DOI:
10.1039/C6FO01475E
(Review Article)
Food Funct., 2017,
8, 15-38
Gastrointestinal interactions, absorption, splanchnic metabolism and pharmacokinetics of orally ingested phenolic compounds
Received
8th October 2016
, Accepted 29th December 2016
First published on 11th January 2017
Abstract
The positive health effects of phenolic compounds (PCs) have been extensively reported in the literature. An understanding of their bioaccessibility and bioavailability is essential for the elucidation of their health benefits. Before reaching circulation and exerting bioactions in target tissues, numerous interactions take place before and during digestion with either the plant or host's macromolecules that directly impact the organism and modulate their own bioaccessibility and bioavailability. The present work is focused on the gastrointestinal (GI) interactions that are relevant to the absorption and metabolism of PCs and how these interactions impact their pharmacokinetic profiles. Non-digestible cell wall components (fiber) interact intimately with PCs and delay their absorption in the small intestine, instead carrying them to the large intestine. PCs not bound to fiber interact with digestible nutrients in the bolus where they interfere with the digestion and absorption of proteins, carbohydrates, lipids, cholesterol, bile salts and micronutrients through the inhibition of digestive enzymes and enterocyte transporters and the disruption of micelle formation. PCs internalized by enterocytes may reach circulation (through transcellular or paracellular transport), be effluxed back into the lumen (P-glycoprotein, P-gp) or be metabolized by phase I and phase II enzymes. Some PCs can inhibit P-gp or phase I/II enzymes, which can potentially lead to drug–nutrient interactions. The absorption and pharmacokinetic parameters are modified by all of the interactions within the digestive tract and by the presence of other PCs. Undesirable interactions have promoted the development of nanotechnological approaches to promote the bioaccessibility, bioavailability, and bioefficacy of PCs.
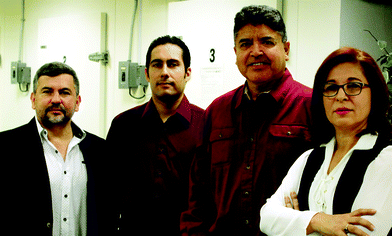 Abraham Wall, Abraham Domínguez, Gustavo González and Maribel Robles | The authors study the health-promoting effects of bioactive compounds from tropical fruits; dietary fiber and polyphenols found in mango pulp are of particular interest. They are currently analyzing the mechanism of action of an improvement in the serum lipid profile, modulation of hepatic and peripheral gene expression and lipoprotein metabolism in rats that is exerted by mango bioactives administered in their diets. The bioavailability, bioaccessibility, metabolism and tissue distribution of phenolics is also an active area of research. Their work is done in collaboration at the Autonomous University of Ciudad Juarez (UACJ), the Research Center for Food and Development (CIAD) and the University of Sonora (UNISON) in northwestern Mexico. |
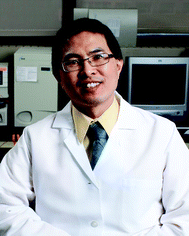 C.-Y. Oliver Chen | Dr Oliver Chen is the scientist of the JM USDA Human Nutrition Research Center on Aging at Tufts University. His research efforts are directed at elucidating health benefits of phytochemicals, as well as functional foods, in the promotion of healthy aging. Further, he is interested in exploring the interrelationship between nutrition and gut bacteria in gut and systemic health. He has published more than 90 scientific articles. He was selected to receive the 2012 Mary Swartz Rose Young Investigator Award, sponsored by the American Society for Nutrition. |
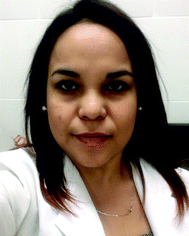 Norma Julieta Salazar-López | Norma Salazar López graduated as Chemist in Food Technology from the Faculty of Chemical and Biological Sciences and has a Master's degree in Food Science from Department of Investigation at the University of Sonora, Mexico. She is currently studying several processes that modify the bioaccessibility of phenolic compounds and its impact on anti-inflammatory and anti-oxidant properties using cell cultures and bioassays as part her PhD project. |
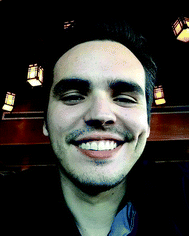 Gustavo R. Velderrain-Rodríguez | Gustavo Ruben Velderrain Rodríguez is a PhD candidate from Mexico. Gustavo studied the career of Biotechnologist Engineering at the Technological Institute of Sonora in Obregon, Sonora, Mexico. He studied a Master's degree at the Research Center for Food and Development (CIAD) in Hermosillo, Sonora. His field of research involves tropical fruits, antioxidants and dietary fiber, and the bioaccessibility and bioactivity of polyphenols under simulated digestion conditions. |
Introduction
Phenolic compounds (PCs) are a diverse group of plant-derived secondary metabolites. Thousands of unique PCs have been identified and classified into different groups including phenolic acids, flavonoids, stilbenes and lignans, although many subcategories exist. The complexity of their chemical structure ranges from simple phenolic acids with low molecular weights to condensed tannins with complex molecular structures and high molecular weights.1 The diversity is increased by the presence of at least one sugar moiety covalently attached to the backbone of the PC. Data produced from decades of scientific research has shown that the consumption of PC-rich diets is associated with a reduced risk of a variety of non-communicable diseases, such as type 2 diabetes, cardiovascular diseases, certain cancers, etc.2–5 However, prior to reaching circulation, PCs interact with a great number of micro- and macromolecules in the gastrointestinal (GI) lumen, enterocytes, and liver, e.g., nutrients in co-consumed foods, digestive enzymes and cellular transporters. All of these interactions reciprocally affect the functionalities of the involved molecules and alter the pharmacokinetic parameters of PCs. The present work will discuss PC interactions within the GI tract, the effects of PCs on the molecules they interact with, and how these interactions alter PC absorption. We will also briefly discuss the novel nanotechnological methods currently being used to enhance PC bioefficacy.
1. Interactions of polyphenolic compounds in the gastrointestinal lumen
PCs are ubiquitously present in plant foods, including fruits, vegetables, whole grains, tree nuts, and spices. Dietary PCs are poorly absorbed mainly due to an array of actions within the GI tract that mediate their transformation and enteric efflux for elimination through stool. Furthermore, interactions with molecules of co-consumed foods interfere with their bioaccessibility for absorption. While the majority of ingested PCs do not reach target tissues at a concentration that allows them to exert bioactions, they can exert health-promoting effects within the GI tract through their interactions with co-consumed nutrients, digestive enzymes and transporters.
1.1. Interactions of phenolic compounds with other nutrients and the consequent health effects.
1.1.1 Interactions with dietary fiber.
PCs in plant foods, including fruits, vegetables, cereals, nuts, and legumes, are predominately present along with indigestible cell wall components, namely fibers. Most consumed PCs remain bound to components of the food matrix, predominantly fiber, and are not readily accessible for digestion and absorption in the upper GI tract.6 The strength and type of binding between fibers and PCs varies widely, primarily depending on the composition of fibers. For example, anthocyanin extractability from grapes (Vitis vinifera L. cv. Tempranillo) differs depending on the composition of cell wall polysaccharides, e.g., cellulose, lignin, etc., which suggests a varied degree of conjugation of PCs with fibers.7 Some fibers, particularly pectin, can form secondary structures, i.e., hydrophobic pockets, to encapsulate PCs.8 Thus, PCs interact with fibers through a combination of hydrogen bonds formed between the hydroxyl groups of PCs and ether bonds of fibers and the hydrophobic interactions that occur within pockets.9 However, due to the varied nature of PCs and fibers, their interactions are not universal and vary according to the chemical structures of both. An example of this has been described by Wang et al. who showed that the in vitro adsorption of flavonoids to oat β-glucan differs depending on the type of flavonoid (flavonol > flavone > flavanone > isoflavone), while structural modifications (hydroxylation, glycosylation, methylation, methoxylation, esterification or galloylation) can widely increase or decrease adsorption.10 Furthermore, PCs can bind to fibers via non-covalent bonds when they are from different food sources. For example, cellulose can rapidly (<1 min) bind with catechin, ferulic acid, chlorogenic acid, gallic acid, and cyanidin-3-glycoside in in vitro studies.11 Because these interactions are largely inevitable in plant foods consumed by individuals, approaches such as industrial food processing and cooking have been identified to make PCs more accessible for absorption in the upper GI tract by weakening these interactions or binding. In vitro cellulase treatment of oat (Avena nuda L.) is able to liberate PCs, significantly enhancing the PC content and antioxidant capacity (as determined by ABTS, DPPH, FRAP and inhibition of protein oxidation).12 In a C57BL6/J mouse model with diet-induced obesity, pretreatment of wheat aleurone with xylanase and feruloyl esterase significantly increased the bioavailability of ferulic acid, which is normally tightly bound to the insoluble arabinoxylan fiber matrix.13 Finally, in a study of healthy men, ferulic acid was found to be more bioavailable in bread made with flour that was pretreated with yeast, xylanase, cellulase, β-glucanase and feruloyl esterase.14
The majority of PCs contained in non-processed plant foods do not reach the circulation because of the previously mentioned interactions with the fibers that transport them to the large intestine. However, the reduced bioavailability of PCs caused by fibers is compensated for by the health benefits of the breakdown products derived from bacterial metabolism in the lower GI tract. For example, Saura-Calixto proposed that antioxidant compounds that are bound to dietary fibers could confer health benefits from their metabolites produced by bacterial metabolism.15 Furthermore, some PCs have been found to possess probiotic characteristics by promoting the growth of beneficial bacteria and inhibiting pathogenic bacteria.16 The beneficial effects of PCs on microbiota are beyond the scope of this review, but they have recently been described by Duda-Chodak et al.17
1.1.2 Interactions with starch.
The study of PC–starch interactions has revealed that starch can form complexes with different PCs.18,19 The mechanism through which PCs interact with starch has been analyzed by X-ray diffraction and appears to depend on the chemical structure of the PC. In the case of catechins in tea, hydrogen bonding is the predominant force, while hydrophobic interactions seem unlikely.20 Interestingly, tannins bind to starch through a combination of hydrogen bonds and hydrophobic interactions.21
PC–starch complexes have been used to protect and transport PCs from various sources. For example, in vitro results suggest that genistein–starch complexes serve to protect genistein from the harsh gastric environment and deliver it to the small intestine where pancreatic amylase hydrolyzes starch to slowly release genistein.18 Similarly, starch protects PCs extracted from yerba mate (Ilex paraguariensis) and preserves their antioxidant capacities.22
Notable consequences of the PC–starch interaction include a decrease in both rapidly digestible starch and slowly digestible starch and, as a consequent, an increase in resistant starch. Rapidly and slowly digestible starches are hydrolyzed by digestive enzymes, while resistant starch remains intact and reaches the large intestine. Accordingly, resistant starch that is produced by its interaction with PCs is functionally similar to dietary fiber and can carry PCs to the large intestine as previously described.23In vitro studies have shown that starches supplemented with blue corn anthocyanins,24 sorghum tannins,25 green tea PCs,26 berry PCs,27 and other sources are able to impede hydrolysis. In healthy men and women (n = 3 and 6, respectively), an inverse correlation was observed between PC content and the glycemic index of pigmented potatoes, thereby suggesting that the formation of complexes between PCs and starch significantly decreases starch digestibility.28 The many health benefits of resistant starches include an increase in satiety, modulation of carbohydrate and lipid metabolism, and several others.29 Bello-Pérez and Paredes-López30 described in detail how resistant starches have various health benefits to consumers and can influence the digestibility of other starches.
