DOI:
10.1039/C6NR07339E
(Paper)
Nanoscale, 2017,
9, 433-439
A temperature, pH and sugar triple-stimuli-responsive nanofluidic diode†
Received
17th September 2016
, Accepted 25th November 2016
First published on 25th November 2016
Abstract
In this article, we have demonstrated for the first time a triple stimuli-responsive nanofluidic diode that can rectify ionic current under multiple external stimuli including temperature, pH, and sugar. This diode was fabricated by immobilizing poly[2-(dimethylamino)ethyl methacrylate]-co-[4-vinyl phenylboronic acid] (P(DMAEMA-co-VPBA)) onto the wall of a single glass conical nanopore channel via surface-initiator atom transfer radical polymerization (SI-ATRP). The copolymer brushes contain functional groups sensitive to pH, temperature and sugar that can induce charge and configuration change to affect the status of the pore wall. The experimental results confirmed that the P(DMAEMA-co-VPBA) brush modified nanochannel regulated the ionic current rectification successfully under three different external stimuli. This biomimetically inspired research simulates the complex biological multi-functions of ion channels and promotes the development of “smart” biomimetic nanochannel systems for actuating and sensing applications.
1 Introduction
In living systems, a variety of biological nanochannels are present in the cell membrane, endoplasmic reticulum membrane and nuclear envelope that respond to external environment stimuli and play important roles in signaling, mass transfer transportation and nerve conduction.1–4 Inspired by these asymmetric structures and the responsive ion transport of biological ion channels, synthetic nanochannels involving various materials have been developed rapidly within various materials to simulate the functions of their biological counterparts.5–7 Compared with the biological ion channels, artificial nanochannels possess the advantages of controllable geometry and size, excellent mechanical robustness, and ease of functionalization convenience.8
Chemical modification of synthetic nanochannels is an important approach for developing intelligent systems that can respond to various external stimuli and thus rectify the ionic current. A large range of functional groups including natural macromolecules, biomacromolecules, polymers and small molecules has been integrated with synthetic nanochannels to achieve a series of smart bio-inspired nanochannels for mimicking the gating mechanisms of biological nanochannels, energy conversion systems, sensors, filters and others.9–11 Specific responses to changes in the external environment, such as pH,12–21 temperature,22,23 light,9,24–26 electric field,27–29 sugar,30–32 proteins33, gas34 and special ions,35–46 have been reported. In addition to the above single-stimulus-responsive nanochannels, biomimetic ion channels that can simultaneously respond to two types of stimuli have recently attracted considerable interest, including pH- and temperature-,47–49 pH- and light-,50–53 pH and molecule,54,55 and dual-ion-responsive56,57 nanochannels. Over the past decade, our group has been committed to building a biomimetic nanochannel system.7,30,47,58,59 However, these reported biomimetic ion channels were responsive to only one or two types of external stimuli; the efforts on extending the capacity of nanofluidic diodes to respond to multiple stimuli will benefit the applications of such nanodevices.
Here, for the first time, we demonstrated the integration of a copolymer poly[2-(dimethylamino)ethyl methacrylate]-co-[4-vinyl phenylboronic acid] (P(DMAEMA-co-VPBA)), which underwent pH-, temperature-, and sugar-induced conformational transitions, into a single glass conical nanochannel to achieve tri-stimuli based ion transport (Scheme 1). The copolymer P(DMAEMA-co-VPBA) was introduced into the surface of a single glass conical nanopore channel via atom transfer radical polymerization (ATRP). In an acidic environment, the tertiary amino groups of the PDMAEMA backbone are protonated,60 so that the interior surface of the nanochannel is positive. On the other hand, the phenylboronic acid groups of the PVBA backbone are ionized under basic conditions61 leading to a negatively charged nanochannel surface. The PDMAEMA backbone and PVBA backbones modified on the surface of the nanochannel compensate for each other so that the nanodevice rectifies the ion current in a broad range of pH values. Moreover, the P(DMAEMA-co-VPBA)-modified nanochannel can switch its conductivity between “low” and “high” ion current states by tuning the external temperature to change the effective nanochannel cross section due to the conformational change of the copolymer brushes with temperature. The ionic current of the P(DMAEMA-co-VPBA) modified nanochannel increased significantly when the temperature was higher than the lower critical solution temperature (LCST). Furthermore, the P(DMAEMA-co-VPBA) modified nanochannel showed an excellent sugar responsive capacity that could reversibly switch between the non-rectifying and rectifying states.
