Selective biomolecular separation system inspired by the nuclear pore complex and nuclear transport†
Received
25th January 2017
, Accepted 6th March 2017
First published on 10th April 2017
Abstract
Inspired by selective molecular transport in the nuclear pore complex, a system that can separate proteins using a facilitated diffusion-based mechanism is developed. The system is comprised of two components: nuclear transporter receptors (NTRs) fused with a peptide tag that can “catch” target biomolecules and a nucleoporin-like protein (NLP) hydrogel that can selectively “trap” target molecule-NTR complexes due to the physical binding between hydrophobic Phe-Gly sequences in the protein polymer network and the NTR. Similar to the nuclear pore complex, substrate selectivity is achieved via complexation with NTRs, which permits transport into the gel, whereas environmental proteins are rejected. The NLP hydrogel effectively transports cargo complexes down to the single nanomolar range in the environment, and a selectivity of approximately 300 can be achieved between target molecule and protein impurity. High selectivity requires both interactions between the hydrogel and cargo complex for efficient “catching” as well as high NLP gel concentration to reduce the pore size for rejecting environmental molecules. As a proof of concept of “catch-trap” for a protein target, a partial green fluorescent protein (GFP) sequence and staphylococcal enterotoxin B (SEB) toxoid are both separated from protein mixtures using engineered NTRs that contain binding tags for the corresponding protein. This represents a versatile system for fusing nuclear transporters with diverse peptide tags, enabling the selective binding and transport of a wide variety of separations of high value biomolecules.
Design, System, Application
Pores in the nuclear envelope are filled with a protein hydrogel that regulates passage of macromolecules into and out of the nucleus with both high selectivity and high transport rate. Therefore, it provides biological inspiration for the design of filters that could selectively separate biomolecules such as proteins or biotoxins. Here, we mimic the nuclear pore system based on two engineered components: a protein hydrogel prepared from minimal consensus repeats that mimic the gel-forming nucleoporins, and nuclear transporters that are engineered to bind and recognize specific proteins, facilitating their selective transport through the filtering gel. In contrast to chromatographic separations, the desired molecule selectively permeates the gel at a higher rate than impurities, providing a new filtration-based mechanism for selective bioseparations. This could have important future applications in areas such as protein purification or dialysis to remove toxic compounds from biological fluids.
|
Introduction
Understanding the function of biological systems provides an inspiration for developing new functional materials, such as plant polyphenol-inspired surface coatings,1 mussel byssus mimicking adhesives,2,3 muscle-inspired energy dissipative materials,4,5 and thermoresponsive elastin-based drug delivery vehicles.6 One very interesting natural system is the nuclear pore complex (NPC), which allows the transport of select molecules between the cytoplasm and the nucleus through the nuclear membrane, while rejecting the passage of undesired molecules.7 This biological system is rare among materials in its capability to achieve highly selective biomolecular separations. Industrially, the selective separation of biomolecules is traditionally achieved by affinity chromatography where particles conjugated with chemical and biological affinity tags adsorb target molecules on their modified surface. Molecular sequestration can be enhanced by using antigens or peptides conjugated to a polymer network.8 Any molecule smaller than the pore size can enter the network, and selectively binding molecules will be absorbed by their binding partners. This offers the advantage of capturing molecules throughout the network. However, in both cases, the approach is based on adsorption where non-interacting molecules pass across the material while interacting molecules are bound and retained to varying degrees.
In contrast to chromatography and adsorption, NPCs, which perforate the nuclear membrane, act as biologically selective filters that reject passage of non-interacting molecules while selectively allowing passage of interacting molecules.7,9 A biopolymer network, composed of proteins named nucleoporins, fills the NPCs and functions as the selective filter. The process is initiated by forming a macromolecular complex between the cargo and the nuclear transport receptors (NTRs) in the cytoplasm. The NTRs physically bind to hydrophobic Phe-Gly (FG) repeats within the nucleoporin network via hydrophobic interactions,10 and the NTR-cargo complexes are passively translocated into the nucleus. The cargo alone is unable to translocate efficiently into the nucleus.11 Unlike synthetic filters for biological separations (e.g. filters for removing viral particles), the NPC is capable of separating molecules using multiple parameters for complex biological selectivity. Importantly, while most man-made filters operate based on the size of objects to be separated, where particles smaller than the filter mesh size are eluted, the NPC does not rely on size only, and large complexes can be selectively transported. For example, less than 1% of total biomolecules above the mesh size limit of the nucleoporin network (30–40 kg mol−1) still can penetrate the network when bound to the NTRs.11,12 Although the specific mechanisms by which the nuclear pore establishes its high selectivity is still being investigated,7,13,14 it is clear that engineered variants of the nuclear pore complex and nuclear transporters could enable the development of highly selective new biological separation systems that operate continuously (i.e. filters) rather than in batch mode (i.e. affinity chromatography) which can impact defense and biomedical technology, including the removal of biotoxins from food.15,16
Based on a minimal consensus repeat of the C-terminal sequence of nucleoporin Nsp1, we recently developed nucleoporin-like protein (NLP) hydrogels17 that mimic the selective separation ability of the natural protein nucleoporin. Each consensus repeat contains one FG sequence (16 total in a full length NLP protein), and a gel is formed by the association of telechelic coiled-coil domains which were fused on either sides of the repeats, forming physical crosslinks.18,19 Similar to the natural system, target molecule-NTR complexes can bind to the NLP hydrogel because of the binding affinity between NTRs and FG repeats in the gel network. Since numerous free FG sites are available inside the gel (e.g. 69.9 mM of FG in 20 wt% gel), the target molecule-NTR complexes diffuse quickly into the gel. For example, while a non-interacting molecule with molar mass of 67 kg mol−1 is mostly blocked, a model cargo with molar mass of 75 kg mol−1 easily permeates the gel if bound to the nuclear transporter, importin β (97 kg mol−1; total molar mass of the complex is 172 kg mol−1), similar to natural nucleoporin hydrogel.17 Porosity restricts transport of molecules above approximately 30 kg mol−1, and large proteins only permeate the gel in the form of cargo-NTR complex.17 These complexes are highly concentrated in the gel compared to the environment because of the binding between NTRs of the macromolecular complexes and FG sites within the gel, as observed in the natural system.11 Therefore, this engineered protein analog provides the potential to capture the biologically selective filtration of the nuclear pore in a synthetic polymeric system.