1.1.3 Interactions with fat.
Dietary fat and micellization have been identified as key determinants of PC bioavailability and bioaccessibility. This notion has been particularly well documented in several models of quercetin absorption. In rats, quercetin bioavailability increases when it is administered alongside lipids and emulsifiers, and this increase is partially attributed to better quercetin solubility.31 In pigs, an increase in dietary fat results in a concomitant increase in the bioavailability and half-life of quercetin.32 In overweight adults, dietary fat increases quercetin absorption.33 However, this effect may be extended to other flavonoids. The results of in vitro studies show that some, but not all, anthocyanins are affected by dietary fat.34 Thus, the impact of dietary fat on PC absorption is probably dependent on the chemical structure of the PC. A common explanation for the dietary fat-mediated increased in PC bioavailability is the increase in micellization, which may exert a protective effect on PCs that is similar to fiber and starch.35
An increase in bioavailability can consequently increase the bioefficacy of PCs in target tissues other than the GI tract. This has been demonstrated in a rat model in which dietary fat increased the bioavailability of peanut skin PCs and in turn their plasma VLDL- and triacylglycerol-lowering abilities were potentiated.36
1.1.4 Interactions with cholesterol and bile.
PC–cholesterol interactions may decrease cholesterol absorption. The interactions can occur within micelles where cholesterol molecules direct PCs toward the micelle membrane and PCs decrease cholesterol accumulation inside the micelles and its further enteric uptake.37 For example, in vitro data shows that theaflavins decrease cholesterol incorporation into mixed micelles,38 and black tea catechins,39 apple PCs,40 luteolin and quercetin41 displace cholesterol from the micelle. In addition to diminishing cholesterol incorporation into micelles, PCs hinder cholesterol absorption by decreasing its solubility. For example, apple PCs can form insoluble precipitates with cholesterol oxidation products and consequently impede their uptake.40 Tea catechins form hydrogen bonds with bile salts, which are then less available to facilitate cholesterol solubility in the intestinal milieu.42 Dimeric and trimeric catechins from peanut skin can dose-dependently degrade intestinal micelles independently of bile.43 Rat studies have also shown that black tea PCs decrease cholesterol micellar solubility, resulting in decreased lymphatic cholesterol recovery.39 PCs can also hinder the enterohepatic circulation of steroids and favor fecal excretion. Catechins display an in vitro bile-binding capacity, with esterifiable catechins being more effective than unesterified ones.44 These data support the lowering effects of PCs from the pecan nut (Carya illinoinensis) on total- and LDL cholesterol levels in rats fed high-fat diets.45 Thus, PCs from diverse sources (red wine, green tea, grape seed and bilberry) have been favorably compared to hypocholesterolemic drugs.46 Although a substantial amount of literature shows that PCs can decrease cholesterol absorption, studies examining the effects of cholesterol on PC absorption remains lacking.
1.1.5 Interactions with dietary protein.
Since proteins can form hydrogen bonds and hydrophobic interactions with PCs, they are remarkably good at binding to PCs of varied molecular structures and can reach colloidal-size aggregates.47 One of the most commonly known effects of PC–protein interactions is protein precipitation by tannins that results in an astringent sensation.48,49 Astringency due to PC–protein interactions is mediated by the combination of hydrogen bonding and hydrophobic interactions, but it is disrupted when certain carbohydrates (xanthan gum > pectin > gum arabic) strongly bind the PCs first.50 PC–protein interactions with milk proteins have been thoroughly studied. For example, resveratrol, genistein and curcumin interact with α and β caseins through hydrophobic interactions and hydrogen bonds.51 Furthermore, green and black tea catechins with low molecular weights have a strong affinity for casein micelles and alter the secondary structures of the proteins.52
PC–protein interactions are not random because the physicochemical characteristics of PCs and proteins can be used to predict their non-covalent interactions. Binding is stronger when PCs have a higher log
P (a higher log
P indicates hydrophobic nature), a double bond at position 2–3, and a keto group at position 4. Furthermore, the isoelectric point, number of acidic/basic amino acid residues, number of proline residues and secondary structures allow for similar predictions to be made for proteins.53 For catechins, a gallate group increases protein affinity, whereas a pyrogallol group decreases it.52 In the case of PCs binding to milk β-lactoglobulin, affinity increases with the number of hydroxyl groups in the PC.54 However, this is not a general rule because the position of the hydroxyl group is more important than the number in most cases.
PC–protein interactions affect both compounds. In vitro digestion experiments have shown that green tea catechins, specifically epigallocatechin-3-gallate (EGCG), slow down milk protein digestion. The binding of EGCG to milk proteins does not decrease its antiproliferative activity, which suggests that the interaction does not impair EGCG bioactivity.55 However, milk proteins can decrease the antioxidant capacity of green and black tea flavonoids,56 particularly with α-casein which exerts a stronger impact on the antioxidant capacity of smaller PCs than larger ones.57 These results suggest that when a protein binds to a PC molecule, the latter is unable to transfer electrons, hydrogen atoms or both. As previously mentioned, antioxidant capacity is one of the most recognized characteristics of PCs, but it also seems to be necessary to prevent the oxidation of essential nutrients before their uptake in the upper GI. Thus, a decrease in their antioxidant capacity can indirectly affect oxidation-labile nutrients. For example, green tea catechins incorporated into micelles prevent linoleic acid (ω-6 fatty acid) peroxidation58 and regenerate α-tocopheroxyl radicals (oxidized α-tocopherol) into α-tocopherol,59 when present within sodium dodecyl sulfate (SDS) micelles in both instances. The oxidation of ω-3 fatty acids (eicosapentaenoic and docosahexaenoic acid) is also decreased by PCs from grape seed extracts.60 It is logical that PCs would be sacrificed to maintain tocopherols and ω-6/ω-3 fatty acids in a reduced state since both are indispensable and PCs are not.
PC–protein interactions confer protection to the PC. Therefore, dairy matrices such as yogurt and cheese have been used for these purposes.61 For example, Cheddar,62 Chihuahua,63 Gouda,64 Pecorino65 and other66,67 cheese types have been used as delivery vehicles for PCs. However, such incorporation also modifies the cheese-making ability of milk and alters sensory parameters.66,68
1.1.6 Interactions with metallic ions.
PCs are able to chelate metallic ions in the GI, thereby decreasing metal-catalyzed free radical production. However, the decreased bioavailability of the PCs and the metals is a notable disadvantage. In vitro digestion experiments have shown that chlorogenic acid and rutin from potato and sweet potato form complexes with iron that result in the decreased bioavailability of both PCs.69 Quercetin in synthetic wine forms complexes with iron that remain intact after the gastric phase of in vitro digestion. Presumably, the iron binds to at least two sites where the hydroxyl groups of quercetin are located.70 Gallic acid, caffeic acid, catechin and rutin can chelate copper ions with higher affinities than iron.71 Zinc chelation by sorghum PCs in healthy adults does not occur unless phytic acid is present,72 while studies of Caco-2 cells show that grape seed extract hinders zinc uptake due to strong binding by procyanidins.73 However, the ion chelation of PCs is strongly dependent on pH and varies between gastric and intestinal conditions.74
Although the micronutrient chelation properties of PCs can raise concerns of nutrient deficiencies, minimal effects on iron, copper and zinc status in the liver have been reported in piglets fed diets supplemented with PC-rich products (1% grape marc meal extract or spent hops).75 A null effect on the status of serum zinc or copper in rats was reported when they were fed red wine, which is rich in PCs, for either a 3 or 28 d period.76
1.2 Effects on digestive enzymes.
As previously discussed, PCs can strongly interact with proteins. Therefore, interactions with the main digestive enzymes are expected, resulting in delayed/decreased macronutrient digestion and absorption. However, it should be noted that not all PC–enzyme interactions will result in a marked inhibition of enzyme activities because PCs may not bind to the active site, or the molecular ratio of the PCs to enzyme may be too low to cause a significant reduction.77
1.2.1 Effects on amylase.
In vitro
78–83 and in vivo84–86 studies have shown that PCs can inhibit α-amylase, and this inhibition can delay the digestion of carbohydrates and/or decrease absorption. PC-rich extracts from finger millet inhibit the amylases developed during malting through mixed non-competitive inhibition, while individual PCs isolated from the extracts (gallic acid, vanillic acid, quercetin and trans-cinnamic acid) act through uncompetitive inhibition, thus showing that inhibition kinetics vary among different PCs.87 Efforts to understand the underlying mechanisms through which PCs bind to and inhibit amylase have been reviewed elsewhere.88 However, molecular characteristics such as hydroxylation, unsaturation, and ideal carbohydrate moiety positioning allow for optimal inhibition, whereas methylation, methoxylation, isomerism, conjugation and others hinder the amylase-inhibiting properties of PCs. In the case of catechins, the presence of a galloyl group rather than a non-galloylated group favors the inhibition of amylase.89 A recent study has shown that only catechins with a galloyl moiety at position 3 exhibit significant α-amylase inhibition. Furthermore, the inhibition by some compounds (EGCG, theaflavin-3,3′-digallate and tannic acid) is competitive, while others (epicatechin gallate, theaflavin-3′-gallate and theaflavin) exhibit mixed-type inhibition.90
Because of the α-amylase-inhibiting properties of PCs, their antidiabetic potential has been compared to acarbose,91,92 a commercially available medication that acts through α-amylase inhibition. In fact, flavonoids are considered to be glucose-lowering agents because they exert acarbose-like effects without negative side effects.93 Most significantly, a synergistic effect has been demonstrated when administering acarbose alongside PCs from berries,94 green tea,78 black tea95 and other96 sources. The health benefits of PCs that inhibit α-amylase in the GI tract are related to a decrease in glucose absorption that in turn impacts insulin secretion, potentially improving glucose/insulin metabolism at the whole organism level.
1.2.2 Effects on lipase.
Lipase hydrolyzes triacylglycerols in the GI prior to their uptake, and their inhibition results in delayed/decreased fatty acid absorption and reduced postprandial lipemia.97,98 Several PC-rich sources have exhibited in vitro lipase-inhibiting properties, including black tea catechins,99 oolong tea,100 black chokeberries (Aronia melanocarpa L.),101 kiwi fruit (Actinidia chinensis L.),102 litchi flower (Litchi chinensis Sonn.)103 and peanut shells.104 Black tea catechins can also exert in vivo lipase inhibition in rats, which consequently decreases postprandial hypertriglyceridemia.105
Luteolin and chrysoeriol extracted from Tacoma stans (a medicinal plant used by some ethnic groups in Mexico to treat diabetes)106 and acteoside from Ligustrum purpurascens (consumed in China as an infusion to aid in weight loss)107 inhibit lipase in vitro, which may underlie the reported health effects of these and likely other medicinal plants.
The lipase-inhibition mechanism has been described as a competitive inhibition in the case of flavonoid and non-flavonoid PCs. For example, chlorogenic acid and caffeic acid exhibit a competitive inhibition against rice bran lipase activity in vitro, which occurs through a combination of hydrogen bonds and hydrophobic interactions.108 Noble muscadine grape extracts, cyanidin and cyanidin-3,5-diglucoside competitively inhibit pancreatic lipase in vitro.109 Mate tea also exhibits competitive inhibition against porcine and human pancreatic lipase.110 Molecular characteristics such as the number and position of hydroxyl groups, the degree of polymerization and carbohydrate conjugation seem to be structural features that modulate the lipase inhibition activity mediated by PCs.111 Furthermore, the presence of a galloyl moiety appears to strongly favor lipase inhibition.105,112 PCs have been favorably compared to orlistat, a commercially available lipase inhibitor with numerous side effects.111,113 However, the effectiveness of orlistat is significantly higher than most of the PCs evaluated, as is the case with PCs extracted from mango cv Ataulfo (tannic acid, penta-O-galloyl-β-D-glucose and mangiferin) that inhibit lipase activity to a lesser extent than orlistat (data not yet published).
1.2.3 Effects on proteases.
PCs can bind to and inhibit pepsin, trypsin and chymotrypsin activity. The binding of EGCG to pepsin occurs through hydrophobic and electrostatic interactions, which result in non-competitive inhibition.114 The inhibitory effect of EGCG on trypsin and α-chymotrypsin is attributed to alterations of their secondary structures that are triggered by high-affinity EGCG binding.115 Catechins can also inhibit trypsin by binding to the active site, which alters the enzyme's 3D structure and activity.116 Tannic acid exhibits modest trypsin inhibition through a combination of competitive and noncompetitive inhibition via hydrophobic and electrostatic interactions.117 Other sources of PCs, such as acteoside, osmanthuside and ligupurpuroside A, B and C from the Kudingcha bitter tea (Ligustrum purpurascens), are also reported to be non-competitive inhibitors of pepsin, trypsin and α-chymotrypsin, but their mechanisms of action have not been described in detail.118 Because of the inhibitory effects that PCs exert on digestive proteases and the previously mentioned binding to dietary proteins, protein digestion is delayed.119
1.3 Effects on intestinal nutrient transporters.
Another mechanism through which PCs influence the digestive process is by interacting with nutrient transporters. Some PCs, such as tiliroside, inhibit glucose transport through both GLUT2 and SGLT1 in Caco-2 cells, having more of an effect on the latter.120 Quercetin and isoquercetin inhibit GLUT2 expressed in Xenopus laevis oocytes through non-competitive kinetics, but they have no effect on GLUT5 or SGLT1.121 Others have confirmed that there are no inhibitory effects of quercetin on either GLUT5 or SGLT1 in the same X. laevis oocyte model.122 Green tea PCs inhibit SGLT1 in everted jejunal sacs from male Wistar rats through a competitive mechanism.123 Quercetin-3-O-glucoside and quercetin-4′-O-glucoside inhibit SGLT1 in a brush-border-membrane vesicle model from pig jejunum, while quercetin-3-O-galactoside, quercetin-3-O-glucorhamnoside, quercetin aglycone, naringenin-7-O-glucoside, genistein-7-O-glucoside and cyanidin-3,5-O-diglucoside had no effect on SGLT1. These results highlight how the carbohydrate moiety and experimental models can produce contrasting results.124
PCs interact with Niemann-Pick C1-Like 1 (NPC1L1), the main intestinal cholesterol transporter, and directly prevent the uptake of dietary cholesterol and modulate its expression. Evidence obtained from a screening of several PCs indicates that liquiritigenin, sakuranetin, isosakuranetin, hesperetin, apigenin, luteolin, quercetin, daidzein, coumestrol, phloretin, and (−)-epicatechin gallate significantly inhibit cholesterol uptake in Caco-2 cells, while further experiments revealed that quercetin significantly decreases the mRNA expression of NPC1L1 in the same cell line.41 Another study has shown that incubation of Caco-2 cells with curcumin significantly decreases NPC1L1 mRNA and protein expression,125 an effect that is mediated by the modulation of transcription factor sterol regulatory element-binding protein 2 (SREBP2).126 Other authors have observed similar results regarding NPC1L1 modulation using different foods such as black chokeberries (rich in anthocyanins)127 and persimmon (Diospyros kaki L., rich in tannins).128
Taken together, the current literature points to PCs being important modulators of macronutrient digestion through direct binding, enzyme inhibition and transporter inhibition. However, no single molecule is capable of mediating all of these effects; therefore, ideally, dietary habits should include the consumption of PCs from various sources to accomplish the synergistic effect of the different PCs to maximize their potential health effects.
