DOI:
10.1039/C6MD00474A
(Research Article)
Med. Chem. Commun., 2017,
8, 158-161
67Ga-metalloprobes: monitoring the impact of geometrical isomers on accumulation profiles in rat cardiomyoblasts and human breast carcinoma cells†
Received
18th August 2016
, Accepted 10th October 2016
First published on 9th November 2016
Abstract
Geometrically similar monocationic gallium(III) complexes and their radiolabeled SPECT counterparts were obtained from Schiff base precursor ligands using ligand exchange reactions to evaluate the impact of cis and trans-isomers on their cellular accumulation profiles in rat cardiomyoblasts (H9c2(2-1)) and human breast carcinoma (MCF-7neo) cells. 67Ga-metalloprobes comprising trans-phenolates showing an overall octahedral geometry and exhibiting uniform spatial distribution of positive charges on their molecular surface show steady-state accumulation in H9c2(2-1) and MCF-7neo cells, and localize in the mitochondria of the cells. Importantly, the surrogate geometrically similar and monocationic metalloprobe counterparts possessing the cis arrangement of phenolates do not show cellular uptake in H9c2(2-1) and MCF-7neo cells. Exploiting their modest fluorescent traits, live cell imaging indicates that trans-isomers of metalloprobes localize within the mitochondria of cells following their penetration, thereby indicating the excellent correlation of radiotracer data and live-cell microscopy results. Overall, these results indicate that the cell uptake profiles of metalloprobes within this class are mediated by the spatial distribution of charges over their molecular surface and hydrophobicity.
Introduction
Radiolabeled metalloprobes have been shown to provide versatile tools for non-invasive imaging of coronary artery disease,1–3 tumors,4–8 and cerebral blood perfusion.9 Over the last two decades, cationic and moderately hydrophobic metalloprobes comprising a selected class of mixed ligands10 and Schiff-base ligands11 have been known to be useful for monitoring the functional expression of P-glycoprotein (Pgp)11,12 and breast cancer resistance protein (BCRP),13,14 the well-characterized barriers of chemotherapeutic resistance, located on the plasma membrane of tumor cells. Recently, we have reported preclinical validation of Galmydar, a PET tracer incorporated with a generator produced radionuclide gallium-68, and demonstrated its potential for imaging myocardial perfusion3 and functional expression of the ABC family of transporters.15 Others16–18 and we have also demonstrated that the presence of an overall cationic charge on metalloprobes mediates their localization within the mitochondria of penetrated cells19,20 and pharmacokinetic profiles in vivo.13,14 However, the relationship between the spatial distribution of charges on their molecular surface resulting from preferential geometry including different geometrical isomers and their overall impact upon penetration of metalloprobes into myocardium and tumor cells has not been ascertained. To evaluate, whether or not spatial charge distribution on the molecular surface of metalloprobes could play an important role in determining their permeation within tumor cells, we report the synthesis and characterization of chemically similar yet geometrically different isomers of monocationic metalloprobes, and determine their cellular accumulation profiles within cardiomyoblasts and tumor cells, using radiotracer and fluorescence bioassays. Herein, we demonstrate that the cellular uptake profiles of cationic metalloprobes are dependent upon geometrical isomers (within this class) contributing to the differential distribution of positive charges on their molecular surface thus mediating penetration within cells.
