DOI:
10.1039/C6LC01263A
(Paper)
Lab Chip, 2017,
17, 169-177
Rapid detection of Hendra virus antibodies: an integrated device with nanoparticle assay and chaotic micromixing†
Received
12th October 2016
, Accepted 20th November 2016
First published on 24th November 2016
Abstract
Current diagnosis of infectious diseases such as Hendra virus (HeV) relies mostly on laboratory-based tests. There is an urgent demand for rapid diagnosis technology to detect and identify these diseases in humans and animals so that disease spread can be controlled. In this study, an integrated lab-on-a-chip device using a magnetic nanoparticle immunoassay is developed. The key features of the device are the chaotic fluid mixing, achieved by magnetically driven motion of nanoparticles with the optimal mixing protocol developed using chaotic transport theory, and the automatic liquid handling system for loading reagents and samples. The device has been demonstrated to detect Hendra virus antibodies in dilute horse serum samples within a short time of 15 minutes and the limit of detection is about 0.48 ng ml−1. The device platform can potentially be used for field detection of viruses and other biological and chemical substances.
1 Introduction
Hendra virus (HeV) is a form of Henipavirus, the deadliest human pathogens within the Paramyxoviridae family.1 It is a single-stranded, negative-sense RNA virus that can cause respiratory and/or encephalitic diseases in many mammalian species such as humans, horses, pigs, dogs, cats and so on.2 It was first discovered in Northern Australia in 1994.3 Since then, it has caused several deaths in humans and has a mortality rate of 80% in horses.4,5 In addition, the potential for HeV to be used as a biological warfare agent targeting either livestock or humans has been documented.6 Due to the lethality and potential epidemic of the disease, rapid diagnosis of HeV infection is critically important in order to understand where outbreaks are occurring and to prevent the spread of HeV. However, the detection of HeV currently relies on laboratory-based tests such as PCR, DNA sequencing, virus isolation/characterization and serology.1,2,7,8 Depending on the location of the outbreak and the distance from the nearest PC4 (Physical Containment Level 4) biological laboratory, these tests may take 24–48 hours or more to become available, which is an unacceptably long time. Further, high level containment facilities (e.g. PC4 laboratories) are rarely available, which prevents the tests to be carried out. Recently, the Luminex ELISA assay9 has been used widely for HeV antibody detection. However, this method takes several hours to complete and requires many steps of laboratory procedures and thus is not suitable as a diagnostic tool for field deployment. Clearly, there is an urgent need for rapid diagnosis of HeV infection so that health officials can ascertain if and where outbreaks are occurring and implement subsequent measures to prevent further spread of the disease.
To detect and combat an emerging outbreak, the most appropriate means would be point-of-care (POC) diagnostics because it would obtain results at the point of sampling and allow for large-scale screening. The ideal POC device would have the following key features: automatic raw sample and reagent processing, portability, simple operating procedures, rapid and accurate generation of diagnostic results, and low cost in device and/or analysis per sample.
Microfluidic technologies have the potential to address these requirements. Over the last decade, there have been extensive efforts to develop microfluidic-based devices for POC virus detection and disease diagnosis.10–13 Among these developments, magnetic particle-based techniques have been commonly used due to the advantages particles have such as high surface-to-volume ratio for high efficiency bio-chemical reactions, the availability of a range of chemistries for their functionalization and on-chip control techniques for their mixing, separation and transport.14–25 For example, Lum et al.21 developed a microfluidic platform for the rapid detection of avian influenza virus based on immunomagnetic nano-beads and non-Faradaic impedance measurement with a detection time around 2 hours. In order to reduce non-specific binding, Hung et al.23 chemically modified the surface of magnetic nanoparticles to carry a positive charge and used micromixing to increase the reaction rate. An integrated microfluidic device has been developed for influenza virus detection with a limit of detection as low as 0.007 HAU and a detection time of 20 min. Lisi et al.26 proposed a protocol for detection of HeV based on a monoclonal antibody conjugated to magnetic beads and quantum dots and suggested that it could be implemented on a microfluidic POC device.
In addition to the control of on-chip assay steps of separation and retention, magnetic beads have been used in previous studies to enhance the mixing of reagents to speed up the detection.27–33 For example, Rida and Gijs27 introduced the concept of a magnetic micromixer based on self-assembled aggregates of magnetic beads, also known as magnetorheological structures. These porous structures were held in position and induced to rotate by AC magnetic fields, making the porous properties of the structure dynamic and inducing a vortex-like motion of the fluid in the microchannel to enhance mixing. Similarly, others32,33 have set clumps of magnetic beads rotating through the imposition of an external, steady rotating magnetic field. Liquid streams passing by the rotating clumps are mixed in close analogy to how a magnetically rotated stirring bar mixes in a benchtop beaker. In a different study, Wang et al.28 positioned two electromagnets on either side of a microchannel and periodically activated them to move single magnetic particles flowing down the microchannel. They attributed the mixing enhancement to the alternating transverse motion of the particles' increasing interaction with the surrounding fluid and found that the mixing efficiency depended on the force and switching frequency of the magnets.
However, the previously reported devices have drawbacks of either low speed, low sensitivity or design complexity that limit their commercialisation potential. Further, most of the lab-on-a-chip devices developed do not integrate liquid (samples and reagents) handling systems and therefore can only be used in laboratory settings. Furthermore, to the best of our knowledge, no lab-on-a-chip device has been developed so far for POC detection of HeV. Therefore, the main objective of this study is to develop an integrated lab-on-a-chip device to fill the technology gap. Here, we demonstrate a new microfluidic platform that utilises magnetic beads to achieve chaotic mixing of infected blood sample fluids with reagents and detects Hendra virus antibodies with fast speed. Superparamagnetic beads are used for both actuating chaotic fluid motion based on periodically reoriented flows and providing reactive surfaces for the two-step magnetic immunosorbent assay for HeV antibody detection. The whole assay can be completed in approximately 15 minutes with a high detection sensitivity.