Fig. 1 shows a graphical depiction of the described luminal interactions between nutrients and enzymes.
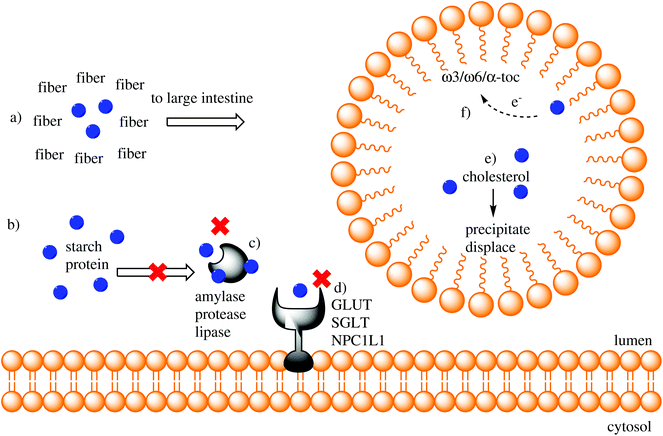 |
| Fig. 1 Gastrointestinal interactions of phenolic compounds (PCs) with other nutrients, digestive enzymes and nutrient transporters. (a) PCs (blue spheres) bound to fiber material in the lumen of the small intestine are not bioaccessible and are carried with it to the large intestine where bacterial metabolism may take place; PCs may delay starch and dietary protein digestion by (b) directly binding to them, (c) inhibiting digestive enzymes or (d) inhibiting transporters; (e) when PCs bind to cholesterol, it will be displaced from the micelle or insoluble precipitates may form, which hinders cholesterol absorption; (f) PCs bound to the micelle membrane can act as antioxidants, aiding in reducing ω3/ω6 fatty acids or α-tocopherol. | |
2 Mechanisms of transport into the enterocyte
After the previously discussed interactions take place in the GI lumen, orally ingested PCs (and other xenobiotics) must pass through the gut epithelia before reaching the bloodstream and/or lymphatic system.129 Bioaccessibility of PCs is critical for their enteric and systemic bioavailability,129,130 which is determined by many factors including PC type, the amount in edible sources, the nature of its matrix and several host-related factors.131 Once released, both hydrophilic (e.g., phenolic acids or glycosylated PCs) and lipophilic (e.g., procyanidin or prenylated PCs) PCs are transported by the various mechanisms that have been previously characterized for other xenobiotics and nutrients.132 Transcellular mechanisms such as passive diffusion (PD), carrier-mediated active (AT) and facilitated (FT) transport and paracellular transport in tight junctions (TJ) are the most recognized,133,134 and all of them make a particular contribution to PC transport and homeostasis, as presented in Fig. 2.
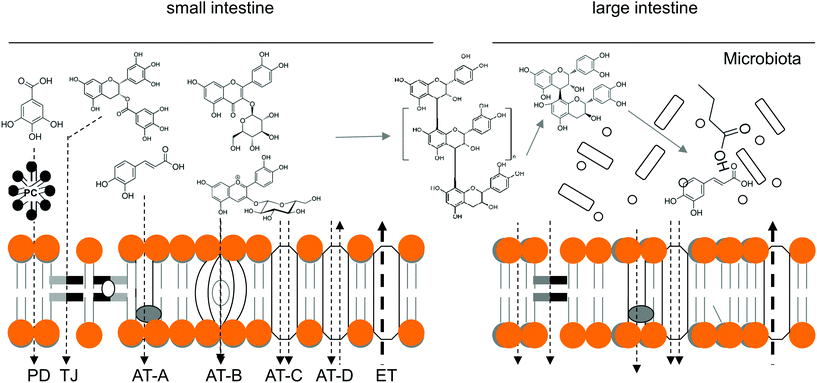 |
| Fig. 2 Hypothetical transport mechanisms for phenolic compounds (PCs). Passive diffusion (PD), paracellular (TJ), single-solute (AT-A), translocase-type (AT-B) and co-solute symporter (AT-C) and antiporter (AT-D) active transporters, efflux transport (ET). | |
Transcellular transport is mediated by transmembrane pumps, channels, and carriers expressed in a polarized fashion, while paracellular transport is driven by solute gradients.133 Transport out of epithelial cells is further facilitated by serosal-facing (basolateral) membrane transporters135 that deliver both free and vesicle-contained PCs into the bloodstream and lymphatic system or efflux them back to the lumen. However, the novel transporters and mechanisms for the intestinal handling of PCs and the inhibitory action of PCs on well-known transporters have been elucidated in recent years,132 which has helped to complete our understanding of the first-pass metabolism of PCs.136 In the following paragraphs, we will attempt to summarize the main transporting mechanisms involved in the transepithelial uptake and efflux of PCs in and out of the enterocyte.137,138
2.1 Passive diffusion (PD).
PCs with small molecular weights that are hydrophobic (log
P > 2.0) or neutral are mainly transported by PD.139,140 As in the case with dietary fats and fat-soluble vitamins,141 the luminal processing of hydrophobic PCs such as alkylated-PCs or procyanidin heterodimers involves bile salt-emulsification, micellar formation and apical membrane translocation.142 Experimental data using Caco-2 cells (TC7 cell line) has shown that several PCs in virgin olive oil (e.g., hydroxytyrosol, tyrosol, p-coumaric acid, apigenin and luteolin) are partitioned in oil/water mixtures and their further absorption (mainly by PD) is different.143 However, PD also depends on many other factors including the interaction of the PCs with the mucous-layer and their size.133 For example, Rastogi and Jana144 evaluated the possible bi-directional transport of caffeic and gallic acids, chrysin, quercetin, resveratrol and rutin in Caco-2 cells and observed a greater permeability in the absorptive direction; therefore, they were more likely to be absorbed for further first-pass metabolism than transported back (efflux) to the lumen. Additionally, free ferulic acid permeates by PD (log
P = 1.5) that is not dependent on its apical concentration, but it does not permeate by transcellular (i.e., TJ) transport.145
Highly complex PCs such as hydrolyzable (e.g., gallotannins and ellagitannins) and condensed (e.g., EGCG and other oligomeric proanthocyanidins) tannins must be hydrolyzed to smaller molecules (tri, di and monomers) to be absorbed through any transporting mechanisms. Because the human small bowel is not prepared to hydrolyze them, they pass through intact to the large bowel and are fermented by the resident microbiota.146,147 Once hydrolyzed (Fig. 2) and before being metabolized by the gut microbiota, monomeric PCs are absorbed through PD by colonic cells.148 Genistein and daidzein (isoflavones) are transformed by the colonic microbiota into dihydro metabolites that are transported by PD according to the pH-partition (proton-gradient) hypothesis and also by breast-cancer resistant protein (BCRP), a member of the ABC transporter proteins.129,139
2.2 Paracellular transport.
Hydrophobic (log
P > 2.0) and neutral PCs and other nanoparticles can easily pass between the narrow gaps (i.e., TJ) that separate the neighboring enterocytes149 by certain proteins called claudins. These proteins are distributed along the apical-basal axis and confer to TJ a particular electrical resistance that varies by 100
000-fold between “tight” and “leaky” epithelia.133 This is the reason why PCs with a small mass that are charged, such as chlorogenic, gallic and rosmarinic acids, can use this transport system, although their permeation rate is low (5 mM), and the non-absorbed molecules travel directly to the large bowel.150,151 It seems likely that EGCG is also absorbed intact via TJ,134 as its Caco-2 permeability (nmol min−1 mg−1 protein) is similar (0.22) to gallic acid (0.16). However, a low degree of discrimination for different-sized PCs suggests the presence of large aqueous spaces in TJ133 that provide a more fluid media for their gradient transport.
TJ transport seems to compensate for the inadequate expression (region-dependent) of active transporters and epithelial damage. Stephens et al.152 evaluated the ex vivo illeal transport of paclitaxel (log
P = 3.96), propranolol (log
P = 3.0) and digoxin (log
P = 1.3) in P-gp-deficient and wild-type mice. Their results suggest that differences in absorption between wild-type and knockout mice are at the level of transcellular permeability, and the ileum and distal colon are regions of relatively high transcellular permeability for xenobiotics that are compensated for by the enhanced expression of P-gp. Additionally, EGCG, myricetin, quercetin and genistein (log
Ps ranging from 1.2 to 2.7) exhibit protective effects on intestinal TJ against oxidative stress, acetaldehyde, enteric bacteria and inflammatory cytokines;153 therefore, their potential TJ transport is plausible.
2.3 Active (AT) and facilitated (FT) transport.
Most pharmacokinetic studies aim to define the AT/FT of PCs to design efficient drug-delivery systems.132 AT utilizes different energy-coupled mechanisms that create ion/solute gradients across membranes, while FT passes solutes across membranes (apical/basolateral) down their electrochemical gradient and does not use energy at all.129 However, it is well recognized that the specificity of these particular substrates for individual transporters is not as specific as it is for enzymes.129,141 In the case of PCs, most of them are either absorbed as glycosides or modified by the brush border and bacterial enzymes [e.g., β-glycosidases (β-GS), lactase-phloridzin hydrolase (LPH)] before being absorbed as aglycones. From a structural stand point, AT/FT is divided into the following three categories: (A) glycosylated PCs, (B) cationic aglycones and (C) neutral or hydrophobic aglycones, as shown in Table 1.
Table 1 Intestinal transporters of PCs
Transporter |
Expression |
Direction |
Area |
Substrate specificity |
PC |
Ref. |
Abbreviations: amphipathic (AMP), broad specificity (BSE), phase II-conjugated PC (PCP), glycosylated PC (GPC), hydrophobic (HPB), inhibition (I), ionic PC (ION), systemic circulation (SC), transport (T). |
Glycosylated-PCs
|
GLUT2 |
High |
AP-BA-SC |
Basolateral |
GPC |
(I) quercetin, quercetin-3-O-glycoside, EGCG, fisetin, myricetin and gossypin |
130 and 135
|
SGLT1 |
High |
AP-BA |
Apical |
GPC |
(I) catechin, EGCG |
129, 130 and 226
|
Ionic aglycones
|
MATE1/SLC47A1 |
Med |
AP-BA |
Apical |
ION (cation) |
(T) quercetin and metabolites |
129, 130 and 158
|
MATE2 |
Low |
AP-BA |
Apical |
ION (cation) |
— |
129 and 130
|
MCT1/SLC16A1 |
Low |
AP-BA |
Apical |
AMP |
(I) p-coumaric, ferulic, gallic, gentisic, 4-hydroxybenzoic, protocatechuic, sinapic, syringic and vanillic acids |
129, 130, 150 and 156
|
MCT4/SLC16A3 |
— |
AP-BA |
Apical |
AMP |
— |
129 and 130
|
OCT1 |
Med |
AP-BA |
Basolateral |
ION (cation) |
(I) red and white wine, grapefruit juice, naringin, naringenin, and bergamottin, phloretin and quercetin |
129, 130, 159 and 160
|
OCT2 |
Med |
AP-BA |
Basolateral |
ION (cation) |
— |
129 and 130
|
OCTN1 |
— |
AP-BA |
Apical |
ION (cation) |
(I) phloretin and quercetin |
130 and 161
|
Efflux
|
BCRP/ABCG2/MXR |
Med |
Efflux |
Apical |
BSE, ION (anion) |
(I) quercetin, coumestrol and isoflavones |
129, 130 and 139
|
MDR1/ABCB1/P-gp |
Med |
Efflux |
Apical |
BSE, ION, AMP, HPB, PCP |
(T) flavonoids, (I) grapefruit juice |
129 and 130
|
MRP1/ABCC1 |
Med-high |
Efflux |
Apical |
ION (anion), PCP |
(T) quercetin, naringenin, (I) grapefruit juice |
129 and 130
|
MRP2/ABCC1 |
Med-high |
Efflux |
Apical |
ION (anion), PCP |
(T) quercetin, naringenin, (I) grapefruit juice |
129 and 130
|
The “classical model of sugar absorption” involves the high-affinity, sodium-dependent and phloridzin-sensitive sodium/glucose co-transporter 1 (SGLT1; depicted in Fig. 1 as AT-C) located on the apical side of the brush border membranes and also glucose transporters (GLUT, depicted in Fig. 1 as AT-B) 2 and 5, which can be translocated upon leptin signaling to either the apical or basolateral membrane.135 AT is used by PC glycosides such as anthocyanins because their PD is less probable.140,154 However, glucose transport by SGLT1 and GLUT-2 may be inhibited by flavonols and green tea catechins (Table 1) through competitive and non-competitive mechanisms; therefore, these PCs must be transported as glycosylated PCs92 or otherwise supplemented with aglycones or unsweetened beverages rich in these PCs to exert antidiabetic effects.92,135,155
Organic cation (OC) translocases move positively and negatively charged molecules according to their electrochemical gradient, and most of them belong to the solute carrier (SLC) and amphiphilic solute facilitator (ASF) families. The influx (apical-basolateral) absorption of small anionic PCs in particular can be absorbed not only by PD but also by these transporters (Table 1) since the role that organic anion transporters (OAT) play in the intestinal absorption of xenobiotic substances is considered negligible.129 Monocarboxylate (MCT) 1–4 transport OCs such as lactate and pyruvate by a proton-coupled transport mechanism. MCT1 (SLC16A1) and MCT4 (SLC16A3) are expressed in the small bowel (at low levels) and large bowel (at high levels)156 and transport quercetin137 and ferulic acid.157 Under physiological conditions, ferulic (194 g mol−1) and p-coumaric (164 g mol−1) acids with a higher hydrophobicity (log
P = 1.5) are more readily absorbed by MCT1 in Caco-2 cells than gallic acid, which is mostly absorbed by PD (170 g mol−1, log
P = 0.7 (ref. 150)). MATE1, which is highly expressed in the liver and intestine and located in the apical membranes, peroxisomes and endoplasmic reticulum, transports quercetin and enhances glucose uptake.158 It is unknown whether MATE2, which is expressed at lower levels in the small bowel, performs the same duties.
