Improving selective targeting to macrophage subpopulations through modifying liposomes with arginine based materials†
Received
14th July 2016
, Accepted 4th November 2016
First published on 7th November 2016
Abstract
The effects of surface modifications on liposomes using a library of arginine derivatives for improved drug delivery were examined. Both unmodified and modified liposomes were tested for their drug delivery properties and propensity for internalization by macrophages. All materials were characterized by dynamic light scattering (DLS) and zeta potential. The resulting liposomes were able to encapsulate doxorubicin with a loading efficiency greater than 90% and cumulative releases of less than 15% after 144 h. The internalization of these particles was examined by loading the liposomes with fluorescein or doxorubicin to test internalization through fluorescence level and half maximal inhibitory concentration (IC50), respectively. RAW 264.7 macrophages were activated with lipopolysaccharide (LPS) or interleukin-4 (IL-4) to induce M1- or M2-like phenotypes. Naïve macrophages were also studied. Most modified liposomes enhanced the cytotoxicity of doxorubicin compared to unmodified liposomes. Macrophage phenotype was also observed to influence the cytotoxicity of doxorubicin entrapped in modified liposomes, with some samples enhancing the cytotoxicity in LPS stimulated macrophages and some enhancing toxicity in IL-4 stimulated cells.
Insight, innovation, integration
Macrophages exist on a spectrum from pro-inflammatory to pro-angiogenic phenotypes. Tumor associated macrophages are generally functionally similar to pro-angiogenic macrophages, resulting in poorer prognosis. Depleting these pro-angiogenic macrophages from tumors may result in inhibition of tumor growth, making these cells attractive drug targets. This study examines the ability to target specific subpopulations of macrophages through surface modifications of liposomes. We examined how the physiochemical properties of the modifiers alter the toxicity of the liposomes. Our study reveals that these different subpopulations of macrophages can be targeted through different surface modifications and that physicochemical properties, namely the number of hydrogen bond donors, freely rotating bonds, zeta potential, and lipophilicity, of the modifiers correlated with this improved selectivity.
|
1. Introduction
Liposomes have been extensively used as biocompatible and biodegradable nanocarriers for a variety of applications extending from basic research to clinical uses. Currently, there are 17 different liposomal formulations that have been approved by US Food and Drug Administration (FDA) for clinical treatments and a large number of formulations are in clinical trials.1 Doxil®, the first FDA approved liposomal doxorubicin formulation, was initially approved in 1995 for use in treatment of Kaposi's sarcoma.2 DaunoXome®, a liposomal formulation of daunorubicin, is used as treatment of leukemia and blood tumors. Clinical reports have demonstrated that DaunoXome® results in less alopecia and neuropathy, and tumor uptake is 10-fold higher compared to free drug.3 Liposomes are unilamellar lipid bilayer nanoparticles and are larger than micelles, providing less drug leakage but enhanced encapsulation capability.4 Liposomes are commonly delivered to tumor sites through passive targeting, exploiting the enhanced permeation and retention (EPR) effects of the tumor.4
Macrophages can change their phenotype based on the stimulus that activates them. Pro-inflammatory or classical activated M1 macrophages5 can be activated with interferon (IFN)-γ or LPS, while anti-inflammatory or alternatively activated M2 macrophages can be activated by IL-4.6 To more accurately describe the phenotype of the macrophages in vitro, the new nomenclature proposed by Murray et al.7 will be used throughout the paper. This nomenclature is based on the molecule used to activate the cells, for example M(LPS) and M(IL-4). Naïve cells will be denoted as M(0). Tumor associated macrophages (TAMs) generally exhibit characteristics of M2 macrophages. Thus, TAMs are associated with poor prognosis in cancer patients due to their propensity to release pro-angiogenic factors.8,9 Depleting TAMs in the tumor microenvironment can result in a significant reduction in tumor growth,10 thus they are an attractive target for developing cancer therapeutics.
Targeting drugs to macrophages has been reported through various methods, many of which utilize liposomes.11,12 Many of these methods use biological ligands to target receptors. One target is CD163, which is a hemoglobin scavenger receptor expressed on M2 macrophages. By incorporating CD163 antibody on the liposome surface, improved internalization in M2 macrophages was observed.13 Histidine-rich glycoprotein has been shown to polarize macrophages towards an M1 phenotype and has potential for targeted delivery.14 It has also been previously demonstrated that materials properties can shift macrophage phenotype,15–18 thus the aim of the work is to examine how physicochemical properties impact delivery to macrophages and to improve selective delivery to M2 macrophages.
Arginine plays an important role in immune responses, and is metabolized by two enzymes differently up-regulated in polarized macrophages: nitric oxide synthase (NOS) and arginase.19,20 The former produces citrulline and reactive nitrogen intermediates in M1 macrophages, and the latter produces ornithine and urea in M2 macrophages.19 Some arginine derivatives have been investigated and have shown therapeutic effects. Nitroarginine has neuroprotective effects in Alzheimer's disease, Parkinson's disease, and AIDS.21 Acetylcarnitine is involved in membrane stabilization and repair, which reduces protein oxidation and toxic fatty acid ethyl esters.22 The immune system is regulated by L-arginine metabolism20 and can be modulated by arginine and arginine-rich peptides.23 All of these factors indicate that arginine derivatives have the potential to interact with macrophage surface receptors of different phenotypes.
In this study, we fabricated liposomes and modified their surfaces with 14 molecules that are chemically similar to arginine in order to investigate the effects of surface modifications on internalization by all phenotypes of macrophages. Also, we characterized the size and surface charge of both modified and unmodified liposomes. Finally, the liposomes were loaded with doxorubicin, an anti-cancer drug, and were examined for their loading efficiency and release kinetics at pH 7.4. Changes in the IC50 of doxorubicin entrapped in the unmodified and modified liposomes was compared to that of free doxorubicin in M(LPS), M(IL-4), and M(0) macrophages.
2. Material and methods
All materials were purchased through Sigma and were used as received, unless otherwise stated. Fresh deionized (DI) water (Milli-Q, Thermo Scientific Nanopure, Waltham, MA) was used throughout this study. Error bars indicate the standard deviation.