2 Experiment
2.1 Microfluidic device and operation
The lab-on-a-chip device designed here includes the features of automatic handling of all liquids, mixing and assay product detection. Fig. 1(A) shows a schematic drawing of these features. Also shown in the figure are a photograph of the internal hardware of the built device (Fig. 1(B)) and two magnetic electrode configurations (Fig. 1(C)).
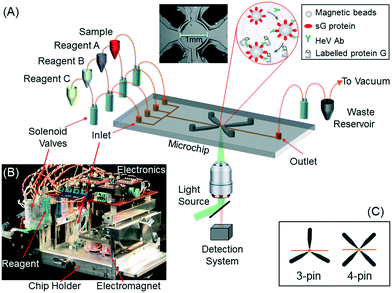 |
| Fig. 1 Principle and some details of the microfluidic device for HeV antibody detection. (A) A schematic drawing of the microchip and liquid handling system. The mixing chamber surrounded with four magnetic pins is used to perform the multiple-step assay (i.e. the left image of the top inset). The outer ends of the four magnetic pins are flush with the top surface of the microchip and touch-connected to four electromagnets (refer to the picture in (B)) for delivering magnetic fields into the chamber. The four inlets on the left side of the microchip are used for connecting with the four liquid solutions (i.e. the four colored tubes in the picture of (B)). Reagents A, B and C are the buffer, magnetic bead solution and R-PE dye solution, respectively. Vacuum pressure is applied through the waste port to pump the liquids. The top insets show a picture of the mixing chamber with four magnetic pins (left) and the principle of the bioassay for this study. The scale bar indicates a length of 1 mm. (B) A picture of the built device with a microchip loading and holder system (aluminum plates) and embedded controls. (C) The bottom right inset shows two arrangements of the magnetic pins. | |
The liquid handling module incorporates four liquid vials, each storing a separate liquid, a waste liquid chamber, and a pumping and actuation system. The four liquid vials are necessary to handle the four solutions required to perform the HeV detection assay, i.e. the blood sample, magnetic bead (MB) solution, washing buffer and a fluorescent label, i.e. R-PE solution. The liquid pumping is realised by applying vacuum pressure through the waste port using a miniaturised vacuum pump, and the actuation of pumping for each line is controlled by inline solenoid valves (Dyne Industries Pty Ltd, Bayswater Victoria, Australia). The four inlet ports allow for introduction of the four liquids into the microchip, either individually or simultaneously. The outlet port is connected to a waste chamber. The vacuum pump, the solenoid valves and the electromagnets are all powered by 20 V DC power supplies. The switching of power for these components is controlled by in-house built microcontroller boards and Labview software. A liquid pumping video and additional figures are provided in the ESI.†
The microfluidic module consists of a microfluidic chip and a loading mechanism. The microchip is designed to perform multiple step assays and fluorescence detection in one single chamber. It has the dimensions of 75 mm × 50 mm and has four liquid inlet ports and one outlet port, each being connected to the liquid vials and waste chamber in the liquid handling module. These liquid ports are connected to the mixing chamber via a network of microchannels with a cross-section of 100 μm × 120 μm. The mixing chamber is used to perform the biochemical assays and the subsequent detection. The height of the chamber is defined by the microchannel height which is 100 μm while the diameter is chosen to be 1 mm. The latter is based on the compromise between the requirements of increasing detection sensitivity with a larger detection volume while limiting the reagent usage and collection optics.34,35 Magnetic pins, with a 1 mm × 1 mm square tip, were embedded into the PDMS microchip structure at a distance of 500 μm from the chamber edge. The magnetic pins were precision-machined from small steel rods into a needle shape with a tapered change at the ends. The size of the end cross-section is 1 mm × 1 mm. Two mixing configurations were used for the current study, one with 3 pins and the other with 4 pins (see the inset of Fig. 1). Most of the studies were carried out using the 4-pin microchip.
During the operation of the device, the microchip is placed in the chip holder (i.e. the aluminum block at the bottom of the device as shown in Fig. 1B) which slides into the device housing. Once the microchip is positioned, the locking system connects the microchip liquid ports to the liquid handling system. The placement of the microchip also connects the magnetic pins to the electromagnets fixed in the device housing (Fig. 1B). The electromagnets are the source of the magnetic fields delivered to the mixing chamber via the conductor pins. A ratiometric, linear, Hall-effect sensor (Allegro MicroSystems, Inc.) was used to measure the magnetic flux density at different distances from the magnetic conductor tip end. At a distance of 1 mm, the magnetic flux density was 400 G (DC: 10 V, 280 mA).
Once loaded, the device is designed to require minimal user intervention. The main operating procedures are as follows.
1. Reagent and sample loading – placing reagent and sample vials into the device.
2. Microchip loading – placing the microchip onto the chip holder, sliding into the device and locking the microchip into position.
3. Performing assay – this includes the assay steps of
(a) Priming: pumping the washing buffer (Reagent A) to the microchamber and all microchannels;
(b) Sample loading: both sample and Reagent B (MB solution) solutions are pumped into the microchip to expel the washing buffer and fill the main chamber with the two solutions;
(c) Mixing: switching on and off of the magnetic pins in a predefined pattern to induce chaotic mixing of the two solutions (for HeV antibody to bind with the sG protein attached to MB);
(d) Washing: switching off of mixing and activating one or two pins to hold the MB in the chamber and then pumping the washing buffer to wash off all spent solutions;
(e) Label loading: with MB held in position, pumping Reagent C (fluorescent label solution) into the chamber;
(f) Mixing: repeating step 3 for labelled protein to bind to HeV antibody;
(g) Washing: pumping buffer solution again to the chamber to wash off all spent solutions.