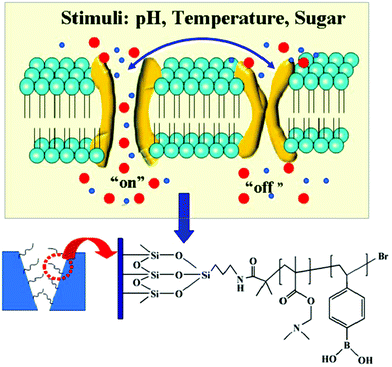 |
| Scheme 1 The schematic of the nanochannel responding to external stimuli. The thermo-, pH-, and sugar-responsive copolymer poly(N,N-dimethylamino ethyl methacrylate-co-4-vinyl benzene boric acid) (P(DMAEMA-co-VPBA)) was polymerized onto the interior surface of single glass conical nanochannels via surface-initiated atom transfer radical polymerization (ATRP). Switching the nanochannel between low and high ion conducting states was actuated by the temperature-sensitive PDMAEMA backbone. The PVBA backbone can bind with sugar, increasing the negative surface charge. Both backbones are complementary to pH responses and expand the nanochannel operating the range from acidic pH to basic pH values. | |
2 Experimental section
2.1 Materials
Prism glass capillaries (outer diameter ca. 1.35 mm, inner diameter ca. 0.95 mm, Hirschmann, Germany), platinum wires (diameter 25 μm, Alfa Aesar), tungsten wires (diameter 0.25 mm, 99.95%, Alfa Aesar); ferrocene (Fc, 99%, Alfa Aesar); tetra-n-butylammonium hexafluorophosphate (TBAPF6, 98%, AlfaAesar), 3-aminopropyl-triethoxylsilane (APTES, 99%, Acros), 2-bromoisobutyryl bromide (97%, Acros), 2-(dimethylamino) ethyl methacrylate (DMAEMA, 98.5%, Acros), CuBr (98%, Acros), 4-vinyl benzene boric acid (VPBA, 97%, Alfa Aesar), and 1,1,4,7,7-pentamethyldiethylenetriamine, (PMDETA, 98%, Acros) were used as received.
2.2 Fabrication of a single glass conical nanochannel
First, glass conical nanodisk electrodes were fabricated according to the bench top method reported by White and coworkers.57,58 A nanodisk sealed in the depth of a glass capillary was selected for experimentation. A tungsten wire was pulled out from a glass capillary after carefully breaking it. Then, one side was sealed with a sharp Pt wire while heating in aqua regia. A glass conical nanochannel was made after corrosion of the Pt wire.
2.3 Characterization
The confocal image of the conical nanochannel (Fig. S1†) was obtained using an inverted confocal microscope (Leica TCS SP5, Leica Microsystems). The SEM image of the Pt tip (Fig. S2†) was obtained by using a Hitachi S-4800 microscope.
2.4 Modification of the single nanochannel with P(DMAEMA-co-VPBA) and the corresponding ionic current measurements
Step 1: Silicon hydroxyl terminal modification. The nanochannel was treated with piranha acid for half an hour. Then, the nanochannel was washed with deionized water and ethanol several times.
Step 2: Amino terminal modification. A solution of 5% APTES in absolute ethanol was inserted into the nanochannel using a 100 μL microsyringe to react with the silicon hydroxyl groups on the interior surface for 30 min. Then, the nanochannel was rinsed with ethanol several times and dried at 110 °C in a vacuum oven for 30 min.
Step 3: Embellishment with an ATRP initiator. A solution containing 5 mL dichloromethane and 2 mL triethylamine was added into a 25 mL Schlenk bottle. The Schlenk bottle was placed in a 0 °C ice water bath, and pumped with argon gas for 15 min. Then, 1 mL of the ATRP initiator, 2-bromobutyryl bromide, was added dropwise. The mixed solution was injected into the nanochannel quickly using a 100 μL microsyringe. The nanochannel was placed into the Schlenk bottle and allowed to react in the ice water bath for 1 h. Then the nanochannel reacted for 12 h at room temperature. After the reaction was completed, the nanochannel was taken out and subjected to ultrasonic cleaning with dichloromethane. Finally, the nanochannel was dried at 110 °C in a vacuum oven for 30 min.
Step 4: P(DMAEMA-co-VPBA) copolymer brush modification via ATRP. A solution containing 5 mL methanol, 7 mL ethyl-N,N-dimethylamino-acrylate (DMAEMA) and 1.6 g 4-vinyl benzene boric acid (VPBA) was added into a 25 mL Schlenk bottle. The mixed solution was stirred until it dissolved completely while degassing by N2 bubbling for 30 min. Then, a solution containing 0.060 g CuBr and 0.2 mL 1,1,4,7,7-pentamethyldiethylenetriamine (PMDETA) was added. The nanochannel containing the ATRP initiator was filled with the mixture and placed into the Schlenk bottle. The Schlenk bottle was degassed by several high-vacuum/argon gas refill cycles. The plug of the bottle was sealed and the mixture was left overnight at room temperature. Finally, the glass conical nanochannel was rinsed with methanol and deionized water using a 100 μL microsyringe several times after ultrasonic cleaning with methanol.