In this work, a versatile NPC-inspired selective biomolecular separation system is developed using engineered NTRs to catch target molecules and the NLP hydrogel to capture the target molecules associated with the NTRs. To accomplish this catch-trap strategy, native NTRs can be genetically fused with diverse peptide tags to physically catch the biomolecules of interest. The NTR-target biomolecule complex can be selectively transported and captured into the NLP hydrogel, while other inert molecules, unbound to NTRs, can be rejected to enter the NLP gel (Scheme 1). Among NTRs, nuclear transport factor 2 (NTF2) was selected due to its structural and physicochemical properties.20–22 The small size of NTF2 (molar mass of 14 kg mol−1, compared to other NTRs, such as importin β with 97 kg mol−1 (ref. 10)) is beneficial to enhance the biosynthesis yield. Moreover, the relatively weak binding affinity of NTF2 to FG repeats (dissociation constant, Kd, ∼ 20 μM),11,20,23 allows fast transport from the gel surface. By investigating the capabilities of the biosynthetic polymeric systems under several circumstances, it is shown that the system can uptake target molecule from the environment down to a single nanomolar range, and the best barrier function to other environmental biomolecules can be obtained by reducing the gel mesh size by increasing the gel concentration. Selective target uptake strongly depends on the strength of molecular interaction between engineered NTRs and the target. To the best our knowledge, this is the first NPC-inspired bimolecular separation system, with the potential to lead to new separations in areas such as defense and biomedical technology.
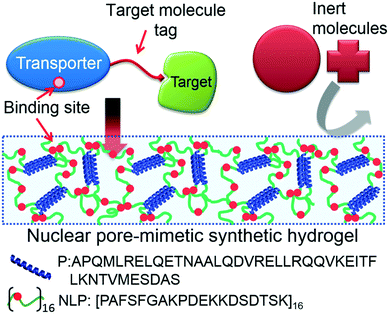 |
| Scheme 1 Design concept for selective biomolecular separation by reverse engineering of the natural nuclear pore complex system. The system is composed of engineered nuclear transporter with a peptide tag that can physically bind to its target molecule and nucleoporin-like protein (NLP) hydrogel which rejects the entry of inert molecules. NLP is a protein polymer composed of 16 repeats of consensus repeats extracted from one of natural nucleoporins Nsp1 and flanked by pentameric (P) coiled-coil domains as a physical network crosslinker.18,19 Red filled circles represent FG sequences. | |
Experimental section
Non-toxic staphylococcal enterotoxin B toxoid (SEB; catalog # NR-10049), recombinant protein from Escherichia coli (E. coil), was obtained from BEI Resources (Manassas, VA, USA). Bovine serum albumin (BSA; catalog # A7906), immunoglobulin G (IgG; catalog # 340-21), fluorescein isothiocyanate (FITC; catalog # L09319) and Alexa Fluore 633 NHS Ester (catalog # A20005) were purchased from Sigma-Aldrich (St. Louis, MO, USA), LEE Biosolutions (Maryland Heights, MO, USA), Alfa Aesar (Tewksbury, MA, USA) and Thermo Fischer Scientific (Waltham, MA USA), respectively. DNA genes of nuclear transport factor 2 (NTF2; NCBI reference sequence: NP_005787) and green fluorescent protein 1–10, (GFP1–10)24–26 were purchased from GenScript (Piscataway, NJ 08854USA).
DNA engineering
NTF2 was amplified by polymerase chain reaction (PCR) to prepare KpnI -NTF2- SpeI - 6xHis tag - BamHI - GFP11,24–26 R418 (ref. 15) or R445 (ref. 15) tags - stop codon - HindIII. All DNA fragments were subcloned into the pQE30 expression plasmid. A superfolder green fluorescent protein27 followed by 6xHis tag and stop codon was amplified by PCR and subcloned into the prepared pQE30-NTF2-GFP11 plasmid using SpeI and HindIII restriction enzyme cleavage sites. Sequences of all plasmids were confirmed by gene sequencing (Genewiz; Boston, MA, USA). All prepared protein sequences and their expression plasmids are summarized in Fig. S1 and Table S1.†
Protein expression and purification of NTF2, NTF2-GFP11, NTF2-R418, NTF2-R445 and NTF2-GFP
All genes were transformed into SG13009 (pRep4) cells. For expression, a freshly-grown bacterial colony was inoculated in 10 mL LB medium with 100 μg mL−1 ampicillin and 50 μg mL−1 kanamycin at 37 °C overnight. 10 mL of overnight culture of each sample was inoculated into 1 L 2× YT media containing 2% glycerol and 30 mM K2HPO4 with 100 μg mL−1 ampicillin and 50 μg mL−1 kanamycin. When OD600 reached 0.5 by culture at 37 °C, expression was induced overnight at room temperature with the addition of 0.5 mM IPTG. The cells were then harvested and stored at −80 °C. Thawed cells were sonicated in buffer containing 50 mM NaH2PO4, 300 mM NaCl, 10 mM imidazole, 10 mM 2-mercaptoethanol and 2 mM phenylmethylsulfonyl fluoride. After sonication, DNase, RNase and lysozyme were added and stored for 1 hour at 4 °C. Cell lysates were clarified by centrifugation (14
000g for 30 min at 4 °C), and the proteins of interest were purified by Ni-NTA affinity chromatography under non-denaturing conditions with 250 mM imidazole used for protein elution. The proteins were further purified by ion exchange chromatography using a HiTrap Q HP column (GE Healthcare, Sweden) in an ÄKTA pure FPLC. Samples containing the desired protein were concentrated using 3 kDA or 10 kDa centrifugal filters (Amicon Ultra-15, catalog # UFC900324 and UFC 901024, EMD Millipore; Billerica, MA, USA) and the buffer was exchanged to phosphate-buffered saline (PBS; catalog # 21-040-CV, Corning cellgro). The purity of samples was determined to be greater than 95% by SDS-PAGE analysis (Fig. S3†). The concentration of proteins was determined using the optical absorbance at 280 nm and more than 100 mg of each protein were obtained from 1 L cell culture.