4. Fluorescence detection and data collection.
5. Microchip removal and disposal.
All the assay steps together with the magnetic field and the fluid motion are software-controlled. For liquid handling images taken during the experiments, please refer to Fig. 1s in the ESI† document.
2.2 Materials
All reagents used in this study were of analytical grade. Carboxylate superparamagnetic beads (5% w/v, 5 m2 g−1 surface area, 99% magnetisation in 10 s, diameter of 0.5 μm) were purchased from Ademtech (Pessac, France). R-PE (the fluorescent protein) was obtained from Innova Biosciences (Cambridge, UK). Protein G, 1-ethyl-3-(3-dimethylaminopropyl)carbodiimide (EDC), was purchased from Pierce Biotechnology (IL, USA). The protein sG, HeV-specific monoclonal antibody (mAb) and raw horse serum samples were all provided by the CSIRO Australian Animal Health Laboratory (Geelong, Victoria). Generation of the viral envelope protein sG, used in this study for capturing HeV antibodies in animal sera, and the HeV-specific monoclonal antibody was described elsewhere.36,37 Virus neutralisation was conducted in a Biosafety Level 4 laboratory at the CSIRO Australian Animal Health Laboratory before delivery to our Microfluidics Laboratory (Clayton, Victoria). The sample used in this study was a raw horse serum sample collected from an immunogenicity study, for which the horses were vaccinated with HeV sG protein vaccine. All sera were gamma irradiated to deactivate any possible live virus prior to use outside the Biosafety Level 4 laboratories. The horse serum sample was diluted 1
:
1000, which was the sample concentration used in this study. The initial sample concentration was unknown.
2.3 Methods
2.3.1 Microchip fabrication.
As the microchips were fabricated using standard photolithography techniques, described in detail elsewhere,38,39 only a brief description is given here. The microchip design mask for the photolithography replica molding was created using L-Edit (Tanner EDA, ver.11) software. The chip master with a positive relief pattern was produced from a water-washable photopolymer sheet (NAPPDECO, S6-17, NAPP Inc., San Marco, CA, USA) by UV light exposure through the mask, followed by development in water. A mixture of a PDMS oligomer and a cross-linking agent (1
:
10, Sylgard 184, Midland, MI, USA) was poured onto the master and degassed for an hour. The PDMS chip was cured overnight at 60°. A Plasma Cleaner (Harrick Plasma, Ithaca, NY, USA) was used to clean the PDMS chip surface and a glass slide for 2 min (RF = 18 W, 500 mTorr) followed by irreversible bonding for the complete microfluidic chip used in this study.
2.3.2 Reagent conjugations.
Conjugation of sG to magnetic beads: magnetic beads (200 μL, 10 mg) were washed once in PBS-T (100 mM phosphate buffer, pH 7.4, 0.05% Triton X-100) and then in activation buffer (100 mM phosphate buffer, pH 6.2), and finally fully resuspended in 1 mL of the activation buffer. Freshly prepared EDC (125 μL, 50 mg mL−1) and NHS (125 μL, 50 mg mL−1) were added to the MNP and the reaction was performed on a rotary suspension mixer for 30 min to activate the nanoparticles. After washing twice in PBS-T, the MNP were resuspended in sG protein solution (1 mL, 0.5 mg mL−1) and the reaction was carried out for 2 hours. The MNP were then washed three times in PBS-T and resuspended in storage buffer (PBS, 1% BSA and 0.05% NaN3) at 10 μg μL−1 and stored at 4 °C. The conjugation was confirmed by determining the sG protein concentrations before and after the conjugation. The MNP conjugated to sG were designated as MNP-sG. Control MNP were treated with bovine serum albumin (BSA) in the same manner as the MNP-sG.
Conjugation of protein G to R-PE: the conjugation was performed using a Lightning-Link R-PE Conjugation Kit that allowed the conjugation of an antibody or protein to R-PE in a single step without the need for desalting or dialysis. Briefly, 1 mg of R-PE was resuspended in 40 μL of the modifier solution, to which 75 μg of Protein G in 400 μL of phosphate buffer (50 mM, pH 7.4) was added. The reaction was kept in the dark for 3 hours, after which 40 μL of the quencher solution was added and the reaction mixture was incubated for further 30 min in the dark. The conjugated protein was stored at 4 °C after adding NaN3 (0.05% w/v) and BSA (1 mg mL−1). The label was diluted 500-fold in PBS-T for antibody assays.
2.3.3 HeV antibody binding assay and on-chip detection.
Gao et al.8 developed a simplified, two-step binding assay for detection of HeV antibodies in animal sera. Briefly, the first step consisted of binding of antibodies from sera to the sG protein conjugated to the magnetic beads. The second step consisted of labeling of the magnetic bead–sG–antibody conjugate with R-PE. The PBS-T buffer was used to prime the microchip channel network. The same solution was used as a washing buffer between the subsequent assay steps. Firstly, the magnetic beads and the sample were introduced into the mixing chamber and mixed by applying a chaotic magnetic mixing protocol. Subsequently, the beads were retained against the mixing chamber wall by switching from the magnetic mixing software mode to magnetic retention mode. Secondly, the G/R-PE fluorescent label solution was introduced and the aforementioned steps were repeated. Finally, after washing off the G/R-PE label from the mixing chamber, the detection was performed.