Organic cation transporter 1 (OCT1) and 2 (OCT2) are localized to the basolateral membranes and transport small-mass, hydrophilic OCs and PCs. However, red and white wine and grapefruit juice, as well as naringin, naringenin, and bergamottin, interfere with the xenobiotic transport of OCs.159,160 An acidic GI milieu may promote solute and taurocholate uptake by OATP2B1, but its substrate specificity is narrower than OATP1A2.129 OCT1 is inhibited by fruit juice flavonoids such as phloretin and quercetin through non-competitive mechanisms.161 It is noteworthy that ionic PCs may suffer a nucleophilic attack along the GI tract (e.g., procyanidins); therefore, they must be protected from harsh GI conditions,131 possibly through rapid AT by one or more of the above transporters. Lastly, micelle-driven PD is a well-known mechanism for lipophilic PCs, but the ongoing dilution of hydrophobic PCs (and other xenobiotics) rapidly reaches supersaturation of intestinal colloids, thereby promoting their absorption by many other mechanisms.162 Transporters that have been previously identified and characterized for fatty acids [fatty acid translocase/cluster of differentiation 36 (FAT/CD-36)] and cholesterol/fat-soluble vitamins [NPC1L1 and scavenger receptor class B member 1 (SRB1)] may be involved in the apical absorption of long-chain alkyl-PCs such as catechols or steroid-like PCs such as isoflavones163 because it is well known that their dietary supplementation modifies the concentration of blood lipids in a structure-specific, competent way.164 However, there are very few reports regarding this phenomenon. Nevertheless, as has been observed with other ionic transporters, significant competitive interactions for hydrophobic PCs that are similar to those between the fat-soluble vitamins A, D, E and K may occur.141
2.4 Efflux transport.
Intracellular homeostasis and the deleterious accumulation of xenobiotic substances such as PCs are controlled by their influx (AT, FT) and efflux (AT). The most investigated efflux transporters in the intestine are P-glycoprotein (P-gp/MDRP1), multidrug resistance protein 1, 2 (MRP 1,2) and breast cancer resistance protein (BCRP), and these transporters are also involved in the efflux of the PC aglycone. P-gp transports hydrophobic, amphipathic and cationic molecules in the basal-to-apical and blood-to-lumen directions, and its secretion varies across different segments of the intestine.129 It transports flavonoids back to the lumen,165 but other fruit flavonoids such as those found in grapefruit juice159 and monoglycerides129 may affect P-gp pharmacokinetics. MDRP1 is highly expressed in the small and large intestine. It is localized to the basolateral membrane and transports substances from the enterocyte into the interstitial fluid rather than to the lumen. MDRP1 prefers anionic compounds, and its inhibition by PCs is poorly understood. MDRP2 is mainly located at the tip of jejunal enterocytes, and its substrate affinity overlaps with P-gp. It excretes flavonoids such as quercetin and naringenin back to the lumen165 and is also inactivated by grapefruit flavonoids.
3 Splanchnic metabolism of phenolic compounds
Absorbed PCs (and other xenobiotics) are subjected to phase I and II metabolism and then either transported into circulation or excreted back into the intestinal lumen. This mechanism affects their bioactivity, their mRNA/protein expression and the activity of the enzymes that metabolized them, thereby regulating their own and other molecules’ metabolism.166,167
3.1 Effects on phase I enzymes and pharmacokinetic parameters of other xenobiotics.
Phase I enzymes are those of the cytochrome P450 (CYP450) superfamily that are expressed in the liver, small intestine, and other tissues,168 and they increase the reactivity of their substrate through oxidation. CYP3A4 is one of the most abundant and relevant CYP450 members, and it is expressed in the small intestine where it accounts for approximately 80% of total CYP450. Other isoforms such as CYP2C9, CYP2C19, CYP2J2 and CYP2D6 are also expressed.169 Because CYP450 metabolizes ingested xenobiotics, a failure to maintain appropriate enzymatic activity can lead to potentially serious drug–drug or drug–nutrient interactions.170,171
Sixty PCs from different subcategories were analyzed for their potential CYP3A4 and CYP2C9 inhibitory activities in vitro. Anthocyanins, flavonoid glycosides and methylated catechins showed minimal inhibition, but several compounds (bergamottin, imperatorin, dicoumarol, proanthocyanidin A2, naringenin, phloretin, amentoflavone, chrysin, apigenin, luteolin, acacetin, galangin, kaempferol, quercetin, myricetin and isorhamnetin) significantly inhibited both enzymes.172 Amentoflavone in particular had the lowest IC50 values for CYP3A4 (0.07 μM) and CYP2C9 (0.03 μM).172 Others including quercetin, rutin, gallic acid and ellagic acid inhibited CYP3A4, and their inhibitory potential decreased according to the order listed. The following PCs are listed based on their decreasing inhibitory effects on CYP2D6: gallic acid, quercetin, ellagic acid and rutin. The inhibitory potential was assessed using in vitro assays in both instances.173 Using human liver microsomes and a recombinant system, gallic acid has been shown to inhibit CYP3A4 activity (IC50 > 600 μM), mostly through its spontaneous oxidation products. Therefore, antioxidants that maintain a reduced state prevent gallic acid-mediated CYP3A4 inhibition.174 Tea infusions prepared from the Artemisia annua (annual wormwood) plant contain rosmarinic acid and chlorogenic acid as its main PC content. Some but not all extracts prepared from A. annua inhibit CYP3A4 and CYP1A1 in Caco-2 cells, but neither rosmarinic acid nor chlorogenic acid produce the same inhibitory effects, which indicates that the PC content from A. annua does not inhibit these enzymes.175
Important pharmacokinetic effects have been documented due to the PC-induced inhibition of the intestinal (and/or hepatic) CYP450 system. One of the most recognized is the grapefruit effect in which bergamottin and other furanocoumarins present therein inhibit CYPs, which leads to altered therapeutic effects of drugs, such as prasugrel (platelet inhibitor),176 statins (hypocholesterolemics),177 fexofenadine (antihistaminic),178 pirfenidone (antifibrotic)179 and others. An IC50 of 1.97 μM for quercetin and 7.8 μM for myricetin on rat CYP3A4 has been reported with a consequent increase in area under the curve (AUC) and peak plasma concentration (Cmax) of the antineoplastic doxorubicin.180,181 Quercetin increases the Cmax, AUC0–24 and half-life (t1/2) of valsartan (antihypertensive) when both compounds are concomitantly administered to rats. The authors of this study suggest that the inhibition of P-gp and possibly CYP3A4 are responsible for the pharmacokinetic effects exerted on valsartan.182 Mice fed 3% green tea PCs for four weeks show no difference in mRNA/protein expression or enzyme activity of CYP3A11, CYP3A13, CY3A16 and CYP3A25 in the small intestine, but a significant decrease was observed in liver. This decrease was reflected by the higher plasma concentration of triazolam (a CYP3A substrate) compared with the control.183 Baicalein is a flavonoid present in Scutellariae radix that can inhibit CYP3A4 and P-gp in rats resulting in an increased Cmax and AUC of orally administered nimodipine (a Ca2+ channel blocker). The same effect was not observed when the drug was administered intravenously.184 Tea PCs and daidzein (but not caffeine) increase the AUC and t1/2 of lansoprazole (a proton pump inhibitor) when administered to rats, a finding that the authors propose is due to CYP3A inhibition.185
The described alterations in drug metabolism can sometimes be considered beneficial, as the parallel consumption of CYP-inhibiting PCs will allow the patient to take a smaller dose, and in the case of doxorubicin, this can lessen side effects. However, potential toxicity can occur with any drug if the dose is not adjusted by the physician, especially for drugs with low therapeutic indexes. Although this concept has been considered extensively for drug–drug interactions over the past few decades,186 it merits further study for nutrient–drug interactions because of the ubiquity of PCs in vegetables.
3.2 Effects on phase II enzymes.
Phase II enzymes can conjugate several groups to their substrates, such as glucuronic acid (UDP-glucuronosyltransferase, UGT), sulfate (sulfotransferase, SULT), glutathione (glutathione-S-transferase, GST) and others. This will typically decrease the bioactivity and potential toxicity of the compounds, while also increasing their polarity and facilitating excretion.
UGT1A1 mRNA expression and glucuronidation activity are increased by resveratrol and resveratrol combined with either curcumin or chrysin in Caco-2 cells.187 The glucuronidation of acacetin, a flavonoid aglycone, is significantly faster than its glycoside tilianin in enterocytes and hepatocytes from both genders of different species (humans, rats, mice, dogs and guinea pigs), thereby demonstrating that the carbohydrate moiety is a determining factor in how PCs are metabolized.188 Quercetin and other flavonoids stimulate the expression of UGT mRNA through AhR (aryl hydrocarbon receptor) activation, a mechanism that evolved to prevent xenobiotic toxicity in animals.189 Baicalein and 3-hydroxyflavone upregulate UGT1A1 mRNA expression in LS180 cells, while also increasing UGT1A protein expression and activity in a dose-dependent manner. The effect of baicalein was through AhR induction, and the effect of 3-hydroxyflavone was through pregnane X receptor (PXR) induction.190 In healthy humans, perfused (−)-epicatechin is conjugated to (−)-epicatechin-3′-O-sulfate (and other sulfated conjugates) and shunted back into the intestinal lumen as a method of excretion that limits intestinal absorption.191 Recent experiments have demonstrated that kaempferol, quercetin and resveratrol act as reversible competitive inhibitors of recombinant SULTs (SULT1A1, 1A3, 1B1, 1E1 and 2A1).192 The effects of low doses of quercetin on UGT1 and GST were analyzed in different cell lines (Caco-2, HT-29, HuTu 80 and LT97) and revealed an induction effect.193 Rosmarinic acid has a low inhibition constant (Ki) of 48.74 nM for GST when analyzed in vitro, suggesting a high capacity for inhibition.194 Although phase I and II metabolism have been considered a barrier that inhibits absorption, favors excretion and decreases bioactivity, it has been recently proposed that in some cases covalent modifications can increase oral absorption and bioactivity.195Fig. 3 represents the effects that PCs exert on phase I and II metabolism.