GFP1–10 synthesis
The GFP1–10 gene in pET15b expression plasmid was transformed into E. coli OverExpress C41(DE3) cells (Lucigen; Middleton, WI, USA). The overnight culture was prepared using the same procedure described above, but only with 100 μg mL−1 ampicillin. 10 mL of overnight culture was inoculated into 1 L LB media at 37 °C with 100 μg mL−1 ampicillin and grown until OD600 ∼ 1. Expression was then induced at 20 °C for 48 hours with the addition of 1 mM IPTG. The cells were harvested and resuspended in 50 mM Tris (pH 8), 300 mM NaCl and 8 M urea, and frozen at −80 °C. Thawed cells were sonicated and clarified by centrifugation (14
000g for 30 min at 4 °C). The proteins of interest were purified by Ni-NTA affinity chromatography under denaturing conditions with 250 mM imidazole used for protein elution. Purified samples were dialyzed against deionized (DI) water, and then 20 mM Tris (pH 8) and 6 M urea were added. The proteins were further purified by ion exchange chromatography using a HiTrap Q HP column (catalog # 17-1154-01, GE Healthcare Life Sciences; Pittsburgh, PA, USA) in an ÄKTA pure FPLC. Samples containing the desired protein were concentrated using a 10 kDa centrifugal filter, and the buffer was exchanged to 20 mM Tris (pH 7.8) and 9 M urea. The purity of samples was determined to be greater than 95% by SDS-PAGE analysis (Fig. S3†).
Fluorescent tag labeling
In order to enhance the fluorescence intensity of NTF2-GFP, the protein was labeled by fluorescein isothiocyanate (FITC) with a molar ratio of 1
:
10 in PBS (pH 9). After overnight reaction at 4 °C, samples were dialyzed against 20 mM Tris (pH 8) and 100 mM NaCl. FITC labeled NTF2-GFP was purified using ion exchange chromatography with a HiTrap Q HP column in an ÄKTA pure FPLC, and then the buffer was exchanged to PBS by using 10 kDa centrifugal filter (catalog # UFC901008, EMD Millipore). SEB was labeled by FITC and IgG, and BSA were labeled by Alexa Fluor 633, with the same incubation condition for FITC-NTF2-GFP but with pH of 8–8.3. Fluorescently tagged proteins were separated from untagged free fluorescent dyes by illustra NAP™ 10 column (catalog # 17-0854-01, GE Healthcare Life Sciences). The samples were dialyzed against 20 mM Tris (pH 8) and 100 mM NaCl and then concentrated while exchanging the buffer to PBS using a 10 kDa centrifugal filter.
Solution preparation for capillary assays
All capillary tests (Fig. 1–5 and Fig. S5 and S6†) contain engineered NTF2, natural NTF2 and target molecules with concentrations noted in the figure captions. In addition, for selectivity tests (Fig. 3–5 and Fig. S6†), 167 μM IgG, 600 μM BSA, 2 μM 633Alexa Fluor-IgG and 2 uM 633 Alexa Fluor-BSA were mixed with the NTF2 and target molecules. PBS was used in all capillary assays.
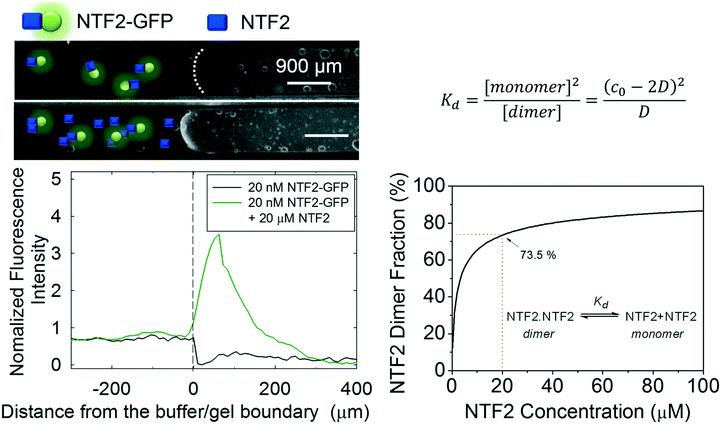 |
| Fig. 1 The effect of additional NTF2 on NTF2-GFP transport into NLP gel. In the presence or absence of 19.98 μM NTF2 with 20 nM NTF2-GFP, 20 nM GFP can be observed to transport into the gel (left) with surplus NTF2 where more than 70% of NTF2 dimer fraction is expected (right). This result confirms that NTF2 dimer is important for binding between the gel and NTF2 transporter and could be the reason of the NTF2 concentration in the nuclear pore complexes.20 The curve was generated by solving the quadratic equation shown in the top right, where c0 and D represent the analytical NTF2 and dimer concentrations, respectively. The equilibrium dissociation constant Kd is obtained in elsewhere.21 | |
Hydrogel preparation
P-2NLP-P and P-C30-P proteins were produced by the procedure described in the previous work.17 Lyophilized P-2NLP-P and P-C30-P samples were dissolved at a concentration of 50 mg mL−1, 100 mg mL−1 or 200 mg mL−1 in PBS and allowed to form a gel at either room temperature or 4 °C.
Capillary transport measurements
1.5 inch borosilicate capillaries with 0.9 mm inner diameters (catalog # 8290, Vitrocom; Mountain Lakes, NJ, USA) were loaded by piercing pre-made hydrogels. The prepared solutions were injected into the capillary and both ends of the capillary were sealed with a 1
:
1
:
1 mixture of vaseline, lanolin, and paraffin. Time lapses of cargo transport into the hydrogels were taken at 1 minute intervals for 1–12 hours on a Nikon Ti Eclipse inverted microscope using a Nikon CFI Plan UW 2X and Hamamatsu C11440-22CU camera. All fluorescence intensity profiles were obtained by averaging the fluorescence intensity within 100 μm slice width through the center of the gel and across the gel/buffer interface. The profiles were normalized by the bath concentration in the capillaries at the first time point. The interface between gel and buffer is assigned by a 20% change in the intensity compared to the initial bath fluorescence intensity as the zero point of the distance scale in each fluorescence intensity profile.