The detection setup consisted of an inverted epifluorescence microscope connected to a photomultiplier tube (PMT, Hamamatsu). A 20× objective lens, a filter cube (Ex: 543 ± 22 nm; DM: 562 nm; Em: 593 ± 40 nm) and a ND8 filter were used in the experimental set-up. All detection measurements were the PMT readouts of the fluorescence intensities from the assay products. Each measurement was repeated three times and the results were presented as mean + standard error. Extensive washing of the microfluidic chip was performed between experimental runs to minimise the background noise.
2.3.4 Micro-PIV measurements.
To measure two-dimensional velocity fields in the mixing chamber, we used micro-particle image velocimetry. We selected 520 nm diameter polystyrene particles (Thermo Scientific) that satisfied the basic requirements for the particles' size to be large enough to dampen the effects of Brownian motion, and small enough to follow the flow without disrupting it.40 The particle density was 1.05 g cm−3, and particles were tagged with Firefli fluorescent red dye (excitation: 542 nm, emission: 612 nm). A green laser beam (DualPower Nd:YAG, 65 mJ per pulse, 532 nm, 15 Hz, Dantec Dynamics) was directed with a liquid light-guide to a 10× (NA = 0.25) objective resulting in a 1 mm × 1 mm field of view with a spatial resolution of 25 μm × 25 μm × 8.5 μm. A filter cube prevented the green light of the laser from being recorded. Pairs of images (Δt = 10 ms) were recorded and processed using the cross-correlation technique (Dynamic Studio, ver.4.1) with a 64 × 64 pixel interrogation window and 50% overlap. The average velocity was computed by averaging over a hundred images. The estimated velocity error due to Brownian motion was less than 1%.
3 Results and discussion
As fluids are removed from or introduced into the chamber during the various steps of the assay, the magnetic nanoparticles are held in place near the tip of one of the magnetic pins. Fluid mixing in the chamber is necessary to increase the efficiency of the assay. It is achieved by turning the magnets on and off in sequence. In Fig. 2, beads start at magnet A, then magnet A turns off and simultaneously magnet B switches on. The magnetic force pulls the beads along the edge of the chamber, generating a fixed quantum of circulation within the chamber. This sequence is repeated as magnet B goes off and C goes on, C goes to D and D goes to A to repeat the sequence. The four images (a)–(d) in Fig. 2 show the measured velocity fields corresponding to a particular magnetic pin switching sequence shown in sub-figure (f). The velocity vector arrows are plotted on top of the vorticity, which is given by the colored background, and dark blue indicates a region of dominant clockwise circulation. Aside from differences introduced by imperfections in the symmetry of the chamber, these circulations have the same geometry but are rotated, or reoriented, with respect to each other. Of course, there is nothing special about four magnets, we could use three or five or in principle any number of magnets. The objective of the analysis in this section is to calculate the best mixing sequence, incorporating the constraints of the device, that is, the simplest and cheapest to mass produce. This section will also show that the selected mixing chamber design and stirring protocol produce rapid antibody binding kinetics compared to a standard laboratory assay. And while other magnetization sequences could be used (e.g. turn on every other magnet to make beads cross the chamber), the simple, sequential protocol can be analyzed and optimized via an existing theory of chaotic mixing for periodically reoriented flows. Prior to that analysis, we first show that the circulations start and stop almost instantaneously by evaluating the force balance on the particles and the fluid. At the end of this section, we show data for the HeV antibody binding rates.
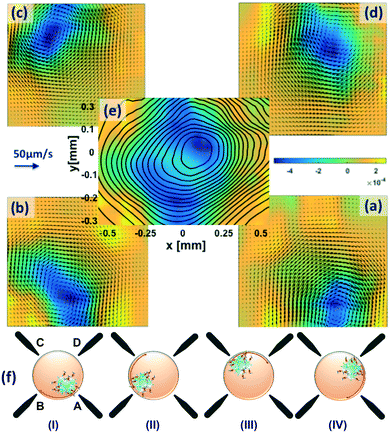 |
| Fig. 2 A particular sequence of flow patterns by turning on successive magnetic pins of the 4-pin microchip. Images (a)–(d) show the measured flow patterns which correspond to configurations (I)–(IV) in sub-figure (f), respectively. Image (e) shows the average of the vector fields in (a)–(d) with lines giving the stream function of the averaged flow. The colored background in images (a)–(e) is the vorticity field calculated from the measured velocity. | |
3.1 Force balance
While many forces operate on small particles moving through fluids,41 here we consider only the magnetic force and Stokes drag, assuming all other forces to be either negligible or ignorable for the sake of simplicity. With these assumptions, the equation of motion for a particle with size a and density ρp moving with velocity vp in a fluid of density ρf and viscosity η is |  | (1) |
where the first term on the right hand side is the magnetic force density42 and the second term is the drag force density. Non-dimensionalizing eqn (1) by velocity scale U and length scale R renders eqn (1) as | 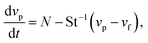 | (2) |
where the Stokes number | 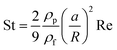 | (3) |
is the ratio of particle response time to a characteristic time of the flow, and the Stuart number | 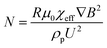 | (4) |
is the ratio of magnetic force to inertial force.