2.1. Liposome modification
In a 250 ml round-bottom flask, 1,2-dioleoyl-sn-glycero-3-phosphoethanolamine (DOPE, Avanti Polar Lipids, Inc., Alabaster, AL) (87.5 mg) and 1,2-dioleoyl-sn-glycero-3-phosphocholine (DOPC, Avanti Polar Lipids, Inc.) (43.75 mg) were dissolved in chloroform (15.75 ml) and rotary evaporated at 40 °C for 5 min. Then, the lipids were mixed with 15 ml phosphate buffered saline (PBS, diluted from 10× solution to 0.1 M, pH 7.4, Fisher Scientific, Pittsburgh, PA). After dialyzing against DI water overnight, the liposome particles were freeze-dried by a lyophilizer (Labconco, 4.5L, Kansas City, MO). Fourteen different molecules (Fig. 1) were used to modify the liposomes: 2-amino-3-guanidinopropionic acid, 3-guanidinopropionic acid, nitroarginine, creatine (Fisher, Pittsburgh, PA), carnitine, citrulline, 5-hydroxylysine, acetylglutamine, N-carbamyl-α-aminoisobutyric acid, acetylcarnitine, 2,4-diaminobutyric acid, acetylornithine, albizziin, and arginine (Amresco, Solon, OH). In a vial, 2 ml PBS, 10 mg of lyophilized liposomes resuspended in PBS at 5 w/v%, 2 mg surface modifier, and 20 mg N-(3-dimethylaminopropyl)-N′-ethylcarbodiimide hydrochloride (EDC) were stirred overnight. This process was repeated for all 14 surface modifiers. The particles were dialyzed overnight against DI water and lyophilized.
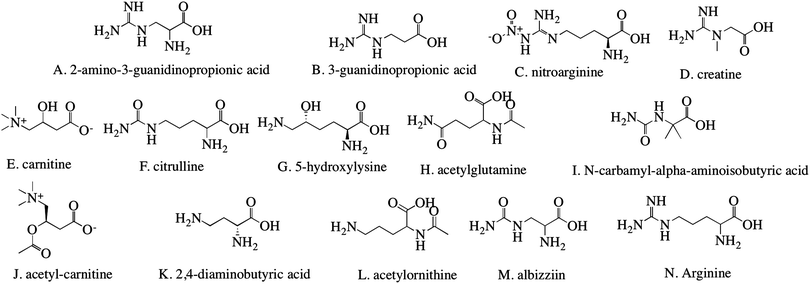 |
| Fig. 1 Chemical structures of molecules used for the modification of liposomal particles. The arginine derivatives shown here are lettered for easier identification in experiments and discussion throughout the paper. | |
2.2. Zeta potential and dynamic light scattering
DI water was adjusted to pH 7 with HCl or NaOH to ensure the ions in the water would not influence the results by interfering with the liposomes. To 5 ml H2O, 100 μl 1% w/v of particles in DI water were added and extruded through a 100 nm polycarbonate membranes using an Avanti Mini-Extruder (Avanti Polar Lipids, Inc.). Zeta potential and particle size was measured with a Zetasizer Nano Z (Malvern).
2.3. Drug loading and release
Doxorubicin was used as a model drug for liposome loading and release. In 2 ml citric acid (150 nM, pH 4), 10 mg modified or unmodified liposome (UM) from Section 2.1 were suspended and extruded 21 times using an Avanti Mini-Extruder. The liposomes were neutralized to pH 7.4 with NaOH or HCl. Both the doxorubicin solution (PBS, 10 mg ml−1) and the extruded liposomes were incubated at 65 °C for 10 minutes. Subsequently, 200 μl doxorubicin were added to the suspended liposomes and incubated at 65 °C for an additional 45 minutes. The loaded liposomes were centrifuged at 3000 rpm for 5 minutes and the supernatant was removed. To a 96 well plate, 50 μl of supernatant and 50 μl of PBS were added into each well. A standard curve was made through a serial dilution of 1 mg ml−1 doxorubicin. The amount of doxorubicin not entrapped was analyzed by measuring the absorbance at 490 nm with a reference at 630 nm using a plate reader. Loading efficiency of the liposomes was calculated by |  | (1) |
where Csup is the concentration of doxorubicin in the supernatant and Ctotal is the concentration of doxorubicin added to the liposomes.
Liposomes loaded with doxorubicin were placed in a dialysis membrane (MWCO 3500), and set in a beaker with 100 ml of PBS. The beaker was sealed and incubated at 37 °C. Aliquots of 1 ml were collected at designated time points and assayed spectrophotometrically using a BioTek Synergy HT Multidetection Microplate Reader (BioTek, Winooski, VT) at an absorbance of 490 nm with a reference at 630 nm. After each sample was removed, 1 ml PBS was added to maintain a constant volume.
2.4. Cell culture
RAW 264.7 macrophages (ATCC, Manassas, VA) were cultured at 37 °C with 5% CO2 in complete medium (CM), consisting of 10% fetal bovine serum, 100 U L−1 penicillin, and 100 μg L−1 streptomycin in Dulbecco's modified Eagle's medium (DMEM). Cells were passaged every three to five days and subcultured between 6.7 × 103 and 2.7 × 104 cells per cm2.
2.5. Fluorescent particles and cellular uptake
To measure internalization of the liposomes by macrophages, 2 mg of liposomes were mixed with 1 ml fluorescein (FC, 1 mg ml−1 in acetone). The liposomes were subsequently dried at 55 °C for 4 h and particles were resuspended in 2 ml PBS. The liposomal suspension was passed through a Sephadex G-50 column (Fisher) to remove the unencapsulated FC. RAW 264.7 cells (1.6 × 105 cells per cm2 with 100 μl CM in each well, except negative control) were seeded in a black 96 well plate. After incubating for 24 h at 37 °C, the media was carefully aspirated and then 200 μl liposomes resuspended in CM were added to the wells. These cells were either incubated at 37 °C to measure internalization of the liposomes, or at 4 °C for cold binding experiments.
To measure internalization of the particles, the cells were incubated at 37 °C for 4 h before the media was aspirated, and 100 μl 0.25% trypan blue (Corning, Manassas,VA) were added to quench the extracellular fluorescence. The trypan blue was aspirated after 1 min and the fluorescence was measured at an excitation of 360 nm and an emission of 460 nm with a plate reader. Cold binding experiments were performed in a similar manner incubating at 4 °C for 4 h. Controls for both internalization and cold binding experiments consisted of FC loaded liposomes without cells and cells without liposomes. Ten replicates were obtained for all liposomes samples. The fluorescence was normalized to the amount of FC added to each well.
2.6. IC50
RAW 264.7 cells (1.6 × 105 cells per cm2 in 100 μl CM in every well except the negative control) were seeded into a 96 well plate for 24 h. A serial dilution of liposomes loaded with doxorubicin was added to the plate. A positive control was conducted without liposomes. After incubating for 48 h at 37 °C, the media was aspirated and 10 μl of 5 mg ml−1 methyl thiazol tetrazolium (MTT) and 100 μl CM were added to each well. The plate was incubated at 37 °C for 2 h. A volume of 85 μl was aspirated from each well and 100 μl dimethyl sulfoxide (Fisher) were added to dissolve the insoluble formazan crystals. The optical density at 540 nm and a reference of 690 nm were measured. Data was normalized to cells cultured without particles and 6 replicates were obtained for each experiment.