Selectivity calculations
The selectivity at a given time (α) for transport of target biomolecules during the capillary assay is given by | 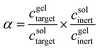 | (1) |
where cgeli and csoli are the concentration of component i in the gel and solution at a given time, respectively, where target is FITC-NFT2-GFP and inert molecules are IgG and BSA conjugated with Alexa fluor 633. In this experimental condition, since concentrations of each biomolecule are directly proportional to fluorescence intensity, FITC or Alexa fluor 633 (see Fig. S7†), the concentration ratios can be substituted by fluorescence ratios. In order to be quantitative, the fluorescence in the gel is calculated from the decrease in fluorescence in the buffer, more than 1 mm away from the gel interface. This avoids challenges with aggregation in the gel that are outside of the linear range, the shape of the gel/fluid interface, and position-dependence of the fluorescence intensity within the gel phase. Thus, the concentration ratios are given by | 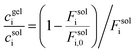 | (2) |
where Fsoli is the fluorescence of component i in solution at a given time, and Fsoli,0 is the fluorescence in the buffer at the beginning of the experiment, when the number of biomolecules uptaken by the gel is negligible.
Results and discussion
Engineered NTF2 synthesis
High synthetic yields of engineered NTF2s were attained when peptide tags or a protein were fused onto their C-terminus by standard recombinant DNA techniques (Fig. S1 and Table S1†). When peptide tags were on the N-terminus, engineered NTF2 precipitated during protein synthesis (Fig. S2†). On the contrary, when the tags or a foreign-protein were fused on the C-terminus of NTF2, all NTF2 fusions were soluble (Fig. S3†). A hexa-histidine (His) tag was introduced between NTF2 and the peptide tags both for purification of NTF2 and to introduce a spacer between the peptide tag and NTF2 to reduce any possible steric hindrance to target molecule binding. The location of the His-tag did not interfere with protein purification using Ni-NTA affinity chromatography, and engineered NTF2 was isolated at a yield of greater than 100 mg from 1 L cell culture after fast protein liquid chromatography (Fig. S3†). Based on the high expression levels of various engineered NTF2s, this approach is expected to generalize to a wide variety of different peptide tags.
Characterization of simplified NLP gel and engineered NTF2 system
Three important interactions affect the performance of the NLP/NTF2 system as a medium for selective biomolecular separations: target molecule binding to NTF2 via the peptide tag, NTF2 dimerization into its active form, and NTF2 interaction with FG repeats in the NLP gel. The strength of all these interactions will have a large effect on the minimum concentration at which the gels can be used to perform a separation. To simplify the kinetics where NTF2 captures target molecules in the catch-trap process, NTF2 that is covalently attached to the target green fluorescent protein (GFP) is initially considered as a model molecule to easily visualize the separation process using fluorescence microscopy. For native function, the naturally forming homodimer structure of NTF2 with Kd of 1.1 μM is crucial for binding between NTF2 and FG repeats.21 To explore how dimerization of NTF2 affects transport, we compare transport of NTF2-GFP at difference concentrations. At a concentration of 20 nM NTF2-GFP, no transport of cargo complexes is observed where fewer homodimer structures could be presented (Fig. 1). However, the addition of more NTF2 can shift the dimerization equilibrium towards the dimer. Thus, in the presence of an additional 19.98 μM of NTF2, transport of NTF2-GFP into the gel is clearly detected by the observation of a bright fluorescent band migrating inward from the surface of the gel in a capillary transport assay17 (Fig. 1). Because 20 μM of NTF2 is the naturally occurring concentration in NPCs,20 the total NTF2 concentration in this study is maintained at 20 μM or higher. Given the value of Kd for NTF2 dimerization, 73% of NTF2 is calculated to exist in the dimeric form at 20 μM (Fig. 1). In future work, the dissociation of the dimer can be circumvented preparing a mutant NTF2 where the two halves of the homodimer are chemically conjugated to maintain the dimerized state, independent of concentration.
Capillary transport experiments as a function of NTF2-GFP concentration show that the detectable minimum concentration for separation is approximately 2 nM in the environment. To enhance fluorescence intensity when imaging gels, NTF2-GFP molecules are labeled by fluorescein isothiocyanate (FITC) to yield FITC-NTF2-GFP (Fig. 2a and Fig. S4†). Various concentrations of FITC-NTF2-GFP while maintaining a total NTF2 concentration of 20 μM were prepared in the capillary together with 20 wt% NLP hydrogel (Fig. 2b). Stronger fluorescence intensity at the gel-buffer interface than the intensity of solution is detected immediately after the measurement with 200 nM FITC-NTF2-GFP and within 10 minutes at 20 nM concentrations, indicating the transport of FITC-NTF2-GFP into the gel. In the case of 2 nM FITC-NTF2-GFP, the fluorescence intensity at the gel interface appeared after one hour (Fig. 2c and d). The analysis of the fluorescence intensity at the gel interface clearly shows that the molecule is transported into the gel (Fig. 2d and Fig. S5†). Subnanomolar concentrations of FITC-NTF2-GFP do not lead to detectable transport into the gel in our current experimental condition (Fig. S5†). However, without FITC labeling of NTF2-GFP, even detection at 2 nM is not possible. Therefore, it is unclear whether the observed detection limit is a physical limit of the system's ability to transport cargo complexes or whether it represents the fluorescence detection limit of the instrumentation used to image the gels.