In initially quiescent fluid, vf = vp = 0. When the magnetic field is switched on, the second term of eqn (2) is zero and vp increases at a rate of N. With vf= 0, eqn (2) is readily integrated to give the particle velocity
With St ∼ 10
−7, a particle in our experiment almost instantaneously reaches its terminal velocity of
| 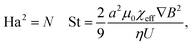 | (6) |
where Ha is a particle Hartmann number giving the ratio of magnetic force to viscous force on a particle. As
vp increases, drag force sets the fluid in motion until
vf ≈
vp when the second term of
eqn (2) becomes zero. The start-up of fluid motion also occurs on times of the order of St. Magnetic force continues to accelerate particles until
vp >
vf at which point fluid drag increases to slow the particle and maintain it at terminal velocity until particles encounter the hard surface of the chamber boundary at the magnetic pole face where particle motion stops. The Reynolds number of the flow Re is the ratio of inertial to viscous forces in the moving fluid and is defined as
|  | (7) |
where
ρ is the fluid density,
V is the mean bead cluster velocity,
L is the characteristic length, and
η is the fluid viscosity. For this study, Re = 0.02. As Re is small, when particle motion stops, fluid motion stops as well.
3.2 Diffusion or mixing?
In a steady laminar flow, the material must follow fluid streamlines, and there is no mixing except by diffusion. In a microfluidic setting with quite small length scales, a question may arise, why not just let diffusion accomplish mixing and drive reactions to completion? The answer is found by looking at the diffusion-limited reaction time scale |  | (8) |
where L is the characteristic length and D is the diffusion coefficient. This is the timescale that it takes for diffusion alone to mix constituents so that reactions can occur. As the diffusion coefficient of antibodies43 is about D = 3.9 × 10−11 m2 s−1 and the chamber diameter is 1 mm, the time needed for solutes to travel half the chamber diameter by diffusive motion alone is around 1.8 hours, which is an unacceptably long time. Another way to express this is with the non-dimensional Peclet number Pe which is the ratio of the rate of reactant transport accomplished by fluid motion to that of diffusion alone. Small Pe means that diffusion by itself would be effective for mixing reactants, while large Pe means that some stirring of fluid is required in order for reactions to occur swiftly. With Pe ∼ 103 in the current system, stirring is required to make a practical field device for Hendra virus detection.
3.3 Mixing theory for periodically reoriented flows
The flow in the mixing chamber generated by the motion of the magnetic beads is essentially a single flow that is periodically reoriented by the sequential activation of the magnetic pins. This allows us to bring a large body of theoretical results on periodically reoriented flows (PRFs) to bear to design the transport characteristics of the chamber. The theory of chaos and mixing in PRFs is well established44–47 but not well known, where mathematically PRFs are generalizations of linked twist maps.48 Here, we will consider the approximation that the experimental microfluidic flow is 2-dimensional. In its simplest version, a planar PRF consists of one flow with a stream function Ψ0 that operates over a dimensionless time interval τ, after which the flow stops and then resumes with Ψ0 rotated through an angle Θ. The validity of the approximation that the flow stops and starts instantaneously is valid when the fluid Reynolds and particle Stokes numbers are both small, which in the previous section was shown to be the case here.
The total stream function Ψ of a PRF is given by the sum of the base flow over all of its orientations.44–46 Switching on magnetic pins A, B, C, and D sequentially actuates magnetic forces acting on the magnetic bead clusters and induces fluid motion. For each of the four flow fields in Fig. 2, we summed the four velocity fields to obtain a single velocity field and then calculated the total stream function shown in image (e) of Fig. 2. The total stream function Ψ consists of a single vortex, which is not entirely symmetric because the four flows are not entirely symmetric (Fig. 2). A planar PRF can be analyzed as a perturbation of this set of nested, topological circles (via the mathematical machinery of the KAM and Poincáre–Birkhoff theorems) to design a stirring protocol and to select the number of magnetic pins to maximize the mixing and reaction rates for the assay.49–51
Fig. 3 shows the mixing rate for around 5000 simulations calculated using the fast method described by Lester et al.52 for Pe = 103. The colors denote the mixing rate of a scalar concentration field in a PRF that is topologically identical to the experimental flow in Fig. 2; the axes are reorientation angle Θ and time interval between reorientations τ. Note that the mixing rate does not vary smoothly—in fact, it's distribution in parameter space is fractal—and it would be difficult to guess a priori where mixer design optima lie. For the design of a PRF mixer, Fig. 3 shows a map whose maximum value, subject to the constraints of the mixer, gives the optimum design and operating point of the mixer to complete the assay in the shortest possible time.
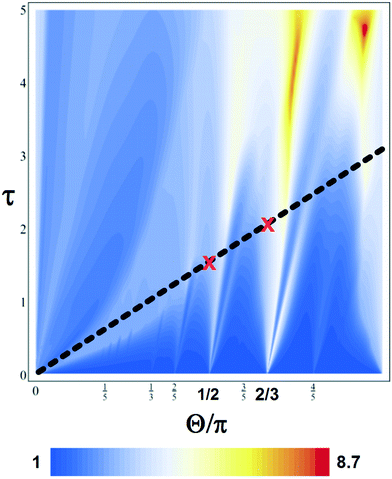 |
| Fig. 3 Predictions of enhanced scalar mixing rates at Pe = 103 with no-flux boundary conditions for a periodically reoriented flow topologically identical to that in Fig. 1. The axes are the reorientation angle Θ and the time interval between reorientations τ. The magnetic mixer is constrained to lie along the thick black line with the red crosses marking the 4-pin (2π/4 = π/2 tongue) and 3-pin (2π/3 tongue) design points. | |
As the motion of the magnetic beads drives the flow while they move from one magnetic pin to the next, in our experiment τ and Θ are not independent; in fact, they are constrained to τ = Θ. In addition, as the number of magnetic pins is constrained to be a whole number, the realizable reoriented magnetic bead mixer designs lie at the intersection of the dashed black line in Fig. 3 and rational values of Θ. In Fig. 3, 4-pin and 3-pin designs are marked by red crosses. In this case, as there is only a small difference predicted for mixing with the 3- or 4-pin configuration, we are free to choose without performance penalty the more convenient or less costly configuration.