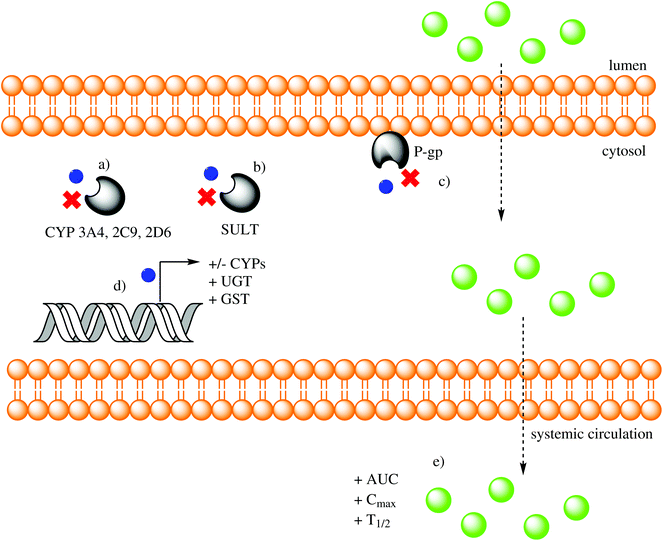 |
| Fig. 3 Effect of phenolic compounds (PCs) on phase I/II enzymes and other xenobiotics. PCs (blue spheres) can inhibit (a) phase I enzymes (members of the cytochrome P450 superfamily, CYP), (b) phase II enzymes (sulfotransferases, SULT) or (c) efflux enzymes (P-gp); (d) they can also upregulate (+) or downregulate (−) mRNA transcription of phase I (CYP) or II (UDP-glucuronosyltransferase, UGT; glutathione-S-transferase, GST) enzymes; (e) net effect of phase I/II modulation is an increase (+) in area under the curve (AUC), maximum plasma concentration (Cmax) and half-life (t1/2) of other orally-ingested xenobiotics (green spheres). | |
4 Pharmacokinetics of phenolic compounds
As with any xenobiotic, orally ingested PCs are subjected to absorption, distribution, metabolism and excretion (ADME), which indicates that their pharmacokinetic profiles can be obtained.196 Some parameters that have been utilized to evaluate their pharmacokinetic behavior include maximum concentration (Cmax), time to reach maximum concentration (Tmax), area under the plasma concentration curve (AUC), mean residence time (MRT) and elimination half-life (T1/2). Table 2 summarizes the pharmacokinetic parameters for different PCs in plasma after oral administration.
Table 2 Pharmacokinetic parameters of selected orally ingested PC
PC |
Condition/dose (per kg of body weight) |
C
max
|
T
max (h) |
T
1/2 (h) |
AUC |
Model/reference/units |
ND not detected; NR not reported; ** standard error of the mean; # standard deviation; Cmax maximum concentration; Tmax time maximum concentration; T1/2 elimination half-life; AUC area under the plasma concentration curve; * MRT mean residence time; O-S (O-sulphate); O-G (O-glycoside); O-GN (O-glucuronide); O-R (O-rutinoside). |
Ferulic acid (FA)# |
14.75 mg pure FA per kg |
0.7 ± 0.1 |
0.1 ± 0.0 |
3.81 ± 1.0 |
1.11 ± 0.2 |
Sprague-Dawley rats198 |
FA# |
Rhizoma chuanxiong extract/14.75 mg FA per kg |
0.4 ± 0.1 |
0.3 ± 0.1 |
16.3 ± 4.1 |
0.77 ± 0.1 |
C
max μg L−1 |
FA# |
Nao-De-Sheng decoction/14.75 mg FA per kg |
0.5 ± 0.1 |
0.1 ± 0.0 |
13.4 ± 3.5 |
1.02 ± 0.1 |
AUC0–∞ μg h L−1 |
Puerarin (P)# |
Pure compound/100 mg P per kg |
0.2 ± 0.0 |
0.5 ± 0.3 |
6.0 ± 3.3 |
1.24 ± 0.1 |
|
P# |
Radix puerariae extract/100 mg P per kg |
0.1 ± 0.0 |
0.9 ± 0.1 |
10.8 ± 1.9 |
0.33 ± 0.1 |
|
P# |
Nao-De-Sheng decoction/100 mg P per kg |
0.2 ± 0.1 |
0.9 ± 0.1 |
4.05 ± 1.9 |
1.01 ± 0.1 |
|
|
FA# |
FA pure compound/10 mg FA per kg |
8.1 ± 1.0 |
0.03 ± 0.00 |
1.7 ± 0.7 |
2961.7 ± 166.5 |
Sprague-Dawley rats197 |
FA# |
FA with Honghua/10 mg FA per kg + 700 mg Honghua per kg |
9.7 ± 1.9 |
0.09 ± 0.05 |
1.0 ± 0.6 |
4794.9 ± 663.2 |
C
max μg ml−1 |
FA# |
FA with clopidogrel/10 mg FA per kg + 7 mg clopidogrel per kg |
1.4 ± 1.53 |
0.1 ± 0.1 |
0.8 ± 0.5 |
4843.6 ± 1121.8 |
AUC0–∞ h ng per mL |
|
Quercetin (Q)** |
Beverage (500 mg Q + 26.9 g Tang®)/500 mg |
354.4 ± 87.6 |
4.7 ± 0.3 |
8.3 ± 1.4 |
3845.9 ± 689.8 |
Men and women227 |
Q** |
Bar (Q fortified First Strike™ bar)/500 mg |
698.1 ± 189.5 |
2.3 ± 0.5 |
8.0 ± 1.3 |
5314.8 ± 1432.4 |
C
max μg L−1 |
Q** |
Chews (Q in 2 chews)/500 mg |
1051.9 ± 393.1 |
3.3 ± 0.8 |
5.5 ± 0.9 |
4147.1 ± 671.8 |
AUC total μg L−1 h−1 |
|
Q# |
2.5 mg pure Q per kg with R |
165.3 ± 31.9 |
3.37 ± 0.5 |
7.7 ± 1.1* |
972.1 ± 85.8 |
Wistar rats200 |
Q# |
2.5 mg Q per kg from polyherbal formulation |
90.8 ± 21.4 |
3.27 ± 0.9 |
12.2 ± 1.5* |
406.6 ± 24.8 |
C
max ng mL−1 |
Rutin (R)# |
2.5 mg pure R per kg with Q |
61.1 ± 29.3 |
4.17 ± 1.2 |
11.9 ± 1.5* |
456.6 ± 29.8 |
AUC0–∞ ng mL−1 h−1 |
R# |
2.5 mg R per kg from polyherbal formulation |
121.7 ± 19.2 |
4.43 ± 0.8 |
7.4 ± 1.1* |
731.4 ± 31.1 |
|
|
Naringenin (NG)** |
7 g raw tomatoes per kg (0.50 ± 0.03 μg NG per g) |
ND |
ND |
ND |
ND |
Men and women208 |
NG** |
3.5 g cooked tomato sauce per kg (3.39 ± 0.1 μg NG per g) |
11.9 ± 2.7 |
0.3 ± 0.1 |
1.0 ± 0.2* |
979 ± 360 |
C
max nmol L−1 |
NG** |
3.5 g tomato sauce + olive oil cooked/kg (3.82 ± 0.22 μg NG per g) |
12.8 ± 3.1 |
0.7 ± 0.1 |
0.9 ± 0.2* |
1459 ± 159 |
AUC nmol L−1 min−1 |
NG glucuronide** |
7 g raw tomatoes per kg |
17.4 ± 3.8 |
2.1 ± 1.0 |
3.6 ± 0.3* |
5330 ± 1105 |
|
NG glucuronide** |
3.5 g cooked tomato sauce per kg |
427.9 ± 59.0 |
0.6 ± 0.1 |
1.7 ± 0.1* |
49.7 ± 7.8 |
|
NG glucuronide** |
3.5 g cooked tomato sauce + olive oil/kg |
353.1 ± 55.3 |
0.9 ± 0.1 |
2.4 ± 0.3* |
58.6 ± 12.1 |
|
Caffeic acid (CA) glucuronide** |
Raw tomato/7 g raw tomatoes per kg |
6.2 ± 1.5 |
1.6 ± 0.7 |
2.6 ± 0.4* |
1141 ± 56 |
|
CA glucuronide** |
3.5 g cooked tomato sauce per kg |
5.0 ± 2.7 |
2.5 ± 0.5 |
2.7 ± 0.8* |
835 ± 138 |
|
CA glucuronide** |
3.5 g cooked tomato sauce + olive oil/kg |
4.1 ± 0.5 |
1.5 ± 0.5 |
2.3 ± 0.0* |
689 ± 122.8 |
|
FA glucuronide** |
Raw tomato/7 g raw tomatoes per kg |
389.0 ± 82.6 |
3.0 ± 1.1 |
4.6 ± 0.8* |
134.6 ± 32.1 |
|
FA glucuronide** |
3.5 g cooked tomato sauce per kg |
343.9 ± 77.6 |
3.0 ± 1.1 |
7.5 ± 1.4* |
184.1 ± 34.3 |
|
FA glucuronide** |
3.5 g cooked tomato sauce + olive oil/kg |
351.5 ± 72.0 |
6.4 ± 3.7 |
5.8 ± 1.7* |
139.1 ± 46.8 |
|
|
Cichoric acid (CiA)# |
E. purpurea extract/10 g per kg extract (2.4 mg CiA per g) |
1263.6 ± 114.5 |
0.2 ± 0.0 |
8.4 ± 0.3 |
2426.5 ± 178.8 |
Sprague-Dawley rats228 |
Chlorogenic acid (ChlA)# |
E. purpurea extract/10 g per kg extract (0.99 mg ChlA per g) |
307.9 ± 24.5 |
0.2 ± 0.0 |
7.7 ± 1.4 |
692.5 ± 140.2 |
C
max ng mL−1 |
Quinic acid (QnA)# |
E. purpurea extract/10 g per kg extract (0.12 mg QnA per g) |
612.7 ± 48.3 |
1.4 ± 0.1 |
6.6 ± 1.6 |
6723.6 ± 485.2 |
AUC0–∞ ng h per mL |
CA# |
E. purpurea extract/10 g per kg extract (0.58 mg CA per g) |
558.4 ± 25.0 |
6.0 ± 0.0 |
4.9 ± 1.6 |
3976.1 ± 266.6 |
|
|
Mangiferin (Mn)# |
Pure compound/270 mg Mn per kg |
0.4 |
0.8 |
14.1 |
5.4 |
Wistar rats199 |
Mn# |
Zhi mu tablet (Anemarrhenae rhizoma)/270 mg Mn per kg |
1.3 ± 0.4 |
5.0 ± 0.6 |
4.9 ± 2.6 |
11.3 ± 4.1 |
C
max μg ml−1 |
|
|
|
|
|
|
AUC0–∞ μg h L−1 |
|
Delphinidin-3-O-G** |
350 mL of 100% grape juice (58 ± 1 μmol) |
1.4 ± 0.3 |
1.4 ± 0.4 |
0.9 |
3.5 ± 0.5 |
Men and women229 |
Delphinidin-O-GN** |
|
1.5 ± 0.4 |
3.3 ± 0.3 |
1 |
6.9 ± 2.2 |
C
max nmol L−1 |
Petunidin-3-O-G** |
350 ml (6.8 ± 0.1 μmol) |
1.0 ± 0.5 |
1.3 ± 0.5 |
NR |
2.0 ± 0.6 |
AUC nmol h L−1 |
Petunidin-O-GN** |
|
2.0 ± 0.5 |
2.6 ± 0.2 |
1.4 |
7.6 ± 2.9 |
|
p-Coumaric acid** |
350 ml (1.9 ± 0.1 μmol) |
64 ± 14 |
0.7 ± 0.1 |
NR |
88 ± 27 |
|
m-Dihydrocoumaric acid** |
|
355 ± 57 |
5.8 ± 0.5 |
NR |
4080 ± 968 |
|
Dihydrocoumaric acid-O-S** |
|
27 ± 1 |
6.0 ± 2.0 |
NR |
388 ± 92 |
|
CA** |
350 ml (0.7 ± 0.1 μmol) |
178 ± 53 |
0.5 ± 0.2 |
NR |
1306 ± 312 |
|
CA-3′-O-S** |
|
47 ± 11 |
1.0 ± 0.0 |
NR |
90 ± 23 |
|
Dihydrocaffeic acid-3′-O-S** |
|
161 ± 37 |
3.9 ± 0.4 |
NR |
1164 ± 368 |
|
Dihydroferulic acid-4′-O-S** |
|
42 ± 9 |
4.4 ± 0.7 |
NR |
359 ± 164 |
|
FA** |
350 ml (0.4 ± 0.0 μmol) |
63 ± 13 |
1.8 ± 0.9 |
NR |
468 ± 215 |
|
FA-4′-O-S** |
|
63 ± 9 |
1.2 ± 0.2 |
NR |
370 ± 115 |
|
|
Onion supplement# |
Onion stewed and homogenized/100 mg |
2.3 ± 1.4 |
0.6 ± 0.2 |
10.9 ± 4.1 |
9.7 ± 6.9 |
Men and women230 |
Q-4′-O-G# |
Isolated from onions/100 mg |
2.1 ± 1.6 |
0.7 ± 0.3 |
11.9 ± 4.0 |
8.4 ± 9.1 |
C
max μg mL−1 |
Buckwheat tea# |
Buckwheat tea/200 mg |
0.6 ± 0.6 |
4.3 ± 1.8 |
10.3 ± 3.5 |
3.8 ± 3.9 |
AUC0–24h h μg mL−1 |
Q-3-O-R# |
Q-3-O-R (tablet)/200 mg |
0.3 ± 0.3 |
6.9 ± 2.9 |
11.8 ± 3.1 |
2.5 ± 2.2 |
|
|
Q aglycone** |
Q aglycone + 3% fat/30 μmol Q per kg |
0.51 ± 0.05 |
1.7 ± 0.1 |
NR |
117.3 ± 18.5 |
Pigs32 |
Q aglycone** |
Q aglycone + 17% fat/30 μmol Q per kg |
0.58 ± 0.06 |
1.1 ± 0.1 |
NR |
184.5 ± 19.8 |
C
max μmol L−1 |
Q aglycone** |
Q aglycone + 32% fat/30 μmol Q per kg |
0.56 ± 0.05 |
0.8 ± 0.1 |
NR |
176.0 ± 18.5 |
AUC0–24h min μmol L−1 |
Q-3-O-G** |
Q-3-O-G + 3% fat/30 μmol Q per kg |
0.90 ± 0.08 |
1.1 ± 0.1 |
NR |
205.5 ± 19.8 |
|
Q-3-O-G** |
Q-3-O-G + 17% fat/30 μmol Q per kg |
0.89 ± 0.08 |
0.8 ± 0.1 |
NR |
270.9 ± 19.8 |
|
Q-3-O-G** |
Q-3-O-G + 32% fat/30 μmol Q per kg |
0.64 ± 0.08 |
0.7 ± 0.1 |
NR |
249.7 ± 19.8 |
|
Pharmacokinetic parameters vary for each compound and depend on different factors such as the molecular structure, dose, food matrix, localization in food, and study model, among others. For example, while the Tmax of pure ferulic acid is 0.03–0.18 h, the Tmaxes of puerarin and mangiferin are 0.55 and 0.83 h, respectively.197–199 The oral co-administration of quercetin and rutin flavonoids, either pure or from herb extracts (Hippophae rhamnoides), has revealed differences in the Cmax, Tmax, T1/2 and AUC in plasma.200 The Cmax and AUC of quercetin were higher than rutin when ingested together as pure compounds, which shows that quercetin affects the absorption of rutin. However, when both of the compounds naturally found in herb extracts were co-administered, the Cmax and AUC of rutin were higher than quercetin.200 In another study, puerarin was administered together with catechin, and the AUC and Cmax for puerarin were 2.48- and 3.91-fold higher than when puerarin was administered alone. The opposite behavior was observed for catechin, which had lower AUC and Cmax values than catechin administered alone. This behavior could be explained by the competitive efflux of catechin and puerarin by P-gp and MRP2 in the small intestine.201
Several studies have been developed using common Chinese herbs combined with Western drugs. Hongua and clopidogrel are often combined with ferulic acid-containing herbs to aid in cardiovascular disease treatment. Li et al. found that the Cmax and AUC of plasma ferulic acid were significantly increased in rats when it was co-administered with clopidogrel (74.3 and 79.7%, respectively) compared with pure ferulic acid. Likewise, the average Tmax was reduced 3 and 3.76 times when ferulic acid was co-administered with Honghua and clopidogrel, respectively. This behavior was the opposite of what was observed with pure ferulic acid administration and was associated with local interactions of ferulic acid with Honghua and clopidogrel in the intestine and/or an alteration in phase I/II metabolism (as previously discussed).197 Ouyang et al. observed that the AUC, Tmax and Cmax of pure ferulic acid and ferulic acid from a Nao-De-Sheng decoction were higher than ferulic acid from the aqueous extract of Rhizoma chuanxiong. Similar behavior was observed when pure puerarin was orally administered, and the AUC, Tmax and Cmax were higher than when Radix puerariae extract was used as a source of puerarin.198