2.7. Statistics and data analysis
Statistical analysis was performed using JMP statistical software. Statistical significance of the mean comparisons was determined by a two-way ANOVA. Pair-wise comparisons were analyzed with Tukey's honest significance difference test. Differences were considered statistically significant for p < 0.05. Principal component analysis (PCA) was used to provide a description of the covariance structure. The principle components are linear combinations of the original variables, plotted on axes to represent directions of maximum variance. The relationship between materials can be observed through projections of the first and second principal components (PC1 and PC2) in two-dimensional space. In this work, PCA was used to explain relationships between modifier properties and internalization by RAW 264.7 macrophages, along with IC50 values obtained for M(LPS), M(IL-4), and M(0) stimulated cells.
3. Results
3.1. Liposome characterization
Subtle differences between the different modifiers were reflected in zeta potential values derived from the electrophoretic mobility measurements under identical experimental conditions. Zeta potential represents the relative surface charge of particles, which contributes to particle internalization.24Fig. 2A shows the zeta potential of both modified and unmodified liposomes. Unmodified liposomes have a zeta potential of −16.8 ± 0.8 mV, which is similar to previous reports25,26 and reflects the phosphate group in the lipids. All modified liposomes were negatively charged over a wide range (from −8.9 to −33.9 mV), which may arise from the different functional groups of the modifiers. Details of multiple comparisons for this data are in Table S1 (ESI†).
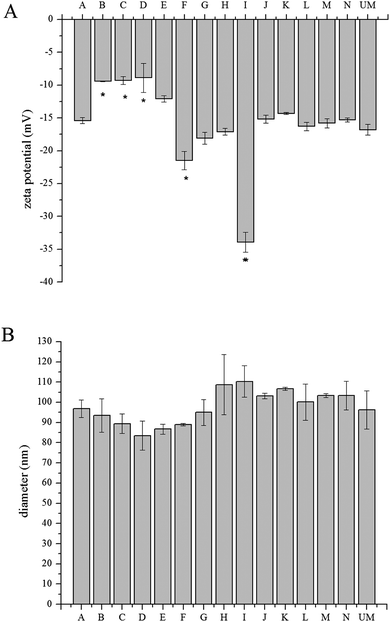 |
| Fig. 2 Material characterization of modified liposome particles. (A) Zeta potential and (B) DLS measurements of the liposomes. Both measurements represent three replicates for each sample, all data are shown by mean value ± standard deviation. (*) indicates p < 0.05 compared with unmodified liposomes by pairwise comparison. UM = unmodified liposomes. | |
Nanoparticle size is a determining factor in drug delivery.24 Through dynamic light scattering, the diameter of the nanoparticles was measured (Fig. 2B). The unmodified liposomes were found to be 96.3 ± 9.4 nm and the size of modified liposomes was measured to be ∼100 nm, with the largest liposome being 110.3 ± 7.8 nm. The size of the unmodified liposome matched previous reports.26,27 The polydispersity index (PDI) ranged from 0.096 to 0.167 for all of the liposomes. Details of multiple comparisons for this data are in Table S2 (ESI†).
3.2. Drug loading and release
A transmembrane pH gradient was employed as an active loading method to encapsulate doxorubicin in liposomes.28 Through drug self-association and interaction with salts, doxorubicin precipitates in the aqueous core of the liposome.29 This is a direct method to encapsulate doxorubicin to achieve optimum efficiency and reduce costs. The loading efficiency data (Fig. 3) shows that all materials have a loading efficiency of greater than 90%. Liposomes loaded with doxorubicin were incubated in PBS at 37 °C for 144 h to monitor drug release using the dialysis bag method. All of the liposomes released less than 15% of encapsulated doxorubicin after 144 h, as shown in Fig. 4, which indicates sustained release capability. Modification F has a lower release percentage compared to the unmodified liposome (p < 0.05). According to the statistical analysis, there are no differences among the unmodified liposomes and other modified samples (p > 0.05), indicating uniform drug retention. Details of multiple comparisons for the cumulative release data are in Table S3 (ESI†).
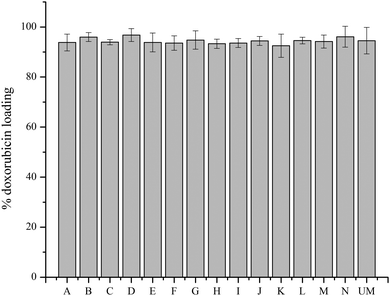 |
| Fig. 3 Doxorubicin loading efficiency for unmodified and modified liposomes. Measurements represent three replicates for each sample; all data are shown by mean value ± standard deviation. | |
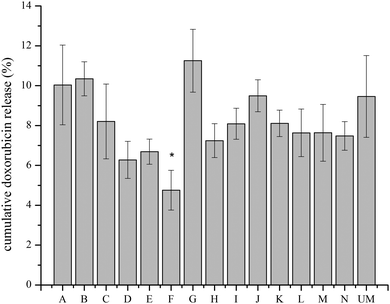 |
| Fig. 4 The majority of doxorubicin remains entrapped in the modified liposomes after 144 h. Cumulative doxorubicin release from modified and unmodified liposomes at 144 h incubation in PBS. (*) indicates p < 0.05 compared with unmodified liposomes by pairwise comparison. | |
3.3. Particle internalization
The unmodified and modified liposomes were loaded with FC and were incubated with RAW 264.7 macrophages to measure particle internalization. A constant particle concentration (0.1 mg ml−1) was used throughout the study. Fig. 5 shows the fluorescence level of liposomes internalized by the macrophages. At 4 °C, all pathways for cell internalization are blocked.30 The lack of fluorescence indicates that there is very little particle internalization or adsorption on the cell surface at 4 °C. At 37 °C there is a substantial increase in the fluorescence level, which demonstrates that the liposomes are internalized by the macrophages (Fig. 5A). For M(LPS), the fluorescence values for particles H, I, M, and N are statically higher than unmodified liposomes (p < 0.05) with the remaining modifications being similar to unmodified liposomes (Fig. 5). For M(IL-4), modification A, B, C, D, E, and L are statistically similar to unmodified liposomes (p > 0.05) and the other particles have 2- to 4-fold higher fluorescence levels than unmodified liposomes (p < 0.05) (Fig. 5). The highest fluorescence value for liposomes incubated with M(0) cells is for modification N, which is around 4 fold greater than the lowest modification, M. Particles A, C, D, G, H, K, and M are statistically similar to unmodified liposomes (p > 0.05) with all other modifications more favorably internalized compared to unmodified liposomes (p < 0.05) (Fig. 5). Unlike M(0)cells, incubation of the liposomes with M(LPS) or M(IL-4) cells at 4 °C revealed some fluorescence, suggesting that the particles may be adsorbing to the cell surface (Fig. 5B). Details of multiple comparisons for this data are in Tables S4–S9 (ESI†).