Fig. 4 demonstrates the effectiveness of mixing of the current design with a time series of photographic images. Two fluids are introduced into the mixing chamber, one with white dye and the other clear, initially occupying the whole chamber without mixing. When the magnetic mixing is switched on, the two fluids start to mix and mix completely to the diffusion limit within a minute (a corresponding video is available in the ESI†).
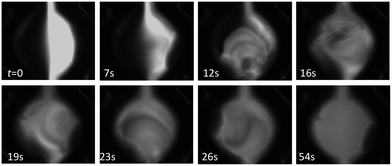 |
| Fig. 4 Visualisation of a magnetic mixing protocol using the 4-pin microchip. The photographs were taken at times of 0, 7, 12, 16, 19, 23, 26, and 54 s, respectively, from the start of mixing. For the chamber dimensions, please refer to Fig. 1. | |
3.4 Detection of HeV antibodies
With the mixing chamber and stirring protocol designed, we used the chip to detect HeV antibodies from diluted horse blood samples. The assay is first performed as described in the methods section. During the mixing stage, the bead cluster dilates as it moves between poles. As it dilates and moves, the porosity of the cluster changes and fluid moves through the porous bead cluster. This means that the entire surface area of all the beads in the cluster is available as a reaction surface to enhance the overall fluorescence signal.
The end point fluorescence measurements were carried out after a reaction time of 2 to 45 minutes. Each experiment started with the same number of beads in the reaction chamber, but the final porous cluster size and shape varied from experiment to experiment. Despite that, the measurement error was low ranging from 2.8% for 2 minutes to 0.5% for 45 minutes. A lower error value was expected for a longer mixing time due to the saturation of the fluorescence signal. In Fig. 5, the binding time course of the current assay is compared with the same assay performed in a test tube, using a permanent magnet for the retention and separation of the beads and a rotary mixer for the mixing.8 Assuming that the fluorescence intensity f plateaued after 45 minutes of mixing, the figure shows that after 20 minutes of mixing, the on-chip assays, carried out in microchips with 3 and 4 magnetic pins, reached the saturation point.
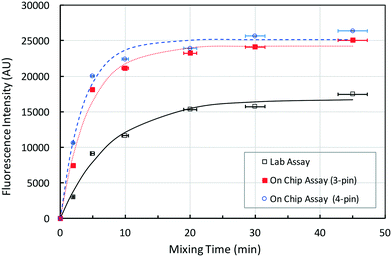 |
| Fig. 5 Comparison of the HeV antibody binding kinetics between on-chip assays and a laboratory assay. Symbols are the measured data and the curves are the corresponding least-square fittings using the exponential growth model f = f∞[1 − exp(−σt)]. The f∞, σ and R2 values are 16 740, 0.128 and 0.988 for the lab assay, 24 230, 0.228 and 0.988 for the 3-pin chip assay, and 25 140, 0.284 and 0.988 for the 4-pin chip assay, respectively. | |
An exponential growth model of the fluorescence increase, i.e., f = f∞[1 − exp(−σt)], is least-square fitted to three data sets. Here, f∞ is the asymptotic value of fluorescence intensity and σ is the rate of increase of fluorescence intensity in each assay. The model fits the experimental data well. The σ values are estimated to be 0.128, 0.228 and 0.284 for the lab and two on-chip assays, respectively. The 3-pin and 4-pin on-chip assays are roughly 1.8 and 2.2 times faster than the lab assay, which is in agreement with the prediction in Fig. 3. Fig. 3 predicts that the rates for either 3-pin or 4-pin magnetic mixing are approximately the same and the rate for the on-chip chaotic mixing assay is approximately 3 times faster than that for the diffusion-based assay. The current lab-based assay used a rotator mixer and, though not efficient, had a certain degree of mixing.
Additionally, Fig. 5 shows that the asymptotic fluorescence levels f∞ are 16
740, 24
230 and 25
140 for the lab and two on-chip assays, respectively. The on-chip assay not only is much faster but also generates a 40–50% larger absolute fluorescence intensity. The time needed for the assays to complete is ≈1 hour, assuming 20 min as a necessary mixing time. However, the on-chip assay with integrated chaotic mixing showed better performance for the lower mixing times. For example, after 5 minutes of mixing, the on-chip assay reached 75% of its maximum value, which is 30% higher than the value measured for the standard assay. If 5 minutes was adopted as a mixing time for chaotic magnetic micromixing on-chip, the time needed for the whole assay to complete can be reduced from 1 hour to 15 minutes. This reduction in time is significant when compared to 2–3 hours reported for detection of HeV antibodies in animal sera using Luminex or standard ELISA assays.53
Using this adopted mixing time, we further performed HeV antibody detection experiments by varying the antibody concentration. Fig. 6 shows a dose response curve using the current microchip device. Also shown in the figure are the data from the same assay but using the standard laboratory method.8 Both data are normalized by the maximum fluorescence intensity after subtracting the background fluorescence values. Both data agree with each other well and are well represented by the sigmoidal model, with an EC50 value of 12.5 ng ml−1. The limit of detection is estimated to be around 0.48 ng ml−1.