Another study compared the pharmacokinetics of isorhamnetin, quercetin and kaempferol after the oral administration of three different Hippophae rhamnoides preparations (TFH) in beagle dogs. The results showed that the Cmax and AUC of isorhamnetin and quercetin in TFH-solid dispersion (TFH-SD) and TFH-self-emulsifying (TFH-SE) preparations were significantly enhanced compared to the TFH preparations, which represents a new alternative for the oral administration of flavones from TFH. Additionally, a double-peak phenomenon was observed with isorhamnetin (three TFH preparations), while this was only observed with quercetin in the TFH-SD preparations. This behavior revealed that quercetin and isorhamnetin have two sites of absorption, the stomach and gut. These PCs also exhibited different behaviors in different TFH preparations.202 These results indicate that the source of PC, the interactions between them and their interactions with other molecules in the matrix alter their pharmacokinetic parameters.
All of the previously discussed interactions of PCs with fiber and other nutrients will be reflected in the pharmacokinetic behavior of PCs. In cereals such as corn, sorghum and wheat, the majority of PCs (>75%) are bound to indigestible cell wall polysaccharides.203 The linkage of ferulic acid to xylans from wheat results in the low bioaccessibility of this compound in the small intestine (0.5%).204 Additionally, the presence of hydroxyl groups on PCs favors hydrogen bond formation between PCs and cellulose or xylans.205 The consequent decrease in absorption is reflected by a decrease in pharmacokinetic values. However, other studies have shown that PC–fiber interactions in fruits such as mango, papaya or pineapple have no impact on the bioaccessibility or antioxidant capacity of PCs.206 PC–protein and PC–lipid interactions show that the pharmacokinetic behaviors of PCs can be affected.207,208 Rosmarinic acid can form complexes with whey proteins in bovine milk (α-lactalbumin, β-lactoglobulin and lactoferrin) by hydrophobic, hydrogen bonding and dipole–dipole non-covalent interactions, and these complexes are more stable than proteins alone in in vitro digestion models.207
The pharmacokinetic behavior of PCs can also be altered by other compounds that may be generated as a result of their metabolism (phase I/II and/or bacterial).209 For example, caffeic acid can be metabolized to dihydrocaffeic acid (reductase), caffeic acid-3-O-sulfate (SULT), ferulic acid or isoferulic acid (catechol-O-methyltransferase), which can result in an overestimation of caffeic acid levels and disturb its pharmacokinetics.209,210 Thus, pharmacokinetic profiles are a result of all of the previous GI interactions and metabolism.
5 Nanotechnology to improve the bioefficacy of phenolic compounds
Different techniques are currently being developed to minimize or bypass the previously described PC interactions in favor of chemical protection and targeted delivery. Nanotechnology is a novel and promising way to manipulate several metabolic processes to prevent or treat specific health-related problems.211 Encapsulation allows for the controlled released of PCs and can be achieved at a nanoscale level, such as with liposomes and polymeric nanoparticles.212 Also, loading biocompatible and biodegradable nanoparticles with PCs can enhance their aqueous solubility, chemical stability, bioavailability, circulation time and target specificity.213 In that sense, nanoparticles have been proposed as carriers for PCs to improve their bioavailability and bioefficacy.
Haratifar et al.214 used casein micelles as encapsulating material to protect tea EGCG from oxidation at the intestinal level. They found that EGCG encapsulation with casein micelles did not diminish its bioefficacy on proliferation inhibition in a cancerous colonic epithelial cell line (D/v-src), whereas the proliferation of the normal cell line (4D/WT) was maintained. A similar study performed by Guri et al.215 reported that a PC–casein complex had similar effects as free PCs and did not decrease the viability of HT29-MTX, which mimics the presence of a mucus barrier in the intestine, whereas it did decrease the cell viability of adenocarcinoma cells (HT-29) proportional to the EGCG concentration. However, Jain et al.216 have reported that the co-encapsulation of tamoxifen and quercetin in poly-(lactic-co-glycolic acid) nanoparticles results in a 5- and 3-fold increase in the oral bioavailability of tamoxifen and quercetin, respectively.
The use of nanosystems could facilitate the passage of PCs or other bioactive compounds through biological barriers and protect them from the metabolic modifications that result in lower absorption rates.217 The most common biocompatible and biodegradable nanoparticles include nanoliposomes, nanoemulsions, lipid nanocarriers, micelles and poly-(lactic-co-glycolic acid) nanoparticles.211
Nanoliposomes, or nanometric liposomes, are colloidal structures that are commonly composed of phospholipids. These nanomaterials have been shown to enhance the bioefficacy of bioactive compounds. Due to their natural composition, they should be able to overcome regulatory hurdles to be readily attainable, and newly developed food products or nutraceutical formulations could be quickly implemented. Zou et al.218 studied the stability of PCs from tea in nanoliposomes and reported that relatively favorable sustained release properties could be achieved with only 29.8% of the released PCs after 24 h of incubation. However, the use of nanoliposomes improved the chemical stability of PCs under alkaline pH conditions. These results suggest that further studies must be performed to enhance the use of nanoliposomes in food products with long shelf lives and improve bioefficacy.
Nanoemulsions consist of 10–100 nm oil droplets dispersed within an aqueous continuous phase, with each oil droplet surrounded by surfactant molecules.219 Either micro- or nanoemulsions have been commonly developed to encapsulate lipophilic components so they can be dispersed into an aqueous medium. However, it is also possible to prepare water-in-oil micro- and nanoemulsions.220 In that sense, nanoemulsions can also be successfully applied to PC entrapment. Baccarin et al.221 showed that a PC-rich ethyl acetate fraction from pomegranate seeds entrapped in oil nanoemulsions was internalized as nanodroplets by the cells, but a higher proportion was detected along the cell membrane, which results in protection against UVB-induced DNA damage in the keratinocyte HaCaT cell line. Recently, a study performed by Nasr222 focused on developing a mucoadhesive lipid nanoemulsion based on hyaluronic acid and co-encapsulated resveratrol and curcumin for the transnasal treatment of neurodegenerative diseases. This nanoemulsion was safe for use on the nasal mucosa, and it improved the brain delivery of PCs with a 7- and 9-fold increase for resveratrol and curcumin, respectively. Likewise, Rocha et al.223 reported that polysaccharide nanoparticles can be successfully applied to enhance the chemical stability of EGCG while retaining its bioactivity, thereby reducing cell viability and inducing apoptosis in Du145 prostate cancer cells.
Nanotechnology can be applied to enhance the bioefficacy of certain types of PCs and combinations of them. Singh et al.224 studied the antiproliferative effects of EGCG and theaflavin nanoparticles on diverse human cancer cell lines such as A549 (lung carcinoma), HeLa (cervical carcinoma) and THP-1 (acute monocytic leukemia) cells. They reported that EGCG and theaflavin enhanced the potential benefits of cisplatin. However, the nanoparticles containing EGCG-theaflavin and cisplatin were 20-fold more effective than the free compounds.
By using liposomes or other nanoparticles, nanotechnology can increase the bioavailability and cellular uptake of these compounds.225 Hence, nanoparticle material should be selected based on the desired effect or the specific tissue to which PC delivery is required, and the chemical stability of both the nanoparticles and PCs along the GI tract should be taken into consideration.
Conclusion
Phenolic compounds interact with several macro- and micronutrients and host proteins (digestive enzymes, nutrient transporters and xenobiotic-metabolizing enzymes) within the gastrointestinal tract, and these interactions result in delayed or decreased nutrient digestion and absorption. Their absorption and pharmacokinetic parameters depend on their size, molecular complexity, charge, the food matrix, and the presence of other phenolic compounds or drugs. They can inhibit phase I and II enzymes and regulate their expression, which can lead to potential drug–drug or drug–nutrient interactions. Knowledge of all of the mentioned interactions has inspired novel nanotechnological approaches that are currently under development to avoid undesired interactions, protect PCs from harsh chemical environments and allow for targeted delivery. The study of the specific molecules that are able to transport and protect these compounds is necessary to complement and provide an alternative to the therapies that are commonly used to treat different types of cancers and vascular disorders. The mechanism and mode of action of most PCs require further study due to their complex structures that affect their molecular interactions with other biomolecules, minerals, vitamins and enzymes.
Acknowledgements
The authors declare no competing financial interests. This manuscript was edited for English language by American Journal Experts (AJE). Financial support from the National Council of Science and Technology (Conacyt) is acknowledged. This work is part of the project 563 Fronteras de la Ciencia of Conacyt “Un enfoque multidisciplinario de la farmacocinética de polifenoles de mango Ataulfo. Interacciones moleculares, estudios preclínicos y clínicos”.
References
- V. Cheynier, Polyphenols in foods are more complex than often thought, Am. J. Clin. Nutr., 2005, 81, 223s–229s CAS
.
- R. K. Lall, D. N. Syed, V. M. Adhami, M. I. Khan and H. Mukhtar, Dietary Polyphenols in Prevention and Treatment of Prostate Cancer, Int. J. Mol. Sci., 2015, 16, 3350–3376 CrossRef CAS PubMed
.