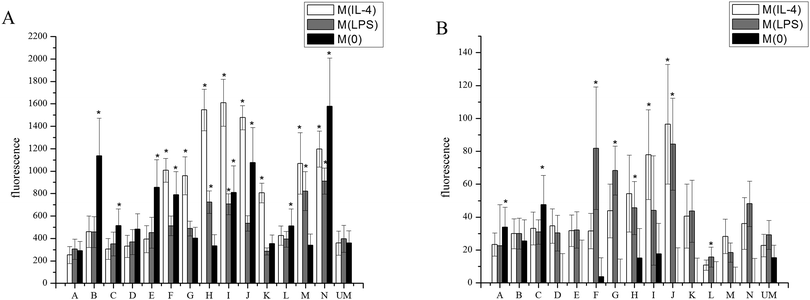 |
| Fig. 5 Fluorescence level of FC loaded particles incubated with macrophages. M(LPS), M(IL-4), and M(0) cells were incubated with FC loaded particles at (A) 37 °C and (B) 4 °C. Data presents the mean value of ten replicates for each sample ± standard deviation. The fluorescence was normalized to the amount of FC added to each well. (*) indicates p < 0.05 compared with unmodified liposomes with the same incubation temperature and stimulation by pairwise comparison. | |
3.4. IC50
IC50 is the half of maximum of inhibitor concentration, which is a measurement of the cytotoxic effects of liposomal delivery of doxorubicin. Macrophages stimulated with LPS or IL-4, along with naïve macrophages were incubated with the doxorubicin loaded liposomes for 48 h and their responses was calculated as a percentage of the cells not treated with the liposomes.31 Sigmoidal dose–response curves were used to calculate IC50 values for each macrophage condition and liposome modification, shown in eqn (2): | 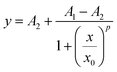 | (2) |
where A1 is the upper limit of the dose curve, A2 is the lower limit, x0 is the IC50, and p is the steepness of the curve. M(LPS), M(IL-4), and M(0) macrophages were tested. Fig. 6 shows that surface modifications alter the IC50 compared to unmodified liposomes for many of the macrophage phenotypes. Unmodified liposomes have similar IC50 levels for M(IL-4) and M(0) and lower IC50 values for M(LPS). Lower IC50 values indicate higher cytotoxicity. For LPS activated macrophages, most of the liposomal doxorubicin formulations have more acute activity compared to the unmodified liposome (IC50 < 10 μg ml−1). For IL-4 activated macrophages, unmodified liposome and modifications A, B, and C show weaker toxicities than free doxorubicin, with IC50 values of 24.6, 25.5, 27.3, and 32.6 μg ml−1, respectively, while modifications E through N show comparably more toxic effects (p < 0.05). Naïve macrophages exhibited higher IC50 in the presence of unmodified liposomes and modifications A and N compared to other modified liposomes (p < 0.05). All tested particles display a high toxicity, with the lowest being modification C in the presence of IL-4 activated macrophages (IC50 = 32.6 μg ml−1). Most modifications were lower for LPS-activated macrophages than IL-4 activated macrophages, with the exception of modifications H and I and negligible differences between the two phenotypes for modification E. The lower IC50 values for M(IL-4) cells compared to M(LPS) cells suggests that modifications H and I could be used to improve targeted delivery to TAMs. From the activated and non-activated data it could be concluded that doxorubicin loaded in modified liposomes alters the IC50 depending on the stimulation of macrophages and can be exploited to improve drug delivery. Details of multiple comparisons for this data are in Tables S10–S12 (ESI†).
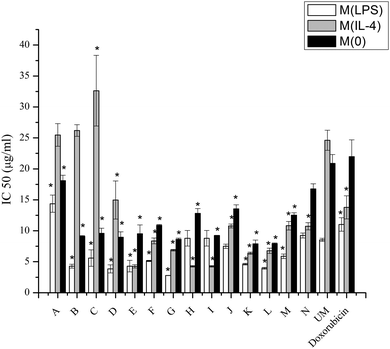 |
| Fig. 6 IC50 concentrations for LPS or IL-4 or non-activated macrophages. IC50's for modified liposomes, unmodified liposome loaded with doxorubicin and free doxorubicin by LPS or IL-4 activated or naïve macrophages. Data represents the mean value of three replicates for each sample ± standard deviation. (*) indicates p < 0.05 compared with unmodified liposomes with the same activation by pairwise comparison. | |
3.5. Correlations between IC50 values for M(LPS), M(IL-4), and M(0) macrophages and materials properties
The relationships between properties of the modifiers and the IC50 values for doxorubicin loaded liposomes on the three different macrophage states were then investigated through informatics analysis (Fig. 7). In PCA both the distance between two points and the angle between the points and origin are considered to develop a Euclidian geometric map and uncover similarities between the physicochemical properties of the modifiers and the IC50 values. This has been plotted as PC1 and PC2, which are linear combinations of the original variables presented on new axes. Projecting the original multi-dimensional data on a 2D plot provides a method for visually assessing relationships between variables. The wide spread of observations in the score plot indicates that the materials induced a variety of IC50 values for M(LPS), M(IL-4), and M(0) cells (Fig. 7A). Physicochemical properties of the modifiers were obtained through a database.32 These values included lipophilicity parameters, number of hydrogen bond donors and acceptors, number of freely rotating bonds, the enthalpy of vaporization, surface tension, and the polar surface area. Measured values for the zeta potential of the modified liposomes were also included. The IC50 ratio is the ratio of the IC50 value for M(IL-4): M(LPS) cells and indicates the extent of toxicity in one phenotype over the other. The loading plots describe how well the physicochemical properties and the IC50 values correlate with one another (Fig. 7B). The IC50 ratio is aligned with log
D (7.4). log
D is the octanol water distribution coefficient and can be measured at any pH. The IC50 value for M(IL-4) cells is between the projections of the zeta potential of the liposomes and the log
P of the modifiers, which is the octanol–water partition coefficient. The IC50 values for M(0) and M(LPS) cells are aligned with one another and are situated between the number of hydrogen bond donors and the number of freely rotating bonds.