2.5 Ionic current measurement
A 100 μL microsyringe was used to fill the electrolyte into single glass conical nanochannels with the electrolyte. The remaining air bubbles were removed by brief and mild sonication. An Ag/AgCl electrode (0.5 mm diameter), acting as the working electrode, was inserted into the channel. In addition, another Ag/AgCl electrode, serving as an auxiliary/reference electrode, was placed in the bulk solution, as close as possible to the tip of the nanochannel. In this work, the electrolyte filling both sides of the channel was the same. The current–voltage curve was recorded using a CHI 660C electrochemical workstation (Shanghai CHI Instrument Co. Ltd, China). Linear sweep voltammetry experiments were carried out by scanning the voltage from −1 V to +1 V with a scan rate of 100 mV s−1. The temperature was controlled using a constant temperature circulator (THX – 05, Ningbo TianHeng Instrument Co. Ltd, China), and the pH was adjusted by adding 0.1 M HCl or 0.1 M KOH.
3 Results and discussion
3.1 Monitoring the fabrication process of P(DMAEMA-co-VPBA)
The single conical nanopore (Fig. S1†) used in the study was prepared starting with a sharpened Pt wire (Fig. S2†) sealed in a glass capillary, according to a bench top method reported by White and coworkers.62–64 The modification of the single nanopore channel with P(DMAEMA-co-VPBA) is described in the Experimental section. Because the rectification characteristics of the nanofluidic diode largely relied on its pore surface properties, the modification procedures could be monitored by recording the current–voltage characteristics of this nanodevice. The test was performed in 10 mM KCl electrolyte at pH = 2 and pH = 7.4. The ratio of ionic rectification, which was used to quantify the rectification degree, is defined as the ratio R (I (“on” state)/I (“off” state)) of the absolute current values recorded for voltages of the same magnitude but at opposite polarity.
Fig. 1 shows the I–V responses for the nanodevice under acidic conditions (pH = 2, 10 mM KCl electrolyte). The typical I–V curve of the undecorated nanochannel was approximately linear, indicating no obvious ion rectification. After nanochannel modification using 3-aminopropyl-triethoxylsilane (APTES), the generated terminal amino groups were protonated at pH 2, which led to a positively charged nanopore surface. The current recorded at a positive voltage was higher than that recorded at a negative voltage. After reacting with the ATRP initiator, the functional groups changed from amino groups to alkyl bromide groups, generating a surface of electrically neutral alkyl bromide groups and resulting in an almost linear I–V curve. After modification of the P(DMAEMA-co-VPBA) copolymer brushes, the protonation of the tertiary amino groups induced a positively charged surface with a rectification ratio of 13.7, demonstrating the successful modification of multi-functional copolymers onto the walls of the nanochannel. Similarly, the I–V curves of the nanodevice at pH = 7.4 (10 mM KCl electrolyte) (Fig. S3†) were also recorded, which confirmed the success of the polymer modification on the nanopore surface.
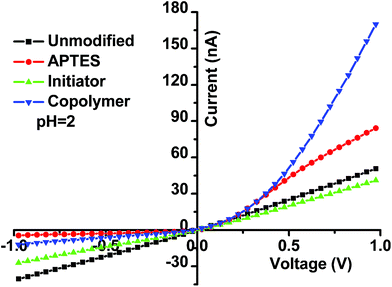 |
| Fig. 1 Monitoring of the modification procedure on a single glass conical nanopore channel (22 nm in orifice radius) by recording I–V curves at pH = 2, using a 10 mM KCl electrolyte. | |
3.2 pH-Stimuli-modulated ion transport
Fig. 2 shows the I–V curves of the single glass conical nanochannel modified with P(DMAEMA-co-VPBA) copolymer brushes under different pH conditions. As shown in Fig. 2B, the ionic currents at positive voltages were higher than those at negative voltages in acidic solution with a pH range of 2–6. In addition, the ion transport of the nanochannel was anion selective, which was caused by the protonation of tertiary amino groups in the PDMAEMA moiety of the copolymers. The isoelectric point of PDMAEMA (pKa) is 7.0–7.5.60,65 When pH < pKa, the tertiary amino group of the PDMAEMA backbone was protonated, leading to a positively charged nanochannel. When a negative voltage was applied, the K+ ion transferred from the bulk solution to the nanochannel, but the positively charged surface was electrostatically repulsive to the K+ ions. As a result, the nanochannel was in the “off” state with a low recorded current. When a positive voltage was applied, the Cl− ions transferred from the bulk solution into the nanochannel. The nanochannel was thus in the “on” state with a high recorded current. Under these conditions, the nanochannel was anion selective. Increasing the pH would reduce the degree of protonation of the tertiary amino groups, so that the current recorded at the positive voltage decreased. When the pH was adjusted to 7.4, the ionic rectifying phenomenon disappeared and the I–V curve was nearly linear.
