Self-Assembly Behavior of Four-Arm Poly(ethylene oxide)-b-poly(2-(diethylamino)ethyl methacrylate) Star Block Copolymer in Salt Solutions, Langmuir, 2007, 23, 2382–2388 CrossRef CAS PubMed.
- T. S. Kale, A. Klaikherd, B. Popere and S. Thayumanavan, Supramolecular Assemblies of Amphiphilic Homopolymers, Langmuir, 2009, 25, 9660–9670 CrossRef CAS PubMed.
- S. Basu, D. R. Vutukuri, S. Shyamroy, B. S. Sandanaraj and S. Thayumanavan, Invertible Amphiphilic Homopolymers, J. Am. Chem. Soc., 2004, 126, 9890–9891 CrossRef CAS PubMed.
- Y. Zhu, L. Liu and J. Z. Du, Probing into Homopolymer Self-Assembly: How Does Hydrogen Bonding Influence Morphology, Macromolecules, 2013, 46, 194–203 CrossRef CAS.
- P. S. Xu, H. D. Tang, S. Y. Li, J. Ren, E. Van Kirk, W. J. Murdoch, M. Radosz and Y. Q. Shen, Enhanced Stability of Core-Surface Cross-Linked Micelles Fabricated from Amphiphilic Brush Copolymers, Biomacromolecules, 2004, 5, 1736–1744 CrossRef CAS PubMed.
- H. Liu, S. Lin, Y. Feng and P. Theato, CO2-Responsive Polymer Materials, Polym. Chem., 2017, 8, 12–23 RSC.
- R. J. Barlow, S. Zimmerman, K. Khoucaz and A. Eisenberg, Phase Separation of Micellar Solutions at Extreme Dilution: A Study of Polystyrene-6-poly(sodium Acrylate) Micelles in Aqueous NaCl Solutions, J. Polym. Sci., Part B: Polym. Phys., 1996, 34, 1197–1212 CrossRef CAS.
- L. Zhang and A. Eisenberg, Morphogenic Effect of Added Ions on Crew-Cut Aggregates of Polystyrene-b-poly(acrylic acid) Block Copolymers in Solutions, Macromolecules, 1996, 29, 8805–8815 CrossRef CAS.
- S. V. Solomatin, T. K. Bronich, T. W. Bargar, A. Eisenberg, V. A. Kabanov and A. V. Kabanov, Environmentally Responsive Nanoparticles from Block Ionomer Complexes: Effects of pH and Ionic Strength, Langmuir, 2003, 19, 8069–8076 CrossRef CAS.
Footnotes |
† Electronic supplementary information (ESI) available. See DOI: 10.1039/c7py00091j |
‡ Both authors contributed equally to this work. |
|
This journal is © The Royal Society of Chemistry 2017 |