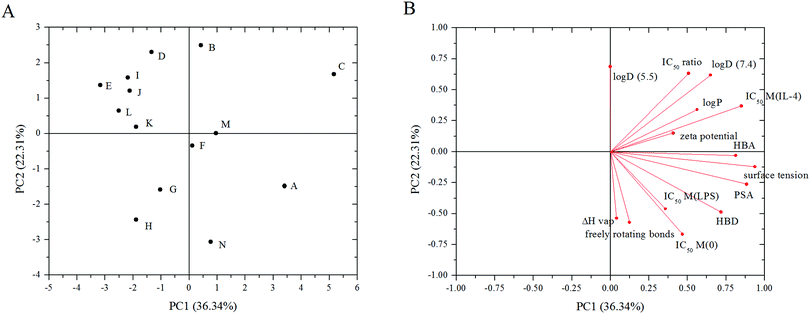 |
| Fig. 7 Observation and loading plots of physicochemical materials properties and their influence on IC50 values of doxorubicin loaded modified liposomes. PC1 explains 36.34% data variance and PC2 explains 22.31% data variance, which represents >50% of the original data information. (A) Observation plots of the modified liposomes and (B) loading plots of the physicochemical properties of the modifiers in PC space. HBD = hydrogen bond donors, HBA = hydrogen bond acceptors, and PSA = polar surface area. | |
4. Discussion
4.1. Liposome properties influenced by surface modification
It has been reported that liposome size and surface charge have a significant effect on potential targeting and encapsulation capability.27,33 Modification of liposomes with the arginine derivatives in Fig. 1 results in changes to the surface charge. Unmodified and all of the modified liposomes explored here are negatively charged, which has been reported to have more efficient delivery than neutral liposomes when encapsulating methotrexate-γ-aspartate.11 Previous studies have reported that the extent of phagocytosis increases with increasing zeta potential, both negative and positive, and was lowest when the zeta potential was zero.11 Several similarities between alterations in the zeta potential value and internalization with FC and IC50 values of encapsulated doxorubicin were observed here. In internalization with FC, with the exceptions of modifications B, F, J, and N, the internalization of the particles by M(0) cells follows the zeta potential with more negative liposome surface charges corresponding to higher FC uptake. Trends between zeta potential and internalization for M(LPS) and M(IL-4) cells were not found. In the IC50 test, M(0) macrophages have increasing cytotoxicity with more negative zeta potential except in the case of modifications C, G, H, I, and L. Attachment of liposomes to cell membranes is the first step of internalization, which is affected by surface charges of particles.34 It is well known that charged particles become opsonized and enter cells through absorptive endocytosis.35 Many positively charged liposomes exert toxic effects on cells,36 thus making negatively charged liposomes, such as those studied here, a more attractive carrier for drug delivery.
There were no significant differences in size between unmodified and modified liposomes after extrusion through 100 nm membranes, which provides uniform diameters and encapsulation volumes. It has been reported that extrusion of liposomes could enhance the entrapment capability.24 Small particle size (<200 nm) is also known to enhance drug accumulation at the tumor site.37 Size has been reported in many studies38–40 as a crucial factor in altering pharmacokinetics due to the impact on tissue distribution and clearance.38 These liposomes were kept around 100 nm to eliminate variables in characterizing drug release profiles and interactions with cells. The high retention of doxorubicin in these liposomes demonstrates similar stability, which has previously been demonstrated as a factor in high drug retention levels in liposomes.27 The loading efficiency showed no significant differences across the library of modified liposomes. Taken all together, liposomal modifications with arginine derivatives have little influence on their size and drug loading ability.
4.2. Influence of macrophage phenotype on internalization and the efficacy of delivered doxorubicin
Macrophages play important roles in creating a permissive environment for tumors, thus targeting drugs to macrophages is an efficient method for drug delivery.11 Targeting macrophages with doxorubicin encapsulated in liposomes is a method of improving the current passive targeting typically used with Doxil®, and increasing intracellular uptake is the main purpose for the future in vivo study with these materials. Doxorubicin is a widely and commonly used drug for cancer treatment. Doxorubicin can intercalate between DNA base pairs, which inhibits DNA and DNA-dependent RNA synthesis by template disordering and steric obstruction.41 Another function of doxorubicin is that it induces the formation of covalent topoisomerase–DNA complex. When copying DNA, this capability could inhibit the religation portion of the ligation–religation reaction. Although doxorubicin contributes to the inhibition of tumor growth, cardiac toxicity is a major side effect. To ameliorate off-target effects, one effective approach is modifying the drug carrier, which could change the biodistribution of doxorubicin, leading to reduced levels of the drug in healthy tissue.
Macrophage phenotype appears to be significant for internalizing liposomes. Lower IC50 levels demonstrate more acute activities of doxorubicin resulting from particle interactions with the cells. The different uptake levels may be influenced by different pathways for the different phenotypes.42 From Fig. 6 it is obvious that M(LPS) cells have lower IC50 values, with the exception of modifications H and I, compared to M(IL-4) cells.
Although the internalization mechanisms of macrophages are complex, the results provide some clues to predict which modifications can be exploited to enhance toxicity of doxorubicin or improve selective delivery to specific macrophage phenotypes. The trend between the IC50 values of doxorubicin and internalization measured through entrapped FC are very similar (R2 = 0.79) with the exceptions of modifications A, B, F, H, and M. A possible explanation for these modifications not following this trend will be discussed in a subsequent paragraph. Higher fluorescence values for modifications F–K, M, and N in M(IL-4) cells (p < 0.05) combined with the decreased IC50 values for the same modifications loaded with doxorubicin suggests that the surface modifications could enhance cellular uptake. The fluorescence measured for M(IL-4) cells incubated with modifications F–K compared to the unmodified liposome is higher than those same modifications incubated with M(LPS) cells compared to M(LPS) with unmodified liposomes. For modifications H, I, and J, the increase is four fold for M(IL-4) compared to 1.5 fold for M(LPS) cells. This suggests that modifications can improve selective delivery to specific macrophages phenotypes.
In examining the IC50 values, the toxicity of doxorubicin is enhanced compared to unmodified liposomes with a few exceptions. For LPS activated macrophages, only modifications A and N are higher than unmodified liposomes (p < 0.05), while modification H and I are similar to unmodified liposomes. For IL-4 activated macrophages, modifications A, B, and C are higher than unmodified liposomes (p < 0.05). For non-activated macrophages, none of modifications have higher IC50's than unmodified liposomes. Thus, it is obvious that modifications to liposomes can enhance the cytotoxicity of encapsulated drugs.
Lipophilicity is an important factor that can lead to a difference in internalization. Lipophilicity can be quantified by measuring the octanol–water distribution coefficient at various pHs (log
D). For log
D's between −2.4 and −1.4, fatty acids activated Epstein Barr virus (EBV), increasing the lipophilicity of the fatty acids to −1.0 to −0.3 decreased activation and activation was blocked for log
D > 0,43 illustrating how lipophilicity can influence biological responses. IC50 values of encapsulated doxorubicin in modifications A, B, F, H, and M, which did not exhibit correlations between internalization of FC loaded particles and doxorubicin IC50 values, appear to be correlated with the log
D at pH 5.5 of the modifier (R2 = 0.77). These modifiers have log
D values in the range of −4.0 to −4.5, with all other modifiers in the library being outside this range. There was no correlation for these materials with log
D at pH 7 (R2 = 0.00). Other factors that are influential in internalization are surface tension and polar surface area. It has been shown that hydrophobic liposomes are more susceptible to phagocytosis than their hydrophilic counterparts.11 There was modest correlation between surface tension and polar surface area with the IC50 values for the above-mentioned modifiers (R2 = 0.48 and R2 = 0.56, respectively). Most modifications have lower IC50 values than unmodified liposomes when comparing similar macrophage activations, indicating improvement of cytotoxicity.