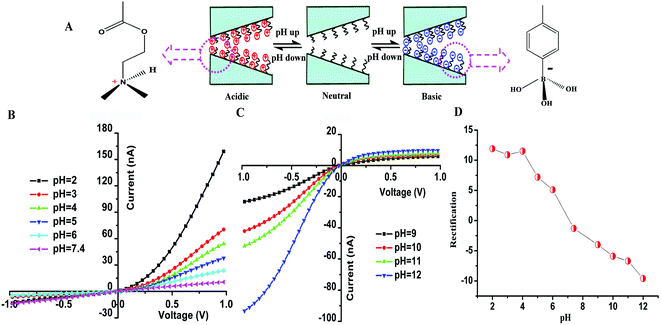 |
| Fig. 2 (A) Schematic diagram of the charge change modulated by pH on nanochannel walls modified with PDMAEMA-co-PVPBA. The pH dependence of the current rectification of a single glass conical nanochannel (with ∼22 nm orifice radius) modified with P(DMAEMA-co-VPBA) in 10 mM KCl with 1 mM PBS buffer solution. (B) I–V curves recorded at pH values from 2 to 7.4. (C) I–V curves recorded at pH values from 9 to 12. (D) The ion current rectification ratios of the nanochannel at pH values from 2 to 12. | |
As shown in Fig. 2C, the ionic currents recorded at negative voltages were higher than those at positive voltages under basic conditions. The ion transport of the nanochannel changed from anion to cation selective and the ionic rectifying direction was opposite to the direction under acidic conditions, which resulted from the dissociation of the boric acid groups in the copolymers (the pKa value of phenylboronic acids is ∼8
66). Benzene boric acid, a weak Lewis acid, can bind with OH−, resulting in a negative charge on the inner surface of the nanochannel. When the applied voltage was positive, the negatively charged nanochannel surface rejected the migration of Cl− ions from the bulk solution into the nanochannel, leading to the “off” state with a low conductivity in the nanochannel. While the applied voltage was negative, the K+ ions transferred from the bulk solution into the nanochannel, and the nanodevice was in the “on” state with a high recorded current due to their electrostatic attraction to the negative charges on the interior surface. Under these conditions, the nanochannel was cation selective (Fig. 2D). In addition, the current consistently reverses the direction in the single glass conical nanopore channel upon switching the pH between 2 and 12 (Fig. S7†).
These results demonstrated that the nanofluidic diode integrated with the appropriate co-polymer P(DMAEMA-co-VPBA) responded to the pH stimuli sensitively in a broad range of pH values (from 2 to 12) (Fig. 2D), which is very useful for designing pH sensors.
3.3 Temperature-stimuli-modulated ion transport
The influence of temperature on the conductivity was measured in the nanochannel. The polymer fragment PDMAEMA is a temperature-sensitive complex with an LCST between 40 and 50 °C.67,68 As shown in Fig. 3A, when the temperature is higher than the LCST, the conformation of the polymers changes from a swollen state to a collapsed state, leading to an increase in the effective size of the nanochannel.
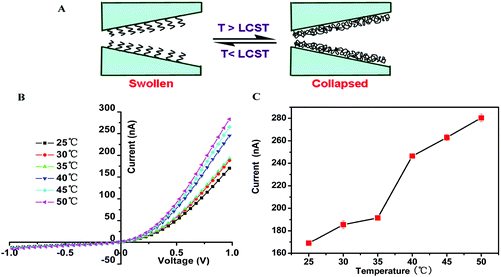 |
| Fig. 3 (A) Schematic diagram of the configuration change modulated by temperature on nanochannel walls modified with PDMAEMA-co-PVPBA. (B) I–V curve of a single glass conical nanochannel channel (22 nm in orifice radius) with P(DMAEMA-co-VPBA) modification in 10 mM KCl (pH = 2). (C) The relationship between the ionic current and temperature of the nanopore modified by P(DMAEMA-co-VPBA) at +1 V in pH = 2(10 mM KCl electrolyte). | |
Fig. 3B shows the I–V curves of the single glass conical nanochannel recorded from 25 to 50 °C. The curves of the nanochannel were similar at the lower temperature range, 25–35 °C, for both positive and negative voltages. When the temperature reached 40 °C, there was a sharp increase in the ionic current at positive potential voltages. In addition, the conductivity of the nanochannel increased significantly, because the temperature can control the strength of the hydrophobic interactions between the polymer fragment PDMAEMA and the solvent.60,65 In acidic solutions, the polymer fragment PDMAEMA becomes a positively charged polyelectrolyte due to the protonation of the tertiary amine, and the conformation of PDMAEMA is swollen due to geometrical constraints such as electrostatic repulsions and chain–solvent interactions. When above the LCST, the conformation of the polymer fragment PDMAEMA becomes collapsed due to adverse solvent conditions, leading to an increase of the effective cross section of the nanochannel and a sharp increase in conductivity (Fig. 3A). When the temperature is greater than the LCST from 40–50 °C, the copolymer chains remained in a state of collapse, and the effective cross-section of the nanochannel changes slightly. Therefore, the ion current increased slowly similar to the situation at the lower temperature range. In the control experiment, the ion current of the nanochannel without modification increased slowly and evenly from 25 to 50 °C (Fig. S4†). As shown in Fig. 3C, the current difference within 35–40 °C was much larger compared with the other temperature intervals. The abrupt current difference was 3 times higher than the increase in current in the control experiment that exhibited similar trends at pH = 7.4 (Fig. S5 and S6†). The experimental results indicated that the ion transport properties can be regulated by changing the ambient temperature.