- G. M. Pasinetti, J. Wang, L. Ho, W. Zhao and L. Dubner, Roles of resveratrol and other grape-derived polyphenols in Alzheimer's disease prevention and treatment, Biochim. Biophys. Acta, Mol. Basis Dis., 2015, 1852, 1202–1208 CrossRef CAS PubMed
.
- A. K. Ristic, P. A. Kroon and M. Glibetic, Modulation of platelet function with dietary polyphenols A promising strategy in the prevention of cardiovascular disease, Agro Food Ind. Hi Tech, 2015, 26, 15–18 CAS
.
- C. Spagnuolo, M. Russo, S. Bilotto, I. Tedesco, B. Laratta and G. L. Russo, Dietary polyphenols in cancer prevention: the example of the flavonoid quercetin in leukemia, Ann. N. Y. Acad. Sci., 2012, 1259, 95–103 CrossRef CAS PubMed
.
- S. Arranz, J. M. Silvan and F. Saura-Calixto, Nonextractable polyphenols, usually ignored, are the major part of dietary polyphenols: A study on the Spanish diet, Mol. Nutr. Food Res., 2010, 54, 1646–1658 CAS
.
- J. M. Hernandez-Hierro, N. Quijada-Morin, L. Martinez-Lapuente, Z. Guadalupe, B. Ayestaran, J. C. Rivas-Gonzalo and M. T. Escribano-Bailon, Relationship between skin cell wall composition and anthocyanin extractability of Vitis vinifera L. cv. Tempranillo at different grape ripeness degree, Food Chem., 2014, 146, 41–47 CrossRef CAS PubMed
.
- C. Le Bourvellec, B. Bouchet and C. M. G. C. Renard, Non-covalent interaction between procyanidins and apple cell wall material. Part III: Study on model polysaccharides, Biochim. Biophys. Acta, Gen. Subj., 2005, 1725, 10–18 CrossRef CAS PubMed
.
- M. Pinelo, A. Arnous and A. S. Meyer, Upgrading of grape skins: Significance of plant cell-wall structural components and extraction techniques for phenol release, Trends Food Sci. Technol., 2006, 17, 579–590 CrossRef CAS
.
- Y. X. Wang, J. Liu, F. Chen and G. H. Zhao, Effects of Molecular Structure of Polyphenols on Their Noncovalent Interactions with Oat beta-glucan, J. Agric. Food Chem., 2013, 61, 4533–4538 CrossRef CAS PubMed
.
- A. D. T. Phan, G. Netzel, D. J. Wang, B. M. Flanagan, B. R. D'Arcy and M. J. Gidley, Binding of dietary polyphenols to cellulose: Structural and nutritional aspects, Food Chem., 2015, 171, 388–396 CrossRef CAS PubMed
.
- D. F. Chen, J. L. Shi and X. Z. Hu, Enhancement of polyphenol content and antioxidant capacity of oat (Avena nuda L.) bran by cellulase treatment, Appl. Biol. Chem., 2016, 59, 397–403 CrossRef CAS
.
- J. Pekkinen, N. N. Rosa, O. I. Savolainen, P. Keski-Rahkonen, H. Mykkanen, K. Poutanen, V. Micard and K. Hanhineva, Disintegration of wheat aleurone structure has an impact on the bioavailability of phenolic compounds and other phytochemicals as evidenced by altered urinary metabolite profile of diet-induced obese mice, Nutr. Metab., 2014, 11, 1 Search PubMed
.
- N. M. Anson, A. M. Aura, E. Selinheimo, I. Mattila, K. Poutanen, R. van den Berg, R. Havenaar, A. Bast and G. R. M. M. Haenen, Bioprocessing of Wheat Bran in Whole Wheat Bread Increases the Bioavailability of Phenolic Acids in Men and Exerts Antiinflammatory Effects ex Vivo, J. Nutr., 2011, 141, 137–143 CrossRef CAS PubMed
.
- F. Saura-Calixto, Dietary Fiber as a Carrier of Dietary Antioxidants: An Essential Physiological Function, J. Agric. Food Chem., 2011, 59, 43–49 CrossRef CAS PubMed
.
- M. Duenas, I. Munoz-Gonzalez, C. Cueva, A. Jimenez-Giron, F. Sanchez-Patan, C. Santos-Buelga, M. V. Moreno-Arribas and B. Bartolome, A survey of modulation of gut microbiota by dietary polyphenols, BioMed Res. Int., 2015, 2015, 850902 Search PubMed
.
- A. Duda-Chodak, T. Tarko, P. Satora and P. Sroka, Interaction of dietary compounds, especially polyphenols, with the intestinal microbiota: a review, Eur. J. Nutr., 2015, 54, 325–341 CrossRef CAS PubMed
.
- R. Cohen, B. Schwartz, I. Peri and E. Shimoni, Improving Bioavailability and Stability of Genistein by Complexation with High-Amylose Corn Starch, J. Agric. Food Chem., 2011, 59, 7932–7938 CrossRef CAS PubMed
.
- L. M. Zhang, X. Yang, S. Li and W. Y. Gao, Preparation, physicochemical characterization and in vitro digestibility on solid complex of maize starches with quercetin, LWT–Food Sci. Technol., 2011, 44, 787–792 CrossRef CAS
.
- Y. W. Chai, M. Z. Wang and G. Y. Zhang, Interaction between Amylose and Tea Polyphenols Modulates the Postprandial Glycemic Response to High-Amylose Maize Starch, J. Agric. Food Chem., 2013, 61, 8608–8615 CrossRef CAS PubMed
.
- D. B. Amoako and J. M. Awika, Polymeric tannins significantly alter properties and in vitro digestibility of partially gelatinized intact starch granule, Food Chem., 2016, 208, 10–17 CrossRef CAS PubMed
.
- L. Deladino, A. S. Teixeira, A. S. Navarro, I. Alvarez, A. D. Molina-Garcia and M. Martino, Corn starch systems as carriers for yerba mate (Ilex paraguariensis) antioxidants, Food Bioprod. Process., 2015, 94, 463–472 CrossRef CAS
.
- H. N. Englyst and G. J. Hudson, The classification and measurement of dietary carbohydrates, Food Chem., 1996, 57, 15–21 CrossRef CAS
.
- G. A. Camelo-Mendez, E. Agama-Acevedo, M. M. Sanchez-Rivera and L. A. Bello-Perez, Effect on in vitro starch digestibility of Mexican blue maize anthocyanins, Food Chem., 2016, 211, 281–284 CrossRef CAS PubMed
.
- F. Barros, J. M. Awika and L. W. Rooney, Interaction of Tannins and Other Sorghum Phenolic Compounds with Starch and Effects on in Vitro Starch Digestibility, J. Agric. Food Chem., 2012, 60, 11609–11617 CrossRef CAS PubMed
.
- Y. Wu, Q. L. Lin, Z. X. Chen and H. X. Xiao, The interaction between tea polyphenols and rice starch during gelatinization, Food Sci. Technol. Int., 2011, 17, 569–577 CrossRef CAS PubMed
.
- R. Quek and C. J. Henry, Influence of polyphenols from lingonberry, cranberry, and red grape on in vitro digestibility of rice, Int. J. Food Sci. Nutr., 2015, 66, 378–382 CrossRef CAS PubMed
.
- D. D. Ramdath, E. Padhi, A. Hawke, T. Sivaramalingam and R. Tsao, The glycemic index of pigmented potatoes is related to their polyphenol content, Food Funct., 2014, 5, 909–915 CAS
.
- L. Zhang, H. T. Li, L. Shen, Q. C. Fang, L. L. Qian and W. P. Jia, Effect of Dietary Resistant Starch on Prevention and Treatment of Obesity-related Diseases and Its Possible Mechanisms, Biomed. Environ. Sci., 2015, 28, 291–297 Search PubMed
.
- L. A. Bello-Pérez and O. Paredes-López, Starches of Some Food Crops, Changes During Processing and Their Nutraceutical Potential, Food Eng. Rev., 2009, 1, 50–65 CrossRef
.
- K. Azuma, K. Ippoushi, H. Ito, H. Higashio and J. Terao, Combination of lipids and emulsifiers enhances the absorption of orally administered quercetin in rats, J. Agric. Food Chem., 2002, 50, 1706–1712 CrossRef CAS PubMed
.
- S. Lesser, R. Cermak and S. Wolffram, Bioavailability of quercetin in pigs is influenced by the dietary fat content, J. Nutr., 2004, 134, 1508–1511 CAS
.
- Y. Guo, E. Mah, C. G. Davis, T. Jalili, M. G. Ferruzzi, O. K. Chun and R. S. Bruno, Dietary fat increases quercetin bioavailability in overweight adults, Mol. Nutr. Food Res., 2013, 57, 896–905 CAS
.
- D. M. Ribnicky, D. E. Roopchand, A. Oren, M. Grace, A. Poulev, M. A. Lila, R. Havenaar and I. Raskin, Effects of a high fat meal matrix and protein complexation on the bioaccessibility of blueberry anthocyanins using the TNO gastrointestinal model (TIM-1), Food Chem., 2014, 142, 349–357 CrossRef CAS PubMed
.
- N. Ortega, J. Reguant, M. P. Romero, A. Macia and M. J. Motilva, Effect of Fat Content on the Digestibility and Bioaccessibility of Cocoa Polyphenol by an in Vitro Digestion Model, J. Agric. Food Chem., 2009, 57, 5743–5749 CrossRef CAS PubMed
.
- R. R. Bansode, P. Randolph, M. Ahmedna, S. Hurley, T. Hanner, S. A. S. Baxter, T. A. Johnston, M. M. Su, B. M. Holmes, J. M. Yu and L. L. Williams, Bioavailability of polyphenols from peanut skin extract associated with plasma lipid lowering function, Food Chem., 2014, 148, 24–29 CrossRef CAS PubMed
.
- A. de Athayde Moncorvo Collado, F. G. Dupuy, R. D. Morero and C. Minahk, Cholesterol induces surface localization of polyphenols in model membranes thus enhancing vesicle stability against lysozyme, but reduces protection of distant double bonds from reactive-oxygen species, Biochim. Biophys. Acta, Biomembr., 2016, 1858, 1479–1487 CrossRef CAS PubMed
.
- M. A. Vermeer, T. P. J. Mulder and H. O. F. Molhuizen, Theaflavins from Black Tea, Especially Theaflavin-3-gallate, Reduce the Incorporation of Cholesterol into Mixed Micelles, J. Agric. Food Chem., 2008, 56, 12031–12036 CrossRef CAS PubMed
.
- I. Ikeda, T. Yamahira, M. Kato and A. Ishikawa, Black-Tea Polyphenols Decrease Micellar Solubility of Cholesterol in Vitro and Intestinal Absorption of Cholesterol in Rats, J. Agric. Food Chem., 2010, 58, 8591–8595 CrossRef CAS PubMed
.
- Y. Ogino, K. Osada, S. Nakamura, Y. Ohta, T. Kanda and M. Sugano, Absorption of dietary cholesterol oxidation products and their downstream metabolic effects are reduced by dietary apple polyphenols, Lipids, 2007, 42, 151–161 CrossRef CAS PubMed
.
- M. Nekohashi, M. Ogawa, T. Ogihara, K. Nakazawa, H. Kato, T. Misaka, K. Abe and S. Kobayashi, Luteolin and Quercetin Affect the Cholesterol Absorption Mediated by Epithelial Cholesterol Transporter Niemann-Pick C1-Like 1 in Caco-2 Cells and Rats, PLoS One, 2014, 9(5), e97901 Search PubMed
.
- K. Ogawa, S. Hirose, S. Nagaoka and E. Yanase, Interaction between Tea Polyphenols and Bile Acid Inhibits Micellar Cholesterol Solubility, J. Agric. Food Chem., 2016, 64, 204–209 CrossRef CAS PubMed
.
- T. Tamura, N. Inoue, M. Ozawa, A. Shimizu-Ibuka, S. Arai, N. Abe, H. Koshino and K. Mura, Peanut-Skin Polyphenols, Procyanidin A1 and Epicatechin-(4 beta -> 6)-epicatechin-(2 beta -> O -> 7, 4 beta -> 8)-catechin, Exert Cholesterol Micelle-Degrading Activity in Vitro, Biosci., Biotechnol., Biochem., 2013, 77, 1306–1309 CrossRef CAS PubMed
.
- Z. Wu, J. Teng, L. Huang, N. Xia and B. Wei, Stability, antioxidant activity and in vitro bile acid-binding of green, black and dark tea polyphenols during simulated in vitro gastrointestinal digestion, RSC Adv., 2015, 5, 92089–92095 RSC
.
- J. A. Dominguez-Avila, E. Alvarez-Parrilla, J. A. Lopez-Diaz, I. E. Maldonado-Mendoza, M. D. Gomez-Garcia and L. A. de la Rosa, The pecan nut (Carya illinoinensis) and its oil and polyphenolic fractions differentially modulate lipid metabolism and the antioxidant enzyme activities in rats fed high-fat diets, Food Chem., 2015, 168, 529–537 CrossRef CAS PubMed
.