Several mechanisms for endosomal escape have been proposed. The proton sponge effect, also known as the pH-buffering effect, is mediated by agents with a high buffering capacity and the flexibility to swell when protonated.44 An extensive inflow of ions and water induced by protonation into the endosomal environment, results in bursting the endosomal membrane and releasing the encapsulated contents through increases in the osmotic pressure of the endosome.45 Second, the flip-flop mechanism involves exchange of the liposomes in the endosomal wall and the delivered liposome, resulting in endosomal escape into cytosol.46 Third, membrane fusion involves the destabilization of the endosomal membrane due to fusogenic peptides, which is a significant process in cellular delivery and endocytosis.47 When liposomes interact with macrophages, the encapsulated drugs are taken by diffusing out of vehicles or directly “eaten” through lysosomal degradation. Escape from the liposome depends on the drug release rate from liposomes and macrophage permeability to the drugs.40 The mechanism for endosomal escape and the particle's ability to escape may be altered for the different modifications, which may explain the differences between the internalization data and the IC50 values.
PCA was applied to the dataset to determine the relationships between physicochemical properties of the modifiers and the IC50 values. The multidimensional dataset was reduced to a two-dimensional plot (Fig. 7) to better facilitate analysis of latent relationships. The physicochemical properties were chosen based on previous reports describing attributes of drug molecules such as Lipinski's rule of five: polar surface area,48 flexibility,49 enthalpy,50 lipophilicity,51 and charge.51 Macrophages were observed to have different correlations with these physicochemical properties based on their phenotype. The IC50 values for both M(LPS) and M(0) cells were well aligned on the projection map (Fig. 7B) and were situated between the number of hydrogen bond donors and the number of freely rotating bonds of the modifiers. The M(IL-4) cells were dependent on the zeta potential of the liposomes and the log
P of the modifiers. Taken together, these insights may elucidate design principles in drug delivery targeted to specific macrophage phenotypes. Further work on a larger library is necessary to determine if these relationships between the identified materials properties and IC50 values hold for different macrophage polarizations. Other future work includes examining and comparing the pathways responsible for improved toxicity to M(IL-4) cells, seen in modification I, with those pathways involved with the decreased toxicity to M(IL-4) cells, such as modification B.
5. Conclusions
Here, liposomes with surface modifications were studied for their ability to enhance drug delivery. Liposomes were modified with arginine derivatives. These modifications had no influence on particle size and drug loading efficiency. Differences were observed on the measured zeta potentials, which impacts internalization by macrophages. Cellular uptake of the liposomes was found to be dependent upon macrophage phenotype and surface modifications. There were also differences in trends between internalization of liposomal FC and the IC50 of liposomal doxorubicin, which were attributed to changes in the ability of doxorubicin to escape the endosome. Two modifications were able to increase the toxicity of encapsulated doxorubicin for M(IL-4) cells over M(LPS) cells, improving targeted delivery to specific macrophage subpopulations. This work demonstrates the importance of investigating how liposomes interact with different macrophage types and the ability to preferentially deliver drugs to specific phenotypes. The results suggest that liposomes modified with arginine derivations are promising efficient nanoparticle vehicles for delivery to macrophages.
Acknowledgements
This work was supported by the National Science Foundation under Grant No. CBET 1227867 and the Roy J. Carver Charitable Trust Grant No. 13-4265. The authors also acknowledge support from NSF ARI-R2 (CMMI-0963224) for funding the renovation of the research laboratories used for these studies.
References
-
Y. E. Rahman, Liposome Encapsulation of Chelating Agents, 1973 Search PubMed.
- P. P. Wibroe, D. Ahmadvand, M. A. Oghabian, A. Yaghmur and S. M. Moghimi, An Integrated Assessment of Morphology, Size, and Complement Activation of the PEGylated Liposomal Doxorubicin Products Doxil, Caelyx, DOXOrubicin, and SinaDoxosome, J. Controlled Release, 2016, 221, 1–8 CrossRef CAS PubMed.
- H. I. Chang and M. K. Yeh, Clinical Development of Liposome-Based Drugs: Formulation, Characterization, and Therapeutic Efficacy, Int. J. Nanomed., 2012, 49–60 Search PubMed.
- T. M. Allen and P. R. Cullis, Liposomal Drug Delivery Systems: From Concept to Clinical Applications, Adv. Drug Delivery Rev., 2013, 65(1), 36–48 CrossRef CAS PubMed.
- A. C. Li and C. K. Glass, The Macrophage Foam Cell as a Target for Therapeutic Intervention, Nat. Med., 2002, 8(11), 1235–1242 CrossRef CAS PubMed.
- A. A. Tarique, J. Logan, E. Thomas, P. G. Holt, P. D. Sly and E. Fantino, Phenotypic, Functional, and Plasticity Features of Classical and Alternatively Activated Human Macrophages, Am. J. Respir. Cell Mol. Biol., 2015, 53(5), 676–688 CrossRef CAS PubMed.
- P. J. Murray, J. E. Allen, S. K. Biswas, E. A. Fisher, D. W. Gilroy, S. Goerdt, S. Gordon, J. A. Hamilton, L. B. Ivashkiv, T. Lawrence, M. Locati, A. Mantovani, F. O. Martinez, J.-L. Mege, D. M. Mosser, G. Natoli, J. P. Saeij, J. L. Schultze, K. A. Shirey, A. Sica, J. Suttles, I. Udalova, J. A. van Ginderachter, S. N. Vogel and T. A. Wynn, Macrophage Activation and Polarization: Nomenclature and Experimental Guidelines, Immunity, 2014, 41(1), 14–20 CrossRef CAS PubMed.
- E. Y. Lin and J. W. Pollard, Tumor-Associated Macrophages Press the Angiogenic Switch in Breast Cancer, Cancer Res., 2007, 67(11), 5064–5066 CrossRef CAS PubMed.
- A. Mantovani, T. Schioppa, C. Porta, P. Allavena and A. Sica, Role of Tumor-Associated Macrophages in Tumor Progression and Invasion, Cancer Metastasis Rev., 2006, 25(3), 315–322 CrossRef PubMed.