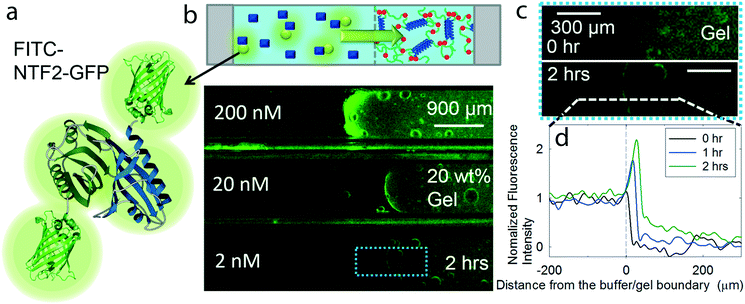 |
| Fig. 2 Concentration limits of biomolecular separation system. (a) FITC labeled NTF2-GFP to enhance the fluorescence intensity (Fig. S4†); (b) a schematic representing the contents in a capillary assay set-up, filled with NLP hydrogel and with various concentration of FITC-NTF2-GFP and 20 μM non-labeled NTF2 to promote NTF2 dimerization (Fig. 1) for enhanced binding between NTF2 and NLP gel (Fig. S5†); (c) magnified blue dotted box in (b) to investigate fluorescence intensity changes at the interface between the gel and the buffer which contains 2 nM GFP target molecules; (d) tracked normalized fluorescence intensity profiles along with the white dotted line of (c) in time series. | |
In addition to low concentration separations, a crucial function of the NPC-inspired separation system is the selective transport of target molecules into the gel while rejecting the passage of other inert molecules, particularly other proteins. To mimic the crowded protein environment of a biological fluid relevant to biomedical applications such as toxin separation from blood, solutions for selectivity tests are prepared with major components of blood plasma,28 containing 600 μM bovine serum albumin (BSA, ∼66 kg mol−1) and 167 μM immunoglobulin G (IgG, ∼150 kg mol−1), together with 0.2 μM FITC-NTF2-GFP and 19.8 μM NTF2 (Fig. 3a). BSA and IgG are labeled at a level of less than 1% with red fluorescent dye to avoid fluorescence intensity saturation. During the selectivity test, the effect of gel concentrations was also investigated since it might affect the pore size distribution as well as the average pore size. To quantify the selective properties of the gels, we define the percent accumulation of the target molecule from solution into the gel as the selective uptake and the selectivity of the gel as the ratio of accumulated target molecule vs. ratio of inert environmental molecules at the gel and the buffer. NLP hydrogels of 20 wt%, 10 wt% and 5 wt% can uptake 50 ± 6%, 39 ± 6% and 31 ± 4% of the FITC-NTF2-GFP, respectively, from this crowded bath solution in two hours (Fig. 3b and Fig. S6†). These results show that high concentration hydrogels can uptake more NTR-target molecule complexes from the environment, possibly due to an increase of FG binding sites within the gel.
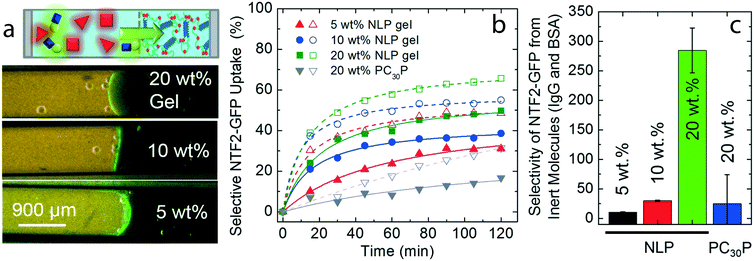 |
| Fig. 3 Selectivity of the separation system. (a) A schematic represents target molecule FITC-NTF2-GFP of 0.2 μM together with inert molecules, such as 600 μM BSA and 160 μM IgG. Less than 1% of BSA and IgG are labeled with red fluorescent dye to visualize the diffusion of inert molecules into the gel over time. The effects on molecular selectivity are also investigated with various gel concentrations and PC30P gel which do not have binding sites for NTF2; (b) average uptake of FITC-NTF2-GFP by the gels in the absence (empty objects) or in the presence (filled objects) of IgG and BSA. The uptake of IgG and BSA is given in Fig. S6;† (c) selectivity as a function of gel concentration and hydrogel types as calculated by eqn (1) and (2). | |
The selectivity of the target molecules in the gel, as calculated by eqn (1) and (2), dramatically improves at higher concentrations of polymer gel (Fig. 3c). To understand how many target molecules can be selectively absorbed by the gel from various environments, the percentage of absorbed target molecules and inert molecules in the gels is analyzed. When IgG and BSA depletion in the solution is measured by tracking changes in red fluorescence intensity, less than 1%, 2% and 4% of these proteins penetrate 20 wt%, 10 wt% and 5 wt% NLP hydrogels, respectively (Fig. 3b and Fig. S6†). The selectivity of 20 wt% gel is 284.6, 27 and 10 times greater than 5 wt% and 10 wt% gels which have the selectivity of 10.5 and 29.8, respectively (Fig. 3c; see experimental section for the calculation). The enhancement in selectivity is due to both improved transport of the NTF2-GFP fusion as well as lower permeation of BSA and IgG model impurities (Fig. 3c). It is interesting to note that, in recombinant Nsp1 gels, the selectivity is lost when the gel concentration is reduced;29 therefore, gels of 15–20 wt% are typically used.29–31 The results here for minimal consensus repeat NLPs are consistent with the behavior of the full length Nsp1. In addition, without the crowding environment, a larger percentage of FITC-NTF2-GFP was absorbed with values of 66 ± 9%, 55 ± 3% and 49 ± 1%, respectively. These results suggest that the presence of inert molecules can slow down the transport of target molecules into the gel probably due to the increase in viscosity.
A comparison between functional NLP gel and a similar gel with an inert C30 midblock17,19 confirms that the high selectivity of the 20 wt% NLP gel is due to the presence of hydrophobic FG repeats necessary for NTF2 binding and the enhanced barrier properties at higher gel concentrations. Artificially engineered C30 protein polymer has a similar molar mass to the NLP polymer and forms a hydrogel by the same association of its telechelic coiled-coil domains. In contrast, C30 gel does not contain FG repeats that interact with FITC-NTF2-GFP. The transport of FITC-NTF2-GFP into a 20 wt% C30 gel is three times smaller than into a 20 wt% NLP gel after 2 hours, confirming the role of the FG repeats in facilitating transport (Fig. 3b). However, the barrier function of the 20 wt% C30 gel for IgG and BSA resembles that of the 20 wt% NLP gel, rejecting more than 99% of the inert molecules (Fig. S6†). Higher polymer gel concentration can reduce the pore size of the network and narrow the pore size distribution, resulting in enhanced barrier properties from a purely physical origin.32 Altogether, the high selectivity of the NLP hydrogel seems to be achieved by enhanced NTF2-gel binding affinity by increasing FG repeats and by reducing the initial hydrogel pore size, which can reject the passage of other molecules, both of which are promoted at higher gel concentration.