Results and discussion
For evaluation, six moderately hydrophobic and cationic gallium(III) metalloprobes were synthesized, using ligand-exchange reactions involving gallium(III) acetylacetonate and their corresponding heptadentate Schiff-base precursor ligands (Fig. 1). For evaluating representative example of chemical structures in the solid state, the crystal structures of Schiff-base gallium(III) complexes (5a and 8a) were determined. To accomplish this aim, crystals of 5a and 8a3 were obtained by slow evaporation of methanol at room temperature over several days. The gallium(III) complex 5a crystallized in the monoclinic space group P21/c. The ORTEP drawing showing the crystallographic numbering scheme for 5a is illustrated in Fig. 2. The crystal data, including refinement parameters, and significant bond angles as well as inter-atomic distances are given in Tables 1 and 2 (ESI†). We have recently reported the crystal data for 8a.3 The crystal data demonstrated the presence of an overall octahedral geometry for both 5a and 8a3 metalloprobes, encompassing the N4O2 donor core (two phenolates, two nitrogen atoms of salicylaldimine, and two secondary nitrogen atoms of the amino group of ethylenediamine). While the incorporation of these donor core atoms with gallium in 5a resulted in the cis-configuration of phenolates and composition of two six-membered and three five-membered rings, the trans configuration of phenolates around the central gallium atom in 8a indicated the formation of one five-membered and 4 six-membered rings involving the gallium atom.3 Further, both 1H NMR and proton-decoupled 13C NMR spectra of 5a and 8a recorded in methanol-d4 at room temperature demonstrated the existence of a two-fold symmetry in the solution state thus correlating with the crystal data in the solid state (X-ray data). These data are also in accord with other metal complexes of related ligands.14,21–26
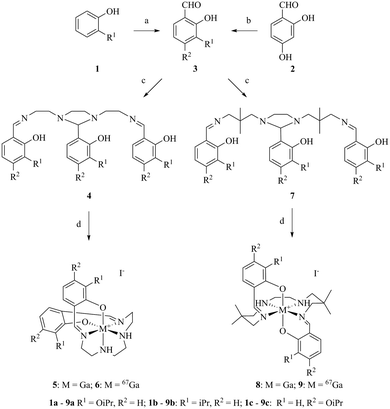 |
| Fig. 1 Reagents and conditions: (a) MgCl2, Et3N, ACN, (HCHO)n, 82 °C 5 h; (b) K2CO3, (CH3)2CHBr, DMF, 25–100 °C 15 h; (c) (CH2NHCH2C(CH3)2CH2NH2)2/(CH2NHCH2CH2NH2)2, EtOH, 78 °C, 45 min; (d) Ga(acac)3, KI/67Ga(acac)3, MeOH, water, 110 °C, 40 min–3 h. | |
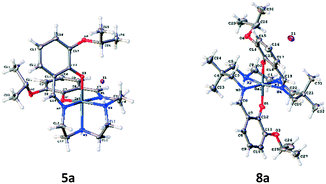 |
| Fig. 2 Projection view of cationic gallium(III) complexes 5a and 8a3 showing the crystallographic numbering scheme. Atoms are represented by thermal ellipsoids corresponding to 50% probability. | |
For performing cell bioassays, radiolabeled 67Ga(III)-metalloprobes (6 and 9) were prepared using 67Ga(III)-acetylacetonate and ligands (4 and 7) in ethanol at 100 °C for 40 min (Fig. 1), using procedures described earlier by others and our laboratories with some modifications.3,14 To perform the bioassays, 67Ga(III)-metalloprobes with a sufficiently long half-life (gallium-67; t1/2 = 78.2 h) were used to enable multiple cellular accumulation assays with the same HPLC purified fraction to allow comparative analysis. The formation of 67Ga(III)-metalloprobes (6 and 9) was monitored via radio-TLC (Rf: 0.23–0.31) in a methanol–saline (90
:
10) mixture, and the reactions were found to be >95% complete. Finally, the radiolabeled products were purified over a C-18 column via a radio-HPLC equipped with a γ-detector and a dual λ detector, employing a gradient eluent mixture of ethanol and saline. The fractions eluting at retention times of 16.8–17.6 min were collected, concentrated, re-suspended in saline containing 10% ethanol, and finally diluted further with media to <0.1% ethanol to perform cellular accumulation assays.
Following incubation of 67Ga-metalloprobes in H9c2(2-1), 9a (438.30 fmol nM0−1 mg protein−1) showed 17-fold greater accumulation compared to 6a (25.12 fmol nM0−1 mg protein−1). Similarly, 9a (389.76 fmol nM0−1 mg protein−1) also showed 49-fold greater accumulation compared to 6a (7.97 fmol nM0−1 mg protein−1) in MCF-7neo cells (Fig. 3). Similar differentiation in uptake profiles was also observed in other 67Ga-metalloprobes (9b and 6b; 9c and 6c). Although radiotracer bioassays provide sensitive and quantifiable uptake data, this technique provides information on population of cells rather than direct information at a single cell level including subcellular localization in live cells. To confirm cellular accumulation in a single live cell, mildly fluorescent molecules 5a and 8a were incubated at 37 °C for 30 min under a continuous flux of 5% CO2, and cellular uptake was analyzed using fluorescence imaging. Ga(III)-complex 8a (20 μM) penetrated cells and showed stable accumulation in rat cardiomyoblasts. To probe its intracellular localization, cardiomyoblasts were also incubated with 8a (20 μM) and MitoTracker Red CM-H2XRos (25 nM, Invitrogen) under identical conditions. The metalloprobe 8a demonstrated localization in the mitochondria of cardiomyoblasts and a nearly close correlation with MitoTracker Red CMH2XRos (Fig. 4, top panel). Conversely 5a demonstrated insignificant accumulation within cardiomyoblasts (Fig. 4, lower panel), indicating the impact of the geometrical preference in mediating cellular accumulation profiles, prevalent within this class of metalloprobes.