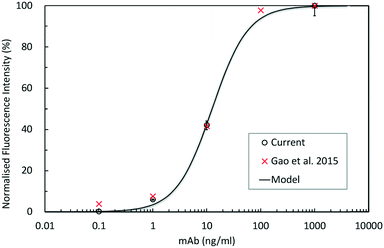 |
| Fig. 6 Dose response curve of HeV antibody detection (mean ± SD, n = 3). ×: lab plate assay data,8 ○: current on-chip data. The curve is the least-square fit of sigmoidal function. | |
4 Conclusions
In this study, a lab-on-a-chip device is developed to address the current technology demand for rapid field diagnosis of Hendra virus infection. This device is capable of automatic liquid handling with detection based on a magnetic nanoparticle immunoassay technique. These particles are also used to achieve rapid chaotic mixing in the assay chamber. A chaotic theory was applied to develop the mixing protocol. With the developed mixing technique, the detection of HeV antibodies can be achieved in approximately 15 minutes, an order of magnitude decrease from the time necessary to perform a standard laboratory-based assay. Such an improvement shows great potential for detection of HeV and other biological and chemical substances at the point of sampling. The limit of detection is estimated to be around 0.48 ng ml−1. The future plan for the device development is to further simplify the reagent loading methods (e.g. pre-loading reagents in the microchip), reduce the cost and size by using cheaper and more portable detectors, and simplify the magnetic pin fabrication method.
Acknowledgements
The authors would like to thank Dr. Jackie Pallister of CSIRO Biosecurity Flagship for providing the infected horse blood samples. This work was performed in part at the Melbourne Centre for Nanofabrication (MCN) in the Victorian Node of the Australian National Fabrication Facility. Y. Zhu also acknowledges the support of MCN through the Technology Fellowship Program.
References
-
L.-F. Wang and P. Daniels, Curr. Topics Microbiol. Immuno, Springer-Verlag, 2012, vol. 359, pp. 179–196 Search PubMed.
- S. Feldman, A. Foord, H. Heine, I. Smith, V. Boyd, G. A. Marsh, J. Wood, A. Cunningham and L.-F. Wang, J. Virol. Methods, 2009, 161, 52–57 CrossRef PubMed.
- P. Murray, R. Rogers, L. Selvey, P. Selleck, A. Hyatt, A. Gould, L. Gleeson, P. Hooper and H. Westbury, Emerging Infect. Dis., 1995, 1, 31–33 CrossRef PubMed.
-
H. Field, G. Crameri, N. Y.-H. Kung and L.-F. Wang, Curr. Topics Microbiol. Immuno, Springer-Verlag, 2012, vol. 359, pp. 11–23 Search PubMed.
- B. T. Eaton, C. C. Broder, D. Middleton and L.-F. Wang, Nat. Rev. Microbiol., 2006, 4, 23–35 CrossRef CAS PubMed.
-
C. D. Stewart, Weapons of Mass Casualties and Terrorism Response Handbook, American Academy of Orthopaedic Surgeons (AAOS), 2006 Search PubMed.
- G. Crameri, L.-F. Wang, C. Morrissy, J. White and B. T. Eaton, J. Virol. Methods, 2002, 99, 41–51 CrossRef CAS PubMed.
- Y. Gao, J. Pallister, F. Lapierre, G. Crameri, L.-F. Wang and Y. Zhu, J. Virol. Methods, 2015, 222, 170–177 CrossRef CAS PubMed.
- K. N. Bossart, J. A. McEachern, A. C. Hickey, V. Choudhry, D. S. Dimitrov, T. E. A. Bryan and L. F. Wang, J. Virol. Methods, 2007, 142, 29–40 CrossRef CAS PubMed.
- C. D. Chin, T. Laksanasopin, Y. K. Cheung, D. Steinmiller, V. Linder, H. Parsa, J. Wang, H. Moore, R. Rouse, G. Umviligihozo, E. Karita, L. Mwambarangwe, S. L. Braunstein, J. van de Wijgert, R. Sahabo, J. E. Justman, W. El-Sadr and S. K. Sia, Nat. Med., 2011, 17, 1015–1020 CrossRef CAS PubMed.
- A. M. Foudeh, T. F. Didar, T. Veresa and M. Tabrizian, Lab Chip, 2012, 12, 3249–3266 RSC.
- G. Wang, G. Shi, H. Wang, Q. Zhang and Y. Li, Adv. Funct. Mater., 2014, 24, 1017–1026 CrossRef CAS.
- Y. Li, J. Xuan, Y. Song, W. Qi, B. He, P. Wang and L. Qin, ACS Nano, 2016, 10, 1640–1647 CrossRef CAS PubMed.
- J. M. Nam, C. S. Thaxton and C. A. Mirkin, Science, 2003, 301, 1884–1886 CrossRef CAS PubMed.
- M. A. M. Gijs, Microfluid. Nanofluid., 2004, 1, 22–40 CAS.
- N. Pamme and A. Manz, Anal. Chem., 2004, 76, 7250–7256 CrossRef CAS PubMed.
- K. S. Kim and J.-K. Parkj, Lab Chip, 2005, 5, 657–664 RSC.
- D. W. Inglis, R. Riehn, J. C. Sturm and R. H. Austin, J. Appl. Phys., 2006, 99, 08K101 CrossRef CAS.
- R. Khnouf, G. Goet, T. Baier and S. Hardt, Analyst, 2014, 139, 4564–4571 RSC.
- C.-H. Wang, K.-Y. Lien, J.-J. Wu and G.-B. Lee, Lab Chip, 2011, 11, 1521–1531 RSC.
- J. Lum, R. Wang, K. Lassiter, B. Srinivasan, D. Abi-Ghanem, L. Berghman, B. Hargis, S. Tung, H. Lu and Y. Li, Biosens. Bioelectron., 2012, 38, 67–73 CrossRef CAS PubMed.
- H. C. Tekin and M. A. M. Gjis, Lab Chip, 2013, 13, 4711–4739 RSC.
- L.-Y. Hung, J.-C. Chang, Y.-C. Tsai, C.-C. Hunag, C.-C. Chang, C.-S. Yeh and G.-B. Lee, Nanomedicine, 2014, 10, 819–829 CAS.