3.4 Sugar-stimuli-modulated ion transport
The P(DMAEMA-co-VPBA) copolymer chains fabricated in the inner surface of the nanochannel contain many benzene boric acid groups that can recognize sugar molecules. Fig. 4A shows the transition of the surface charge in the nanochannel upon introducing sugar as the stimulus. Using a blank electrolyte solution (pH = 7.4), an almost linear I–V curve was measured, with a rectification ratio of approximately (+) 1.3, due to the neutral inner surface. We further investigated the influence of sugar by adding a series of varying glucose and fructose concentrations into the electrolyte solution (Fig. 4B and C). The current recorded at a negative voltage increased with the addition of glucose/fructose, whereas the current at positive voltages decreased. The rectification ratio of the nanofluidic diode rose with the increasing concentrations of sugar from 0 to 1 mM, resulting from the increase of negative surface charges. When the concentration of sugar was higher than 1 mM, the I–V curve changed only slightly, indicating that the binding of sugars onto the boric acid groups on the interior surface had reached saturation.
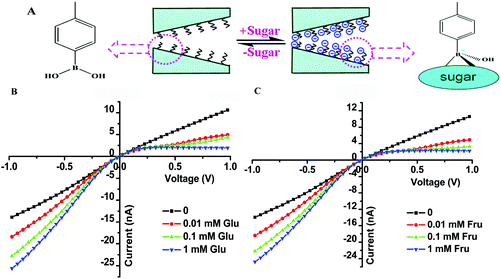 |
| Fig. 4 (A) Schematic diagram of the charge change modulated by sugar on the nanochannel wall modified with P(DMAEMA-co-VPBA). (B) I–V curves of the P(DMAEMA-co-VPBA) modified single glass conical nanochannel (22 nm in orifice radius) in 10 mM KCl (pH = 7.4) with different concentrations of glucose. (C) I–V curves with different concentrations of fructose. | |
Fig. 5 shows that the ionic current rectification ratio of the nanochannel modified with P(DMAEMA-co-VPBA) increases with the concentration of glucose/fructose under neutral conditions. Glucose/fructose is rich in hydroxyl groups, and can bind to phenylboronic acid. Additionally, the rectification ratio in the fructose solution is slightly higher than that in the glucose solution. The reason is that the negative charges on the pore wall are more abundant with fructose due to its higher binding constant with phenylboronic acid compared with fructose, and the generation of boric acid esters is relatively higher at the same concentration. Compared with hydroxyl groups, it seems that the sugars rectify the nanochannel better and the rectification ratio is higher.
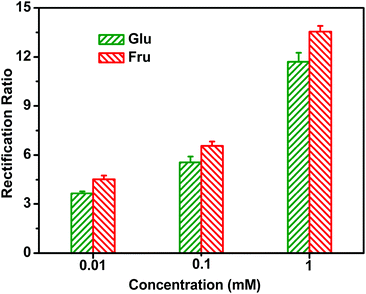 |
| Fig. 5 The relationship between the ionic current rectification ratio and the concentration of glucose/fructose for the nanopore modified by P(DMAEMA-co-VPBA) in 10 mM KCl electrolyte at pH = 7.4. | |
The rectification ratio of the nanochannel can reflect its ability to regulate the ion transport. The rectification phenomenon of the nanochannel modified with PDMAEMA-co-PVPBA disappears in a neutral electrolyte solution (Fig. 2D). However, we can recover the rectification ratio by adding sugar without changing the ionic strength of the solution. Moreover, we can control the regulation of ion transport by adjusting the concentration of sugar in the solution and remove the sugar after ultrasonic cleaning in an acidic solution because the binding between benzene boric acid and sugar is reversible (Fig. S8†), which could be very useful for drug delivery and separation analysis.