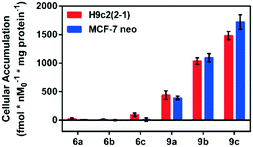 |
| Fig. 3 Cellular accumulation of cationic 67Ga-gallium(III) Schiff-base complexes (6a–c, 9a–c) in H9c2(2-1) and MCF-7neo cells as indicated. Shown is the net uptake at 90 minutes (fmol nM0−1 mg protein−1) using the control buffer. Each bar represents the mean of 4 determinations; the line above the bar denotes ±SD. | |
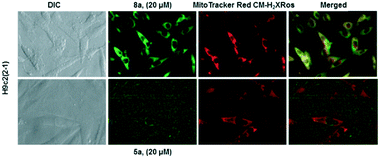 |
| Fig. 4 Cellular accumulation of metalloprobes and confirmation of their intracellular localization via correlation with Mito-Tracker: fluorescence imaging in live H9c2(2-1) cells following 30 min treatment with gallium(III) complexes (5a, lower panel; and 8a, top panel; 20 μM) and Mito-Tracker (25 nM). Note that, while 8a penetrates H9c2(2-1) cells, localizes within the mitochondria, and its localization correlates with the Mito-tracker, 5a does not show significant uptake under identical conditions. Similar results were obtained in MCF-7neo cells (data not shown). | |
To understand whether or not the differential cellular uptake profiles of both classes of compounds were mediated by differences in spatial distribution of charges on the molecular surface resulting from geometrical isomers, arising from the encapsulation of the central metal by the donor core of the scaffold, we further assessed the electrostatic potential map, dipole moment and polarizability, using the molecular modeling software Spartan version 10. Literature precedents indicate that Spartan 10 can allow fairly accurate comparative analysis of molecular properties of CNS drugs in relation to their BBB permeability profiles.27 Using Spartan version 10 and crystal data for 5a and 8a, energetically favored geometrical configurations of donor atoms around the central metal were determined, and electrostatic potential maps were obtained, wherein red color depicts the most negative region, while the blue color indicates the most positive in an order: red < orange < yellow < green < blue. The electrostatic potential map demonstrated a fairly uniform distribution of positive charges on the molecular surface of 8a in comparison with that of 5a, which in turn contributes to large differences in net dipole moments (8a: 2.39; 5a: 14.39 debye). Furthermore, the net polarizability of metalloprobe 5a has been found to be 2-fold higher than that of 8a (8a: 39.63; 5a: 81.36) (Fig. 5). Finally, we also measured log
P (octanol/saline partition coefficient) values for all synthesized compounds. Importantly, the log
P values for 8a and 5a were found to be 1.0 and 0.2, respectively.
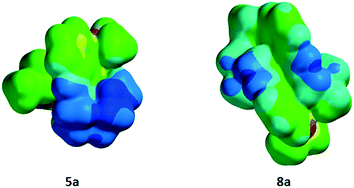 |
| Fig. 5 Space filling models of cationic gallium(III) Schiff-base complexes showing encapsulation of the central gallium(III) atom by the donor core of ligands resulting in an octahedral geometry, including the distribution of electronic charges using the molecular modelling program Spartan. The uniform delocalization of the positive charge on the surface of 8a is consistent with its penetration into cells and localization within the mitochondria compared with simple separation of charges resulting in a salt-like effect of 5a thus impeding its penetration into cells. | |
Conclusions
In conclusion, moderately hydrophobic and monocationic cis and trans octahedral gallium(III) metalloprobes were synthesized, characterized, and evaluated for cellular penetration into rat myocardium and human breast cancer cells. While sharing the overall octahedral arrangement of the donor core around the central metal, addition of an extra methylene group in the hydrocarbon backbone created two cis and trans isomers involving phenolates. Cell bioassays indicate that octahedral complexes possessing the trans configuration of phenolates penetrate cell bilayers in order to localize in the mitochondria, while the cis isomers did not display significant uptake under identical conditions. Combined data indicate that the differential cellular uptake profiles of gallium(III) Schiff-base complexes into rat cardiomyoblasts and human breast carcinoma cells are driven in part by variations in the spatial distribution of charges on the molecular surface of geometrical isomers and associated hydrophobicity.
Acknowledgements
The authors thank Prof. David Piwnica-Worms (MD Anderson, University of Texas, Houston, TX) for helpful discussions. Financial assistance to this work was provided by grants from NIH grants RO1 HL111163 (VS) and R33 AG033328 (VS).
References
- W. C. Eckelman, JACC Cardiovasc. Imaging, 2009, 2, 364 CrossRef PubMed.
- A. L. Baggish and C. A. Boucher, Circulation, 2008, 118, 1668 CrossRef PubMed.