- A. van Reenen, A. M. de Jong, J. M. J. den Toonder and M. W. J. Prins, Lab Chip, 2014, 14, 1966–1986 RSC.
- Q. Cao, X. Han and L. Li, Lab Chip, 2015, 15, 2762–2777 Search PubMed.
- F. Lisi, P. Falcaro, D. Buso, A. J. Hill, J. A. Barr, G. Crameri, T.-L. Nguyenm, L.-F. Wang and P. Mulvaney, Adv. Healthcare Mater., 2012, 1, 631–634 CrossRef CAS PubMed.
- A. Rida and M. A. M. Gijs, Anal. Chem., 2004, 76, 6239–6246 CrossRef CAS PubMed.
- Y. Wang, J. Zhe, B. T. F. Chung and P. Dutta, Microfluid. Nanofluid., 2008, 4, 375–389 CrossRef.
- T. Lund-Olesen, B. B. Buus, J. G. Howalt and M. F. Hansen, J. Appl. Phys., 2008, 103, 07E902 CrossRef , 1–3.
- H. Suzuki, C. M. Ho and N. Kasagi, J. Microelectromech. Syst., 2004, 13, 779–790 CrossRef.
- M. Zolgharni, S. M. Azimi, M. R. Bahmanyar and W. Balachandran, Microfluid. Nanofluid., 2007, 3, 677–687 CrossRef.
-
R. Rong, J. Choi and C. Ahn, Proc. 7th MicroTAS, 2003, pp. 335–338 Search PubMed.
-
R. J. Wang and J. Z. Lin, 1st IEEE Int. Conf. Nano/Micro Engineered and Molecular Systems, 2006, pp. 74–77 Search PubMed.
- N. Le, M. Gel, Y. Zhu, H. Dacres, A. Anderson and S. C. Trowell, Biosens. Bioelectron., 2014, 62, 177–181 CrossRef CAS PubMed.
- N. Le, M. Gel, Y. Zhu, J. Wang, H. Dacres, A. Anderson and S. C. Trowell, Biomicrofluidics, 2014, 8, 064110 CrossRef PubMed.
- J. Pallister, D. Middleton, L. F. Wang, R. Klein, J. Haining, R. Robinson, M. Yamada, J. White, J. Payne, Y. R. Feng, Y. P. Chan and C. C. Broder, Vaccine, 2011, 29, 5623–5630 CrossRef CAS PubMed.
- Z. Zhu, K. N. Bossart, K. A. Bishop, G. Crameri, A. S. Dimitrov, J. A. McEachern, Y. Feng, D. Middleton, L. F. Wang, C. C. Broder and D. S. Dimitrov, J. Infect. Dis., 2008, 197, 846–853 CrossRef CAS PubMed.
- N. Wu, Y. Zhu, S. Brown, J. Oakeshott, T. S. Peat, R. Surjadi, C. Easton, P. W. Leech and B. A. Sexton, Lab Chip, 2009, 9, 3391–3398 RSC.
- Y. Zhu and K. Petkovic-Duran, Microfluid. Nanofluid., 2011, 8, 275–282 CrossRef.
- J. G. Santiago, S. T. Wereley, C.
D. Meinhart, D. J. Beebe and R. J. Adrian, Exp. Fluids, 1998, 25, 316–319 CrossRef CAS.
-
G. Metcalfe, M. Speetjens, D. Lester and H. Clercx, in Advances in Applied Mechanics, ed. E. van der Giessen and H. Aref, Academic Press, 2012, vol. 45, pp. 109–188 Search PubMed.
- E. P. Furlani, J. Appl. Phys., 2006, 99, 024912 CrossRef.
- D. M. Yarmush, R. M. Murphy, C. K. Colton, M. Fisch and M. L. Yarmush, Mol. Immunol., 1988, 25, 17–32 CrossRef CAS PubMed.
- G. Metcalfe, M. Rudman, A. Brydon, L. Graham and R. Hamilton, AIChE J., 2006, 52, 9–28 CrossRef CAS.
- M. Speetjens, G. Metcalfe and M. Rudman, Phys. Fluids, 2006, 18, 1–11 Search PubMed.
-
G. Metcalfe, in Complex Physical, Biophysical, and Econophysical Systems, ed. R. L. Dewar and F. Detering, World Scientific, 2010, vol. 9, pp. 189–242 Search PubMed.
- Ö. Baskan, M. Speetjens, G. Metcalfe and H. Clercx, Chaos, 2015, 25, 1–9 CrossRef PubMed.
- J. M. Ottino and S. Wiggins, Science, 2004, 305, 485–486 CrossRef CAS PubMed.
- D. Lester, M. Rudman, G. Metcalfe and H. Blackburn, J. Comput. Phys., 2008, 227, 3032–3057 CrossRef.
- D. Lester, M. Rudman and G. Metcalfe, Int. J. Heat Mass Transfer, 2009, 52, 655–664 CrossRef CAS.
- Ö. Baskan, M. Speetjens, G. Metcalfe and H. Clercx, Eur. J. Mech. B Fluids, 2016, 57, 1–14 CrossRef.
- D. Lester, G. Metcalfe and M. Rudman, Phys. Rev. E: Stat., Nonlinear, Soft Matter Phys., 2014, 90, 022908 CrossRef PubMed.
- L. McNabb, J. Barr, G. Crameri, S. Juzva, S. Riddell, A. Colling, V. Boyd, C. Broder, L.-F. Wang and R. Lunt, J. Virol. Methods, 2014, 200, 22–28 CrossRef CAS PubMed.
Footnote |
† Electronic supplementary information (ESI) available. See DOI: 10.1039/c6lc01263a |
|
This journal is © The Royal Society of Chemistry 2017 |