4 Conclusion
In summary, we successfully created a copolymer P(DMAEMA-co-VPBA) modified single glass conical nanochannel via atom transfer radical polymerization (ATRP), and demonstrated, for the first time, the tri-stimuli-responsive (pH, temperature, and sugar) ion transport properties of this biomimetic nanochannel. The ion current rectification of the nanofluidic diode changed dramatically with the variation of pH values from acidic (2) to basic (12), and the rectification direction even switched at neutral pH, indicating a wide stimulus response range to pH. Additionally, for the regulation of the environmental temperature, when T > LCST, the ion current of the functionalized nanochannel increased due to the conformational transition of PDMAEMA under the temperature stimuli. Moreover, the nanodevice switched from a non-rectifying state to a rectifying state with the addition of sugar. As advancement over the single or dual external stimuli systems, the triple-stimuli-responsive nanofluidic device developed in this work is a solid step forward for producing highly intelligent and closely biomimetic nanochannels. This research not only provides a platform for the creation of tailor-made nanofluidic devices, but also suggests a broad application in drug delivery, separation analysis, materials science and biosensors.
Acknowledgements
This work was supported by the National Natural Science Foundation of China (21375111, 21127005 and 21521004), the 973 Program of China (2013CB933703) and the funds of the Ministry of Education of China (20110121110011and PCSIRT IRT13036).
Notes and references
- B. Hille, Biophys. J., 1978, 22, 283–294 CrossRef CAS PubMed.
- E. Perozo, D. M. Cortes, P. Sompornpisut, A. Kloda and B. Martinac, Nature, 2002, 418, 942–948 CrossRef CAS PubMed.
- T. M. Fyles, Chem. Soc. Rev., 2007, 36, 335–347 RSC.
- C. R. Martin and Z. S. Siwy, Science, 2007, 317, 331–332 CrossRef CAS PubMed.
- Z. S. Siwy, Adv. Funct. Mater., 2006, 16, 735–746 CrossRef CAS.
- S. Alberti, G. J. A. A. Soler-Illia and O. Azzaroni, Chem. Commun., 2015, 51, 6050–6075 RSC.
- Y. Q. Li, Y. B. Zheng and R. N. Zare, ACS Nano, 2012, 6, 993–997 CrossRef CAS PubMed.
- X. Hou, W. Guo and L. Jiang, Chem. Soc. Rev., 2011, 40, 2385–2401 RSC.
- L. Wen, X. Hou, Y. Tian, F. Q. Nie, Y. Song, J. Zhai and L. Jiang, Adv. Mater., 2010, 22, 1021–1024 CrossRef CAS PubMed.
- L. Wen, Y. Tian and L. Jiang, Angew. Chem., 2015, 54, 3387–3399 CrossRef CAS PubMed.
- L. Wen and L. Jiang, Natl. Sci. Rev., 2014, 1, 144–156 CrossRef.
- M. Ali, P. Ramirez, S. Mafe, R. Neumann and W. Ensinger, ACS Nano, 2009, 3, 603–608 CrossRef CAS PubMed.
- X. Hou, Y. J. Liu, H. Dong, F. Yang, L. Li and L. Jiang, Adv. Mater., 2010, 22, 2440–2443 CrossRef CAS PubMed.
- G. Perez-Mitta, L. Burr, J. S. Tuninetti, C. Trautmann, M. E. Toimil-Molares and O. Azzaroni, Nanoscale, 2016, 8, 1470–1478 RSC.
- B. Yameen, M. Ali, R. Neumann, W. Ensinger, W. Knoll and O. Azzaroni, J. Am. Chem. Soc., 2009, 131, 2070–2071 CrossRef CAS PubMed.
- B. Yameen, M. Ali, R. Neumann, W. Ensinger, W. Knoll and O. Azzaroni, Nano Lett., 2009, 9, 2788–2793 CrossRef CAS PubMed.
- L. X. Zhang, X. H. Cao, Y. B. Zheng and Y. Q. Li, Electrochem. Commun., 2010, 12, 1249–1252 CrossRef CAS.
- Z. Guo, J. Wang, J. Ren and E. Wang, Nanoscale, 2011, 3, 3767–3773 RSC.
- K. Han, L. Heng, L. Wen and L. Jiang, Nanoscale, 2016, 8, 12318–12323 RSC.
- Z. Zhang, X. Y. Kong, K. Xiao, G. Xie, Q. Liu, Y. Tian, H. Zhang, J. Ma, L. Wen and L. Jiang, Adv. Mater., 2016, 28, 144–150 CrossRef CAS PubMed.
- Z. Zhang, X. Y. Kong, K. Xiao, Q. Liu, G. Xie, P. Li, J. Ma, Y. Tian, L. Wen and L. Jiang, J. Am. Chem. Soc., 2015, 137, 14765–14772 CrossRef CAS PubMed.
- W. Guo, H. W. Xia, F. Xia, X. Hou, L. X. Cao, L. Wang, J. M. Xue, G. Z. Zhang, Y. L. Song, D. B. Zhu, Y. G. Wang and L. Jiang, ChemPhysChem, 2010, 11, 859–864 CrossRef CAS PubMed.