Test of the catch-trap concept with a self-associating GFP model
As a proof of concept for the “catch-trap” design for biomolecular separation, NTF2 is prepared as a fusion to the GFP11 tag24,25,33 (an amino acid sequence that is the 11th strand in the GFP β-barrel), and GFP1–10 is separately synthesized as the target molecule (Fig. 4a and Fig. S3†). The non-fluorescent GFP1–10 can physically associate with GFP11 tag, enabling the complex to fold into the full GFP structure (GFP1–11) with green fluorescent emission. Incubation of NTF2-GFP11 and GFP1–10 for more than 24 hours allowed for binding to reach equilibrium (Fig. 4b), and approximately 10% of GFP1–10 assembled with NTF2-GFP11, NTF2-GFP1–11, based on GFP molar extinction coefficient (Fig. 4c). During the capillary assay together with the same concentrations of other inert molecules in Fig. 3, NTF2-GFP1–11 can selectively accumulate in the NLP gel (20 wt%). Because 90% of the non-fluorescent target GFP is not bound to NTF2, the diffusion of the non-fluorescent GFP into the NLP gel is difficult to track, making selectivity measurements difficult. Alternatively, we define the ratio of the relative increases in target molecules over other inert molecules from the gel/buffer interface to 500 μm inward to the gel as the near-gel interface selectivity. After 3 hours (Fig. 4e), the selectivity of near-gel interface shows 7 times greater concentration of NTF2-GFP1–11 in the gel than of the inert proteins (black dotted curve). This example shows that engineered NTRs can catch target molecules in the environment and the complexes can be selectively transported by NLP gel, demonstrating “catch-trap” transport of selected biomolecules.
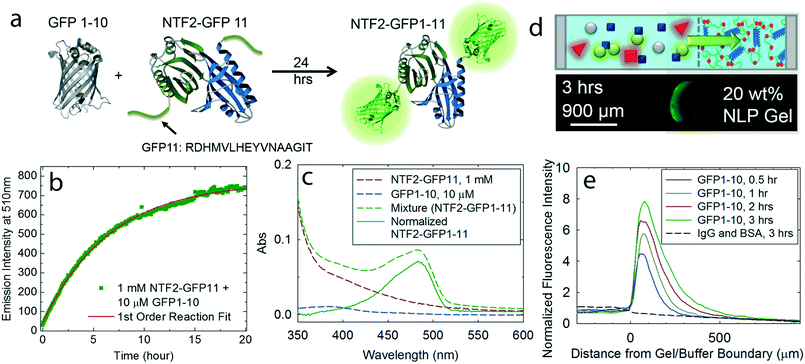 |
| Fig. 4 A proof of concept for “catch-trap” biomolecules using the NPC-inspired separation system; (a) NTF2 fused with GFP11 tag physically associates with GFP1–10, leading to the formation of the full GFP complex; (b) first order reaction fit on measurements of fluorescence intensity (λex = 485 nm) versus time indicates that 97% binding occurs in 23 hours; (c) optical absorbance of NTF2-GFP11, GFP1–10 and their mixture are measured and normalized (green solid curve). Based on the absorbance at 485 nm,37 it is determined that 10% GFP1–10 bound to NTF2-GFP11 and turned into NTF2-GFP1–11; (d) a capillary assay contains 20 wt% NLP gel and various molecules in the buffer, such as 1 μM GFP1–10 (gray), 100 μM NTF2-GFP11 (blue), 600 μM IgG (red) and 160 μM BSA (red); (e) normalized fluorescence intensity profiles of target molecules (solid lines) in time series and of IgG and BSA at 3 hours (black dotted line). | |
SEB toxoid absorption by NPC-inspired materials
As a practical example, staphylococcal enterotoxin B (SEB) toxoid, related to toxic shock syndrome and of human health concern as a potentially weaponized biotoxin,34 is used as the target molecule. As a first step, NTF2 was engineered with anti-SEB peptide tags, R418 and R445 which have higher SEB binding affinities than any other investigated peptides by Kogot et al.,15 and named NTF2-R418 and NTF2-R445 (Fig. 5a). Then, SEB separation is observed with the solution containing 50 μM engineered NTF2, 0.5 μM FITC labeled SEB, and the same concentration of inert molecules IgG and BSA as used for other selectivity assays (Fig. 3 and 4). One capillary assay is also prepared without engineered NTF2 as a control experiment to measure SEB transport in the absence of the transporter (Fig. 5b).
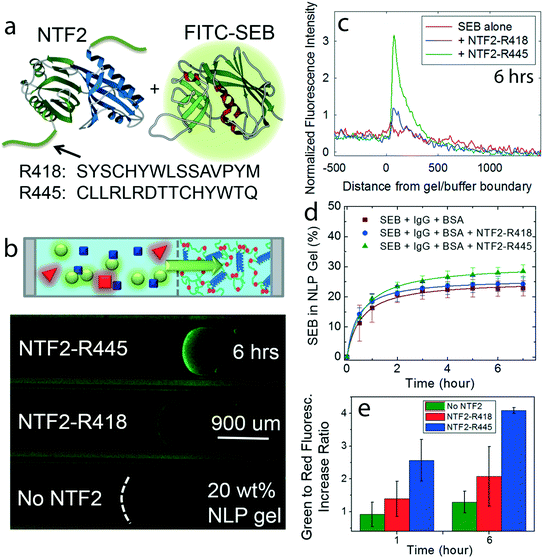 |
| Fig. 5 SEB toxoid separation by NPC-inspired system (a) a schematic presents engineered NTF2 fused with anti-SEB tags which can physically bind to SEB and SEB labeled with FITC to visualize the target transport under fluorescence microscopy; (b) in the capillary assays, buffers contain 0.5 μM FITC-SEB (green), 600 μM IgG (red), 160 μM BSA (red) and 50 μM engineered NTF2 (blue). A white dotted line indicates the interface between the buffer and the gel; (c) normalized fluorescence intensity profiles of SEB at 6 hours; (d) percentage of FITC-SEB transported to the gel calculated from the decrease in fluorescence in the buffer versus time (n = 3); (e) the fluorescence intensity plot (c) from 0 to 500 μm is integrated and the intensity enhancement in the same area for green fluorescence and red fluorescence is calculated in different time points compared to 0 hour. Ratios between FITC-SEB green fluorescence accumulation and IgG/BSA red accumulation in 1 hour and 6 hours are plotted (n = 3), in order to investigate the selectivity of the material system in the near-gel interface. | |
The analysis of fluorescence intensity profiles of SEB clearly showed that NTF2-R445 in solution concentrates SEB into the gel to a greater extent than NTF2-R418 which correspond with the binding affinity results by Kogot et al.,15 and both NTF2 fusions result in higher concentration in the gel than the absence of NTF2 (Fig. 5c). While facilitated transport of SEB carried by NTF2-anti-SEB tags is apparent, transport of unbound SEB also occurs into the NLP gel (Fig. 5d). When the selective uptake of SEB by the gel is measured, fluorescence intensities in all three cases plateau in six hours, and slight differences of SEB concentration in the gel are observed (SEB alone: 23 ± 3%, SEB with NTF2-R418: 24 ± 3% and SEB with NTF2-R445: 29 ± 2%; Fig. 5d). The similarities between the selective uptake of SEB and SEB–NTF2 complexes is due to the porosity of the NLP gel. Diffusion of free SEB occurs due to its small molar mass (28 kg mol−1, less than gel pore size of ∼30 kg mol−1 at the test 20 wt% gel concentration) and agrees with results obtained for FITC-Dextran of different molar masses.17 Therefore, while facilitated transport of SEB carried by NTF2-anti-SEB tags is apparent, transport of unbound SEB alone also occurs into the NLP gel.