- S. M. Zeisberger, B. Odermatt, C. Marty, A. H. M. Zehnder-Fjällman, K. Ballmer-Hofer and R. A. Schwendener, Clodronate-Liposome-Mediated Depletion of Tumour-Associated Macrophages: A New and Highly Effective Antiangiogenic Therapy Approach, Br. J. Cancer, 2006, 95(3), 272–281 CrossRef CAS PubMed.
- F. Ahsan, I. P. Rivas, M. A. Khan and A. I. Torres Suarez, Targeting to Macrophages: Role of Physicochemical Properties of Particulate Carriers – Liposomes and Microspheres – on the Phagocytosis by Macrophages, J. Controlled Release, 2002, 79(1-3), 29–40 CrossRef CAS PubMed.
- T. Harel-Adar, T. Ben Mordechai, Y. Amsalem, M. S. Feinberg, J. Leor and S. Cohen, Modulation of Cardiac Macrophages by Phosphatidylserine-Presenting Liposomes Improves Infarct Repair, Proc. Natl. Acad. Sci. U. S. A., 2011, 108(5), 1827–1832 CrossRef CAS PubMed.
- A. Etzerodt, M. B. Maniecki, J. H. Graversen, H. J. Moller, V. P. Torchilin and S. K. Moestrup, Efficient Intracellular Drug-Targeting of Macrophages Using Stealth Liposomes Directed to the Hemoglobin Scavenger Receptor CD163, J. Controlled Release, 2012, 160(1), 72–80 CrossRef CAS PubMed.
- C. Rolny, M. Mazzone, S. Tugues, D. Laoui, I. Johansson, C. Coulon, M. L. Squadrito, I. Segura, X. Li, E. Knevels, S. Costa, S. Vinckier, T. Dresselaer, P. Åkerud, M. De Mol, H. Salomäki, M. Phillipson, S. Wyns, E. Larsson, I. Buysschaert, J. Botling, U. Himmelreich, J. A. Van Ginderachter, M. De Palma, M. Dewerchin, L. Claesson-Welsh and P. Carmeliet, HRG Inhibits Tumor Growth and Metastasis by Inducing Macrophage Polarization and Vessel Normalization through Downregulation of PlGF, Cancer Cell, 2011, 19(1), 31–44 CrossRef CAS PubMed.
- D. Akilbekova, R. Philiph, A. Graham and K. M. Bratlie, Macrophage Reprogramming: Influence of Latex Beads with Various Functional Groups on Macrophage Phenotype and Phagocytic Uptake in Vitro, J. Biomed. Mater. Res., Part A, 2015, 103, 262–268 CrossRef PubMed.
- H. C. Bygd, K. D. Forsmark and K. M. Bratlie, Altering in Vivo Macrophage Responses with Modified Polymer Properties, Biomaterials, 2015, 56, 187–197 CrossRef CAS PubMed.
- H. C. Bygd and K. M. Bratlie, The Effect of Chemically Modified Alginates on Macrophage Phenotype and Biomolecule Transport, J. Biomed. Mater. Res., Part A, 2016, 104, 1707–1719 CrossRef CAS PubMed.
- D. Wang and K. M. Bratlie, Influence of Polymer Chemistry on Cytokine Secretion from Polarized Macrophages, ACS Biomater. Sci. Eng., 2015, 150225134407009 Search PubMed.
- A. Mantovani, A. Sica, S. Sozzani, P. Allavena, A. Vecchi and M. Locati, The Chemokine System in Diverse Forms of Macrophage Activation and Polarization, Trends Immunol., 2004, 25(12), 677–686 CrossRef CAS PubMed.
- V. Bronte and P. Zanovello, Regulation of Immune Responses by L-Arginine Metabolism, Nat. Rev. Immunol., 2005, 5(8), 641–654 CrossRef CAS PubMed.
- T. Arimoto and G. Bing, Up-Regulation of Inducible Nitric Oxide Synthase in the Substantia Nigra by Lipopolysaccharide Causes Microglial Activation and Neurodegeneration, Neurobiol. Dis., 2003, 12(1), 35–45 CrossRef CAS PubMed.
- V. Calabrese, G. Scapagnini, A. Ravagna, R. Bella, D. A. Butterfield, M. Calvani, G. Pennisi and A. M. Giuffrida Stella, Disruption of Thiol Homeostasis and Nitrosative Stress in the Cerebrospinal Fluid of Patients with Active Multiple Sclerosis: Evidence for a Protective Role of Acetylcarnitine, Neurochem. Res., 2003, 28(9), 1321–1328 CrossRef CAS PubMed.
- P.-L. Yu, M. L. Cross and R. G. Haverkamp, Antimicrobial and Immunomodulatory Activities of an Ovine Proline/arginine-Rich Cathelicidin, Int. J. Antimicrob. Agents, 2010, 35(3), 288–291 CrossRef CAS PubMed.
- S. E. a Gratton, P. a Ropp, P. D. Pohlhaus, J. C. Luft, V. J. Madden, M. E. Napier and J. M. DeSimone, The Effect of Particle Design on Cellular Internalization Pathways, Proc. Natl. Acad. Sci. U. S. A., 2008, 105(33), 11613–11618 CrossRef CAS PubMed.
- M. Ikonen, L. Murtomäki and K. Kontturi, Microcalorimetric and Zeta Potential Study on Binding of Drugs on Liposomes, Colloids Surf., B, 2010, 78(2), 275–282 CrossRef CAS PubMed.
- K. Abe, K. Higashi, K. Watabe, A. Kobayashi, W. Limwikrant, K. Yamamoto and K. Moribe, Effects of the PEG Molecular Weight of a PEG-Lipid and Cholesterol on PEG Chain Flexibility on Liposome Surfaces, Colloids Surf., A, 2015, 474, 63–70 CrossRef CAS.
- N. Mirahmadi, M. H. Babaei, A. M. Vali and S. Dadashzadeh, Effect of Liposome Size on Peritoneal Retention and Organ Distribution after Intraperitoneal Injection in Mice, Int. J. Pharm., 2010, 383(1-2), 7–13 CrossRef CAS PubMed.
- C. Zhao, Q. Feng, Z. Dou, W. Yuan, C. Sui, X. Zhang, G. Xia, H. Sun and J. Ma, Local Targeted Therapy of Liver Metastasis from Colon Cancer by Galactosylated Liposome Encapsulated with Doxorubicin, PLoS One, 2013, 8(9), e73860 CAS.
- N. Lozano, Z. S. Al-Ahmady, N. S. Beziere, V. Ntziachristos and K. Kostarelos, Monoclonal Antibody-Targeted PEGylated Liposome-ICG Encapsulating Doxorubicin as a Potential Theranostic Agent, Int. J. Pharm., 2015, 482(1-2), 2–10 CrossRef CAS PubMed.