- B. Yameen, M. Ali, R. Neumann, W. Ensinger, W. Knoll and O. Azzaroni, Small, 2009, 5, 1287–1291 CrossRef CAS PubMed.
- Z. Zhang, X. Y. Kong, G. Xie, P. Li, K. Xiao, L. Wen and L. Jiang, Sci. Adv., 2016, 2, e1600689 Search PubMed.
- I. Vlassiouk, C. D. Park, S. A. Vail, D. Gust and S. Smirnov, Nano Lett., 2006, 6, 1013–1017 CrossRef CAS PubMed.
- G. Wang, A. K. Bohaty, I. Zharov and H. S. White, J. Am. Chem. Soc., 2006, 128, 13553–13558 CrossRef CAS PubMed.
- C. C. Harrell, P. Kohli, Z. Siwy and C. R. Martin, J. Am. Chem. Soc., 2004, 126, 15646–15647 CrossRef CAS PubMed.
- J. Wu, K. Gerstandt, H. B. Zhang, J. Liu and B. J. Hinds, Nat. Nanotechnol., 2012, 7, 133–139 CrossRef CAS PubMed.
- J. Y. Lin, C. Y. Lin, J. P. Hsu and S. Tseng, Anal. Chem., 2016, 88, 1176–1187 CrossRef CAS PubMed.
- S. Zhao, Y. B. Zheng, S. L. Cai, Y. H. Weng, S. H. Cao, J. L. Yang and Y. Q. Li, Electrochem. Commun., 2013, 36, 71–74 CrossRef CAS.
- Q. H. Nguyen, M. Ali, R. Neumann and W. Ensinger, Sens. Actuators, B, 2012, 162, 216–222 CrossRef CAS.
- Z. Y. Sun, C. P. Han, L. Wen, D. M. Tian, H. B. Li and L. Jiang, Chem. Commun., 2012, 48, 3282–3284 RSC.
- P. B. Tiwari, L. Astudillo, J. Miksovska, X. Wang, W. Li, Y. Darici and J. He, Nanoscale, 2014, 6, 10255–10263 RSC.
- H. Jiang, E. K. Wang and J. H. Wang, RSC Adv., 2015, 5, 35622–35630 RSC.
- X. Hou, W. Guo, F. Xia, F. Q. Nie, H. Dong, Y. Tian, L. P. Wen, L. Wang, L. X. Cao, Y. Yang, J. M. Xue, Y. L. Song, Y. G. Wang, D. S. Liu and L. Jiang, J. Am. Chem. Soc., 2009, 131, 7800–7805 CrossRef CAS PubMed.
- Y. Tian, X. Hou, L. P. Wen, W. Guo, Y. L. Song, H. Z. Sun, Y. G. Wang, L. Jiang and D. B. Zhu, Chem. Commun., 2010, 46, 1682–1684 RSC.
- P. Actis, B. Vilozny, R. A. Seger, X. Li, O. Jejelowo, M. Rinaudo and N. Pourmand, Langmuir, 2011, 27, 6528–6533 CrossRef CAS PubMed.
- M. Ali, S. Nasir, P. Ramirez, J. Cervera, S. Mafe and W. Ensinger, ACS Nano, 2012, 6, 9247–9257 CrossRef CAS PubMed.
- Y. Tian, Z. Zhang, L. P. Wen, J. Ma, Y. Q. Zhang, W. D. Liu, J. Zhai and L. Jiang, Chem. Commun., 2013, 49, 10679–10681 RSC.
- C. Zhao, X. S. Li, L. Y. Li, X. Gong, Y. Chang and J. Zheng, Chem. Commun., 2013, 49, 9317–9319 RSC.
- L. J. Gao, P. Li, Y. Q. Zhang, K. Xiao, J. Ma, G. H. Xie, G. L. Hou, Z. Zhang, L. P. Wen and L. Jiang, Small, 2015, 11, 543–547 CrossRef CAS PubMed.
- P. Li, X. Y. Kong, G. Xie, K. Xiao, Z. Zhang, L. Wen and L. Jiang, Small, 2016, 12, 1854–1858 CrossRef CAS PubMed.
- Q. Liu, L. Wen, K. Xiao, H. Lu, Z. Zhang, G. Xie, X. Y. Kong, Z. Bo and L. Jiang, Adv. Mater., 2016, 28, 3181–3186 CrossRef CAS PubMed.
- Q. Liu, K. Xiao, L. Wen, H. Lu, Y. Liu, X. Y. Kong, G. Xie, Z. Zhang, Z. Bo and L. Jiang, J. Am. Chem. Soc., 2015, 137, 11976–11983 CrossRef CAS PubMed.