Although smaller SEB and larger engineered NTF2 and SEB complexes can penetrate the gel in a similar amount, the analysis of fluorescence intensity profiles of SEB clearly shows that both NTF2 fusions result in higher SEB concentration in the near-gel interface than the absence of NTF2 (Fig. 5e). The near-gel interface selectivity of FITC-SEB compared to IgG and BSA after 6 hours is 2.08 ± 0.91, 4.09 ± 0.09 and 1.29 ± 0.33 in the presence of NTF2-R418, NTF2-R445 and no engineered NTF2, respectively (Fig. 5e). As expected (Fig. 5c), larger accumulation is observed in the presence of NTF2-R445 when compared to SEB alone and SEB with NTF2-R418, in agreement with different binding strengths between the peptide tags (Kd for R445 is smaller than for R418) and SEB,15 presenting that the SEB selectivity by the NLP gel occurred in the near-gel interface.
The results of SEB and GFP1–10 transport studies suggest certain design principles for a NPC-mimetic synthetic gel. First, the pore size of the gel should be smaller than the smallest molecule in the mix to be separated, minimizing non-interacting transport through the gel. Second, the NTR-target molecule binding should be as strong as possible, facilitating the formation of stable complexes for transport through the gel. Finally, the interaction between the transporter (e.g. NTF2) and the NLP gel should be of intermediate strength, since low binding constant would not be compatible with high specificity, whereas high affinity would lead to slow transport rates.35 Although the molecular mass of the engineered NTF2-SEB complex is about twice that of the free cargo (56 kg mol−1versus 28 kg mol−1, respectively), Fig. 5d suggests that the uptake rates by the gels are similar, showing that the NLP gels are not only selecting by size, but also by biochemical interactions. The multiple parameter selectivity is highly reminiscent of the complex selective interactions of the native NPC. Based on obtained information, the use of thin gel slabs, similarly to thin nuclear membrane, could be engineered for practical applications in order to achieve high filtration rates.
Conclusions
Inspired by the selective permeability of the NPC, engineered gels from nucleoporin-like proteins (NLPs) are combined with engineered NTRs fused with peptide tags to develop a novel selective biomolecular separation system. The system adds function to current filter technology, demonstrating a system where desired molecules larger than the filter size can nonetheless pass through the system, as well as maintaining the potential to operate in a continuous process, as opposed to well-established chromatographic methods. When the target is chemically fused to the NTF2, 20 wt% NLP hydrogel can detect nanomolar concentrations of target complex in the environment (Fig. 2). Moreover, the gels are able to selectively absorb the targets in the presence of high concentrations of other inert molecules, such as plasma protein surrogates in this study (Fig. 3). Proof of concept examples for NTF2 with binding tags that can catch and trap model targets GFP1–10 and SEB toxoid are presented (Fig. 4 and 5). By using engineered NTF2 fused with GFP11 tag and anti-SEB tags, we illustrate the versatility of the biomolecular separation system by introducing various peptide tags that can recognize specific biomolecules. Differing performance based on biomolecular size and binding affinity between the engineered NTR and target molecules also suggests specific design rules for mimicking the NPC separation capability in synthetic polymer systems. The ongoing development of diverse peptides for detection and purification, and as epitope tags for specific target molecules by other groups15,16,36 can be applied and expand our catch-trap system which promises applications in defense and biomedical areas, and food toxicology. In addition, the design rules investigated by this biosynthetic system may enable the engineering of new polymer constructs that do not require NTRs as intermediaries.
Acknowledgements
This work was supported by the Defense Threat Reduction Agency under contract HDTRA1-13-1-0038 (MK, WGC, BSS, and BDO), NIH 5-T32-GM008834 (fellowship for WGC) and Program Science without Borders, N. 234283/2014-9 (CNP q scholarship for BSS). We thank Katharina Ribbeck (MIT) for helpful discussions.
Notes and references
- T. S. Sileika, D. G. Barrett, R. Zhang, K. H. A. Lau and P. B. Messersmith, Angew. Chem., Int. Ed., 2013, 52, 10766–10770 CrossRef CAS PubMed.
- B. P. Lee, P. B. Messersmith, J. N. Israelachvili and J. H. Waite, Annu. Rev. Mater. Res., 2011, 41, 99–132 CrossRef CAS PubMed.
- S. C. Grindy, R. Learsch, D. Mozhdehi, J. Cheng, D. G. Barrett, Z. B. Guan, P. B. Messersmith and N. Holten-Andersen, Nat. Mater., 2015, 14, 1210–1216 CrossRef CAS PubMed.
- S. Lv, D. M. Dudek, Y. Cao, M. M. Balamurali, J. Gosline and H. B. Li, Nature, 2010, 465, 69–73 CrossRef CAS PubMed.
- J. Chung, A. M. Kushner, A. C. Weisman and Z. B. Guan, Nat. Mater., 2014, 13, 1055–1062 CrossRef CAS PubMed.