- V. Sharma, J. Sivapackiam, S. E. Harpstrite, J. L. Prior, H. Gu, N. P. Rath and D. Piwnica-Worms, PLoS One, 2014, 9, e109361 Search PubMed.
- D. Brasse and A. Nonat, Dalton Trans., 2015, 44, 4845 RSC.
- E. Gourni, C. Canovas, V. Goncalves, F. Denat, P. T. Meyer and H. R. Maecke, PLoS One, 2015, 10, e0145755 Search PubMed.
- F. C. van de Watering, M. Rijpkema, L. Perk, U. Brinkmann, W. J. Oyen and O. C. Boerman, BioMed. Res. Int., 2014, 2014, 203601 CrossRef PubMed.
- S. E. Harpstrite, H. Gu, R. Natarajan and V. Sharma, Nucl. Med. Commun., 2014, 35, 1067 CrossRef CAS PubMed.
- J. S. Lewis and C. J. Anderson, Methods Mol. Biol., 2007, 386, 227 CAS.
- F. J. Bonte, L. Hynan, T. S. Harris and C. L. White, Int. J. Mol. Imaging, 2011, 2011, 409101 CrossRef PubMed.
- G. Luker, C. Crankshaw, M. Marmion, B. Burleigh, E. Deutsch and D. Piwnica-Worms, Proc. Am. Assoc. Cancer Res., 1996, 37, 317 Search PubMed.
- J. Sivapackiam, S. T. Gammon, S. E. Harpstrite and V. Sharma, Methods Mol. Biol., 2010, 596, 141 CAS.
- V. Sharma, Bioconjugate Chem., 2004, 15, 1464 CrossRef CAS PubMed.
- V. Sharma, J. Prior, M. Belinsky, G. Kruh and D. Piwnica-Worms, J. Nucl. Med., 2005, 46, 354 CAS.
- J. Sivapackiam, S. E. Harpstrite, J. L. Prior, H. Gu, N. P. Rath and V. Sharma, Dalton Trans, 2010, 39, 5842 RSC.
- J. Sivapackiam, S. E. Harpstrite, J. L. Prior, S. Mattingly and V. Sharma, Nucl. Med. Biol., 2016, 43, 191 CrossRef CAS PubMed.
- P. C. Bruijnincx and P. J. Sadler, Curr. Opin. Chem. Biol., 2008, 12, 197 CrossRef CAS PubMed.
- J. L. Hickey, R. A. Ruhayel, P. J. Barnard, M. V. Baker, S. J. Berners-Price and A. Filipovska, J. Am. Chem. Soc., 2008, 130, 12570 CrossRef CAS PubMed.
- C. T. Yang, Y. Li and S. Liu, Inorg. Chem., 2007, 46, 8988 CrossRef CAS PubMed.
- S. Davis, M. Weiss, J. Wong, T. Lampidis and L. Chen, J. Biol. Chem., 1985, 260, 13844 CAS.
- G. Sundaram, M. Sharma, D. Kaganov, J. J. Cho, S. E. Harpstrite and V. Sharma, J. Inorg. Biochem., 2016, 159, 159 CrossRef CAS PubMed.
- M. Fellner, W. Dillenburg, H. G. Buchholz, N. Bausbacher, M. Schreckenberger, F. Renz, F. Rosch and O. Thews, Mol. Imaging Biol., 2011, 13, 985 CrossRef PubMed.
- Y. M. Hsiao, C. J. Mathias, S. P. Wey, P. E. Fanwick and M. A. Green, Nucl. Med. Biol., 2009, 36, 39 CrossRef CAS PubMed.
- S. Harpstrite, J. Prior, N. Rath and V. Sharma, J. Inorg. Biochem., 2007, 101, 1347 CrossRef CAS PubMed.
- E. Wong, S. Liu, T. Lugger, F. E. Hahn and C. Orvig, Inorg. Chem., 1995, 34, 93 CrossRef CAS.
- B. Tsang, C. Mathias, P. Fanwick and M. Green, J. Med. Chem., 1994, 37, 4400 CrossRef CAS PubMed.
- B. Tsang, C. Mathias and M. Green, J. Nucl. Med., 1993, 34, 1127 CAS.
- H. Kim, S. Sulaimon, S. Menezes, A. Son and W. Menezes, J. Chem. Educ., 2011, 88, 1389 CrossRef CAS.
Footnote |
† Electronic supplementary information (ESI) available: Experimental method. CCDC 1496192. For ESI and crystallographic data in CIF or other electronic format see DOI: 10.1039/c6md00474a |
|
This journal is © The Royal Society of Chemistry 2017 |