- H. Yamamoto, H. Sakane, H. Yamamoto, T. Michiue and A. Kikuchi, Wnt3a and Dkk1 Regulate Distinct Internalization
Pathways of LRP6 to Tune the Activation of Beta-Catenin Signaling, Dev. Cell, 2008, 15(1), 37–48 CrossRef CAS PubMed.
- J. A. Stefater, S. Ren, R. A. Lang and J. S. Duffield, Metchnikoff's Policemen: Macrophages in Development, Homeostasis and Regeneration, Trends Mol. Med., 2011, 17(12), 743–752 CrossRef CAS PubMed.
- The Free Chemical Database, Royal Society of Chemistry, www.chemspider.com.
- E. Salva, S. O. Turan, F. Eren and J. Akbuga, The Enhancement of Gene Silencing Efficiency with Chitosan-Coated Liposome Formulations of siRNAs Targeting HIF-1alpha and VEGF, Int. J. Pharm., 2015, 478(1), 147–154 CrossRef CAS PubMed.
- S. Patil, A. Sandberg, E. Heckert, W. Self and S. Seal, Protein Adsorption and Cellular Uptake of Cerium Oxide Nanoparticles as a Function of Zeta Potential, Biomaterials, 2007, 28(31), 4600–4607 CrossRef CAS PubMed.
- H. Epstein-Barash, D. Gutman, E. Markovsky, G. Mishan-Eisenberg, N. Koroukhov, J. Szebeni and G. Golomb, Physicochemical Parameters Affecting Liposomal Bisphosphonates Bioactivity for Restenosis Therapy: Internalization, Cell Inhibition, Activation of Cytokines and Complement, and Mechanism of Cell Death, J. Controlled Release, 2010, 146(2), 182–195 CrossRef CAS PubMed.
- C. Kelly, C. Jefferies and S. Cryan, Targeted Liposomal Drug Delivery to Monocytes and Macrophages, J. Drug Delivery, 2011, 2011, 727241 Search PubMed.
- Z. Xu, W. Gu, J. Huang, H. Sui, Z. Zhou, Y. Yang, Z. Yan and Y. Li,
In Vitro and in Vivo Evaluation of Actively Targetable Nanoparticles for Paclitaxel Delivery, Int. J. Pharm., 2005, 288(2), 361–368 CrossRef CAS PubMed.
- J. Gao, W. Zhong, J. He, H. Li, H. Zhang, G. Zhou, B. Li, Y. Lu, H. Zou, G. Kou, D. Zhang, H. Wang, Y. Guo and Y. Zhong, Tumor-Targeted PE38KDEL Delivery via PEGylated Anti-HER2 Immunoliposomes, Int. J. Pharm., 2009, 374(1-2), 145–152 CrossRef CAS PubMed.
- J. Q. Gao, Q. Lv, L. M. Li, X. J. Tang, F. Z. Li, Y. L. Hu and M. Han, Glioma Targeting and Blood-Brain Barrier Penetration Bydual-Targeting Doxorubincin Liposomes, Biomaterials, 2013, 34(22), 5628–5639 CrossRef CAS PubMed.
- X. B. Xiong, Y. Huang, W. L. Lu, X. Zhang, H. Zhang, T. Nagai and Q. Zhang, Intracellular Delivery of Doxorubicin with RGD-Modified Sterically Stabilized Liposomes for an Improved Antitumor Efficacy: In Vitro and in Vivo, J. Pharm. Sci., 2005, 94(8), 1782–1793 CrossRef CAS PubMed.
- S. A. Abraham, D. N. Waterhous, L. D. Mayer, P. R. Cullis, T. D. Madden and M. B. Bally, The Liposomal Formulation of Doxorubicin, Methods Enzymol., 2005, 391, 71–97 CAS.
- B. N. Brown, B. D. Ratner, S. B. Goodman, S. Amar and S. F. Badylak, Macrophage Polarization: An Opportunity for Improved Outcomes in Biomaterials and Regenerative Medicine, Biomaterials, 2012, 33(15), 3792–3802 CrossRef CAS PubMed.
- K. L. Gorres, D. Daigle, S. Mohanram and G. Miller, Activation and Repression of Epstein-Barr Virus and Kaposi's Sarcoma-Associated Herpesvirus Lytic Cycles by Short- and Medium-Chain Fatty Acids, J. Virol., 2014, 88(14), 8028–8044 CrossRef PubMed.
- A. K. Varkouhi, M. Scholte, G. Storm and H. J. Haisma, Endosomal Escape Pathways for Delivery of Biologicals, J. Controlled Release, 2011, 151(3), 220–228 CrossRef CAS PubMed.
- C. Lin and J. F. J. Engbersen, Effect of Chemical Functionalities in Poly(amido Amine)s for Non-Viral Gene Transfection, J. Controlled Release, 2008, 132(3), 267–272 CrossRef CAS PubMed.
- O. Zelphati and F. C. Szoka, Mechanism of Oligonucleotide Release from Cationic Liposomes, Proc. Natl. Acad. Sci. U. S. A., 1996, 93(21), 11493–11498 CrossRef CAS.
- T. Stegmann, Membrane Fusion Mechanisms: The Influenza Hemagglutinin Paradigm and Its Implications for Intracellular Fusion, Traffic, 2000, 1(8), 598–604 CrossRef CAS PubMed.
- P. Ertl, B. Rohde and P. Selzer, Fast Calculation of Molecular Polar Surface Area as a Sum of Fragment-Based Contributions and Its Application to the Prediction of Drug Transport Properties, J. Med. Chem., 2000, 43(20), 3714–3717 CrossRef CAS PubMed.
- D. F. Veber, S. R. Johnson, H. Cheng, B. R. Smith, K. W. Ward and K. D. Kopple, Molecular Properties That Influence the Oral Bioavailability of Drug Candidates, J. Med. Chem., 2002, 45, 2615–2623 CrossRef CAS PubMed.
- C. Rauch, Toward a Mechanical Control of Drug Delivery. On the Relationship between Lipinski's 2nd Rule and Cytosolic pH Changes in Doxorubicin Resistance Levels in Cancer Cells: A Comparison to Published Data, Eur. Biophys. J., 2009, 38(7), 829–846 CrossRef CAS PubMed.
- M. J. Waring, Defining Optimum Lipophilicity and Molecular Weight Ranges for Drug Candidates-Molecular Weight Dependent Lower Log D Limits Based on Permeability, Bioorg. Med. Chem. Lett., 2009, 19(10), 2844–2851 CrossRef CAS PubMed.
Footnote |
† Electronic supplementary information (ESI) available. See DOI: 10.1039/c6ib00133e |
|
This journal is © The Royal Society of Chemistry 2017 |
Click here to see how this site uses Cookies. View our privacy policy here.