- S. R. MacEwan and A. Chilkoti, J. Controlled Release, 2014, 190, 314–330 CrossRef CAS PubMed.
- K. E. Knockenhauer and T. U. Schwartz, Cell, 2016, 164, 1162–1171 CrossRef CAS PubMed.
- T. A. Douglas, D. Tamburro, C. Fredolini, B. H. Espina, B. S. Lepene, L. Ilag, V. Espina, E. F. Petricoin, L. A. Liotta and A. Luchini, Biomaterials, 2011, 32, 1157–1166 CrossRef CAS PubMed.
- M. Tagliazucchi and I. Szleifer, Mater. Today, 2015, 18, 131–142 CrossRef CAS.
- R. Bayliss, T. Littlewood and M. Stewart, Cell, 2000, 102, 99–108 CrossRef CAS PubMed.
- K. Ribbeck and D. Gorlich, EMBO J., 2001, 20, 1320–1330 CrossRef CAS PubMed.
- D. Mohr, S. Frey, T. Fischer, T. Guttler and D. Görlich, EMBO J., 2009, 28, 2541–2553 CrossRef CAS PubMed.
- M. Stewart, Nat. Rev. Mol. Cell Biol., 2007, 8, 195–208 CrossRef CAS PubMed.
- D. Gorlich and U. Kutay, Annu. Rev. Cell Dev. Biol., 1999, 15, 607–660 CrossRef CAS PubMed.
- J. M. Kogot, J. M. Pennington, D. A. Sarkes, D. A. Kingery, P. M. Pellegrino and D. N. Stratis-Cullum, J. Mol. Recognit., 2014, 27, 739–745 CrossRef CAS PubMed.
- S. R. Crowe, L. L. Ash, R. J. Engler, J. D. Ballard, J. B. Harley, A. D. Farris and J. A. James, J. Infect. Dis., 2010, 202, 251–260 CrossRef CAS PubMed.
- M. Kim, W. G. Chen, J. W. Kang, M. J. Glassman, K. Ribbeck and B. D. Olsen, Adv. Mater., 2015, 27, 4207–4212 CrossRef CAS PubMed.
- W. Shen, K. Zhang, J. A. Kornfield and D. A. Tirrell, Nat. Mater., 2006, 5, 153–158 CrossRef CAS PubMed.
- B. D. Olsen, J. A. Kornfield and D. A. Tirrell, Macromolecules, 2010, 43, 9094–9099 CrossRef CAS PubMed.
- C. Chaillan-Huntington, C. V. Braslavsky, J. Kuhlmann and M. Stewart, J. Biol. Chem., 2000, 275, 5874–5879 CrossRef CAS PubMed.
- C. Chaillan-Huntington, P. J. Butler, J. A. Huntington, D. Akin, C. Feldherr and M. Stewart, J. Mol. Biol., 2001, 314, 465–477 CrossRef CAS PubMed.
- R. Bayliss, S. W. Leung, R. P. Baker, B. B. Quimby, A. H. Corbett and M. Stewart, EMBO J., 2002, 21, 2843–2853 CrossRef CAS PubMed.
- R. Bayliss, K. Ribbeck, D. Akin, H. M. Kent, C. M. Feldherr, D. Gorlich and M. Stewart, J. Mol. Biol., 1999, 293, 579–593 CrossRef CAS PubMed.
- S. Cabantous, T. C. Terwilliger and G. S. Waldo, Nat. Biotechnol., 2005, 23, 102–107 CrossRef CAS PubMed.
- S. Cabantous and G. S. Waldo, Nat. Methods, 2006, 3, 845–854 CrossRef CAS PubMed.
- K. P. Kent, W. Childs and S. G. Boxer, J. Am. Chem. Soc., 2008, 130, 9664–9665 CrossRef CAS PubMed.
- J. D. Pedelacq, S. Cabantous, T. Tran, T. C. Terwilliger and G. S. Waldo, Nat. Biotechnol., 2006, 24, 79–88 CrossRef CAS PubMed.
- E. A. Vogler, Biomaterials, 2012, 33, 1201–1237 CrossRef CAS PubMed.
- S. Frey and D. Gorlich, Cell, 2007, 130, 512–523 CrossRef CAS PubMed.
- A. A. Labokha, S. Gradmann, S. Frey, B. B. Hulsmann, H. Urlaub, M. Baldus and D. Görlich, EMBO J., 2013, 32, 204–218 CrossRef CAS PubMed.
- C. Ader, S. Frey, W. Maas, H. B. Schmidt, D. Görlich and M. Baldus, Proc. Natl. Acad. Sci. U. S. A., 2010, 107, 6281–6285 CrossRef CAS PubMed.
- C. Kotsmar, T. Sells, N. Taylor, D. E. Liu, J. M. Prausnitz and C. J. Radke, Macromolecules, 2012, 45, 9177–9187 CrossRef CAS.
- K. P. Kent, W. Childs and S. G. Boxer, J. Am. Chem. Soc., 2008, 130, 9664–9665 CrossRef CAS PubMed.
- J. M. Rusnak, M. Kortepeter, R. Ulrich, M. Poli and E. Boudreau, Emerging Infect. Dis., 2004, 10, 1544–1549 CrossRef PubMed.
- S. Milles, D. Mercadante, I. V. Aramburu, M. R. Jensen, N. Banterle, C. Koehler, S. Tyagi, J. Clarke, S. L. Shammas, M. Blackledge, F. Grater and E. A. Lemke, Cell, 2015, 163, 734–745 CrossRef CAS PubMed.
- D. W. Wood, Curr. Opin. Struct. Biol., 2014, 26, 54–61 CrossRef CAS PubMed.
- J. D. Pedelacq, S. Cabantous, T. Tran, T. C. Terwilliger and G. S. Waldo, Nat. Biotechnol., 2006, 24, 79–88 CrossRef CAS PubMed.
Footnotes |
† Electronic supplementary information (ESI) available. See DOI: 10.1039/c7me00006e |
‡ Present address: Department of Materials Science and Engineering, Department of Biomedical Engineering, The University of Arizona, Tucson, AZ 85721, USA. |
|
This journal is © The Royal Society of Chemistry 2017 |