- Y. Chen, D. Zhou, Z. Meng and J. Zhai, Chem. Commun., 2016, 52, 10020–10023 RSC.
- G. Perez-Mitta, A. G. Albesa, W. Knoll, C. Trautmann, M. E. Toimil-Molares and O. Azzaroni, Nanoscale, 2015, 7, 15594–15598 RSC.
- L. X. Zhang, S. L. Cai, Y. B. Zheng, X. H. Cao and Y. Q. Li, Adv. Funct. Mater., 2011, 21, 2103–2107 CrossRef CAS.
- X. Hou, F. Yang, L. Li, Y. L. Song, L. Jiang and D. B. Zhu, J. Am. Chem. Soc., 2010, 132, 11736–11742 CrossRef CAS PubMed.
- W. Guo, H. W. Xia, L. X. Cao, F. Xia, S. T. Wang, G. Z. Zhang, Y. L. Song, Y. G. Wang, L. Jiang and D. B. Zhu, Adv. Funct. Mater., 2010, 20, 3561–3567 CrossRef CAS.
- M. H. Zhang, X. Hou, J. T. Wang, Y. Tian, X. Fan, J. Zhai and L. Jiang, Adv. Mater., 2012, 24, 2424–2428 CrossRef CAS PubMed.
- L. P. Wen, Q. Liu, J. Ma, Y. Tian, C. H. Li, Z. S. Bo and L. Jiang, Adv. Mater., 2012, 24, 6193–6198 CrossRef CAS PubMed.
- K. Xiao, G. H. Xie, P. Li, Q. Liu, G. L. Hou, Z. Zhang, J. Ma, Y. Tian, L. P. Wen and L. Jiang, Adv. Mater., 2014, 26, 6560–6565 CrossRef CAS PubMed.
- Q. Q. Zhang, Z. Y. Liu, K. F. Wang and J. Zhai, Adv. Funct. Mater., 2015, 25, 2091–2098 CrossRef CAS.
- M. Ali, B. Schiedt, R. Neumann and W. Ensinger, Macromol. Biosci., 2010, 10, 28–32 CrossRef CAS PubMed.
- I. Vlassiouk, T. R. Kozel and Z. S. Siwy, J. Am. Chem. Soc., 2009, 131, 8211–8220 CrossRef CAS PubMed.
- M. Liu, H. Zhang, K. Li, L. Heng, S. Wang, Y. Tian and L. Jiang, Adv. Funct. Mater., 2015, 25, 421–426 CrossRef CAS.
- H. M. Wang, S. N. Hou, Q. Q. Wang, Z. W. Wang, X. Fan and J. Zhai, J. Mater. Chem. B, 2015, 3, 1699–1705 RSC.
- L.-X. Zhang, Y.-B. Zheng, S.-L. Cai, X.-H. Cao and Y.-Q. Li, Chin. Chem. Lett., 2015, 26, 43–46 CrossRef CAS.
- S. L. Cai, Y. B. Zheng, S. H. Cao, X. H. Cai and Y. Q. Li, Chem. Commun., 2016, 52, 12450–12453 RSC.
- O. Schepelina and I. Zharov, Langmuir, 2008, 24, 14188–14194 CrossRef CAS PubMed.
- T. M. B. Islam, K. Yoshino and A. Sasane, Anal. Sci., 2003, 19, 455–460 CrossRef CAS PubMed.
- B. Zhang, J. Galusha, P. G. Shiozawa, G. L. Wang, A. J. Bergren, R. M. Jones, R. J. White, E. N. Ervin, C. C. Cauley and H. S. White, Anal. Chem., 2007, 79, 4778–4787 CrossRef CAS PubMed.
- B. Zhang, Y. H. Zhang and H. S. White, Anal. Chem., 2004, 76, 6229–6238 CrossRef CAS PubMed.
- B. Zhang, Y. H. Zhang and H. S. White, Anal. Chem., 2006, 78, 477–483 CrossRef CAS PubMed.
- N. Nordgren and M. W. Rutland, Nano Lett., 2009, 9, 2984–2990 CrossRef CAS PubMed.
- J. Yan, G. Springsteen, S. Deeter and B. H. Wang, Tetrahedron, 2004, 60, 11205–11209 CrossRef CAS.
- F. Q. Zeng, Y. Q. Shen, S. P. Zhu and R. Pelton, J. Polym. Sci., Part A: Polym. Chem., 2000, 38, 3821–3827 CrossRef CAS.
- S. H. Cho, M. S. Jhon, S. H. Yuk and H. B. Lee, J. Polym. Sci., Part B: Polym. Phys., 1997, 35, 595–598 CrossRef CAS.
Footnotes |
† Electronic supplementary information (ESI) available. See DOI: 10.1039/c6nr07339e |
‡ These authors contributed equally to this work. |
|
This journal is © The Royal Society of Chemistry 2017 |