- B. T. Metzger, D. M. Barnes and J. D. Reed, A Comparison of Pectin, Polyphenols, and Phytosterols, Alone or in Combination, to Lovastatin for Reduction of Serum Lipids in Familial Hypercholesterolemic Swine, J. Med. Food, 2009, 12, 854–860 CrossRef CAS PubMed
.
- K. Brudzynski and L. Maldonado-Alvarez, Polyphenol-Protein Complexes and Their Consequences for the Redox Activity, Structure and Function of Honey. A Current View and New Hypothesis - a Review, Pol. J. Food Nutr. Sci., 2015, 65, 71–80 CAS
.
- J. C. Boulet, C. Trarieux, J. M. Souquet, M. A. Ducasse, S. Caille, A. Samson, P. Williams, T. Doco and V. Cheynier, Models based on ultraviolet spectroscopy, polyphenols, oligosaccharides and polysaccharides for prediction of wine astringency, Food Chem., 2016, 190, 357–363 CrossRef CAS PubMed
.
- V. de Freitas and N. Mateus, Protein/Polyphenol Interactions: Past and Present Contributions. Mechanisms of Astringency Perception, Curr. Org. Chem., 2012, 16, 724–746 CrossRef CAS
.
- N. Mateus, E. Carvalho, C. Luis and V. de Freitas, Influence of the tannin structure on the disruption effect of carbohydrates on protein-tannin aggregates, Anal. Chim. Acta, 2004, 513, 135–140 CrossRef CAS
.
- P. Bourassa, J. Bariyanga and H. A. Tajmir-Riahi, Binding sites of resveratrol, genistein, and curcumin with milk alpha- and beta-caseins, J. Phys. Chem. B, 2013, 117, 1287–1295 CrossRef CAS PubMed
.
- J. H. Ye, F. Y. Fan, X. Q. Xu and Y. R. Liang, Interactions of black and green tea polyphenols with whole milk, Food Res. Int., 2013, 53, 449–455 CrossRef CAS
.
- K. Nagy, M.-C. Courtet-Compondu, G. Williamson, S. Rezzi, M. Kussmann and A. Rytz, Non-covalent binding of proteins to polyphenols correlates with their amino acid sequence, Food Chem., 2012, 132, 1333–1339 CrossRef CAS
.
- C. D. Kanakis, I. Hasni, P. Bourassa, P. A. Tarantilis, M. G. Polissiou and H. A. Tajmir-Riahi, Milk beta-lactoglobulin complexes with tea polyphenols, Food Chem., 2011, 127, 1046–1055 CrossRef CAS PubMed
.
- A. Guri, S. Haratifar and M. Corredig, Bioefficacy of Tea Catechins Associated with Milk Caseins Tested Using Different In Vitro Digestion Models, Food Dig., 2014, 5, 8–18 CrossRef CAS
.
- M. W. Korir, F. N. Wachira, J. K. Wanyoko, R. M. Ngure and R. Khalid, The fortification of tea with sweeteners and milk and its effect on in vitro antioxidant potential of tea product and glutathione levels in an animal model, Food Chem., 2014, 145, 145–153 CrossRef CAS PubMed
.
- P. Bourassa, R. Cote, S. Hutchandani, G. Samson and H. A. Tajmir-Riahi, The effect of milk alpha-casein on the antioxidant activity of tea polyphenols, J. Photochem. Photobiol., B, 2013, 128, 43–49 CrossRef CAS PubMed
.
- Z. H. Chen, B. Zhou, L. Yang, L. M. Wu and Z. L. Liu, Antioxidant activity of green tea polyphenols against lipid peroxidation initiated by lipid-soluble radicals in micelles, J. Chem. Soc., Perkin Trans. 2, 2001, 1835–1839 RSC
.
- B. Zhou, L.-M. Wu, L. Yang and Z.-L. Liu, Evidence for α-tocopherol regeneration reaction of green tea polyphenols in SDS micelles, Free Radical Biol. Med., 2005, 38, 78–84 CrossRef CAS PubMed
.
- R. Maestre, J. D. Douglass, S. Kodukula, I. Medina and J. Storch, Alterations in the Intestinal Assimilation of Oxidized PUFAs Are Ameliorated by a Polyphenol-Rich Grape Seed Extract in an In Vitro Model and Caco-2 Cells, J. Nutr., 2013, 143, 295–301 CrossRef CAS PubMed
.
- S. Lamothe, N. Azimy, L. Bazinet, C. Couillard and M. Britten, Interaction of green tea polyphenols with dairy matrices in a simulated gastrointestinal environment, Food Funct., 2014, 5, 2621–2631 CAS
.
- H. J. Giroux, G. De Grandpre, P. Fustier, C. P. Champagne, D. St-Gelais, M. Lacroix and M. Britten, Production and characterization of Cheddar-type cheese enriched with green tea extract, Dairy Sci. Technol., 2013, 93, 241–254 CrossRef CAS
.
- D. J. Pimentel-Gonzalez, M. E. Aguilar-Garcia, G. Aguirre-Alvarez, R. Salcedo-Hernandez, J. C. Guevara-Arauza and R. G. Campos-Montiel, The Process and Maturation Stability of Chihuahua Cheese with Antioxidants in Multiple Emulsions, J. Food Process. Preserv., 2015, 39, 1027–1035 CrossRef CAS
.
- H. Y. Choi, C. J. Yang, K. S. Choi and I. Bae, Characteristics of Gouda cheese supplemented with fruit liquors, J. Anim. Sci. Technol., 2015, 57, 15 CrossRef PubMed
.
- R. Branciari, D. Ranucci, M. Trabalza-Marinucci, M. Codini, M. Orru, R. Ortenzi, C. Forte, M. R. Ceccarini and A. Valiani, Evaluation of the antioxidant properties and oxidative stability of Pecorino cheese made from the raw milk of ewes fed Rosmarinus officinalis L. leaves, Int. J. Food Sci. Technol., 2015, 50, 558–565 CrossRef CAS
.
- A. Helal, D. Tagliazucchi, E. Verzelloni and A. Conte, Gastro-pancreatic release of phenolic compounds incorporated in a polyphenols-enriched cheese-curd, Lwt–Food Sci. Technol., 2015, 60, 957–963 CrossRef CAS
.
- A. Rashidinejad, E. J. Birch, D. Sun-Waterhouse and D. W. Everett, Delivery of green tea catechin and epigallocatechin gallate in liposomes incorporated into low-fat hard cheese, Food Chem., 2014, 156, 176–183 CrossRef CAS PubMed
.
- D. F. da Silva, P. T. Matumoto-Pintro, L. Bazinet, C. Couillard and M. Britten, Effect of commercial grape extracts on the cheese-making properties of milk, J. Dairy Sci., 2015, 98, 1552–1562 CrossRef PubMed
.
- L. Miranda, H. Deußer and D. Evers, The impact of in vitro digestion on bioaccessibility of polyphenols from potatoes and sweet potatoes and their influence on iron absorption by human intestinal cells, Food Funct., 2013, 4, 1595–1601 CAS
.
- L. B. Escudero, C. M. Fusari, J. C. Altamirano, A. B. Camargo and R. G. Wuilloud, Stability of Iron-Quercetin Complexes in Synthetic Wine under In Vitro Digestion Conditions, J. Food Sci., 2014, 79, C1933–C1938 CrossRef CAS PubMed
.
- K. Oueslati, D. de La Pomélie, V. Santé-Lhoutellier and P. Gatellier, Impact of the Fenton process in meat digestion as assessed using an in vitro gastro-intestinal model, Food Chem., 2016, 209, 43–49 CrossRef CAS PubMed
.
- M. Brnic, R. Wegmuller, C. Zeder, G. Senti and R. F. Hurrell, Influence of phytase, EDTA, and polyphenols on zinc absorption in adults from porridges fortified with zinc sulfate or zinc oxide, J. Nutr., 2014, 144, 1467–1473 CrossRef CAS PubMed
.
- E. Y. Kim, T. K. Pai and O. Han, Effect of Bioactive Dietary Polyphenols on Zinc Transport across the Intestinal Caco-2 Cell Monolayers, J. Agric. Food Chem., 2011, 59, 3606–3612 CrossRef CAS PubMed
.
- E. Nkhili, M. Loonis, S. Mihai, H. El Hajji and O. Dangles, Reactivity of food phenols with iron and copper ions: binding, dioxygen activation and oxidation mechanisms, Food Funct., 2014, 5, 1186–1202 CAS
.
- A. Fiesel, M. Ehrmann, D. K. Gessner, E. Most and K. Eder, Effects of polyphenol-rich plant products from grape or hop as feed supplements on iron, zinc and copper status in piglets, Arch. Anim. Nutr., 2015, 69, 276–284 CrossRef CAS PubMed
.
- C. Coudray, J. C. Tressol, C. Feillet-Coudray, J. Bellanger, D. Pepin and A. Mazur, Long-term consumption of red wine does not modify intestinal absorption or status of zinc and copper in rats, J. Nutr., 2000, 130, 1309–1313 CAS
.
- W. Xu, R. Shao and J. Xiao, Is There Consistency between the Binding Affinity and Inhibitory Potential of Natural Polyphenols as alpha-amylase Inhibitors?, Crit. Rev. Food Sci. Nutr., 2016, 56, 1630–1639 CrossRef CAS PubMed
.
- J. J. Gao, P. Xu, Y. F. Wang, Y. Q. Wang and D. Hochstetter, Combined Effects of Green Tea Extracts, Green Tea Polyphenols or Epigallocatechin Gallate with Acarbose on Inhibition against alpha-Amylase and alpha-Glucosidase in Vitro, Molecules, 2013, 18, 11614–11623 CrossRef CAS PubMed
.
- M. Miao, B. Jiang, H. Jiang, T. Zhang and X. F. Li, Interaction mechanism between green tea extract and human alpha-amylase for reducing starch digestion, Food Chem., 2015, 186, 20–25 CrossRef CAS PubMed
.
- H. Nyambe-Silavwe, J. A. Villa-Rodriguez, I. Ifie, M. Holmes, E. Aydin, J. M. Jensen and G. Williamson, Inhibition of human alpha-amylase by dietary polyphenols, J. Funct. Foods, 2015, 19, 723–732 CrossRef CAS
.
- G. Oboh, A. O. Ademiluyi, A. J. Akinyemi, T. Henle, J. A. Saliu and U. Schwarzenbolz, Inhibitory effect of polyphenol-rich extracts of jute leaf (Corchorus olitorius) on key enzyme linked to type 2 diabetes (alpha-amylase and alpha-glucosidase) and hypertension (angiotensin I converting) in vitro, J. Funct. Foods, 2012, 4, 450–458 CrossRef CAS
.
- X. P. Yang and F. B. Kong, Effects of tea polyphenols and different teas on pancreatic alpha-amylase activity in vitro, Lwt–Food Sci. Technol., 2016, 66, 232–238 CrossRef CAS
.
- M. Zaklos-Szyda, I. Majewska, M. Redzynia and M. Koziolkiewicz, Antidiabetic Effect of Polyphenolic Extracts from Selected Edible Plants as alpha-Amylase, alpha-Glucosidase and PTP1B Inhibitors, and beta Pancreatic Cells Cytoprotective Agents - A Comparative Study, Curr. Top. Med. Chem., 2015, 15, 2431–2444 CrossRef PubMed
.
- A. O. Ademiluyi, G. Oboh, A. A. Boligon and M. L. Athayde, Effect of fermented soybean condiment supplemented diet on alpha-amylase and alpha-glucosidase activities in Streptozotocin-induced diabetic rats, J. Funct. Foods, 2014, 9, 1–9 CrossRef CAS
.
- V. Beejmohun, M. Peytavy-Izard, C. Mignon, D. Muscente-Paque, X. Deplanque, C. Ripoll and N. Chapal, Acute effect of Ceylon cinnamon extract on postprandial glycemia: alpha-amylase inhibition, starch tolerance test in rats, and randomized crossover clinical trial in healthy volunteers, BMC Complementary Altern. Med., 2014, 14, 351 CrossRef PubMed
.
- S. A. Shodehinde, A. O. Ademiluyi, G. Oboh and A. A. Akindahunsi, Contribution of Musa paradisiaca in the inhibition of alpha-amylase, alpha-glucosidase and Angiotensin-I converting enzyme in streptozotocin induced rats, Life Sci., 2015, 133, 8–14 CrossRef CAS PubMed
.
- S. Chethan, Y. N. Sreerama and N. G. Malleshi, Mode of inhibition of finger millet malt amylases by the millet phenolics, Food Chem., 2008, 111, 187–191 CrossRef CAS
.
- J. B. Xiao, X. L. Ni, G. Y. Kai and X. Q. Chen, A Review on Structure-Activity Relationship of Dietary Polyphenols Inhibiting alpha-Amylase, Crit. Rev. Food Sci. Nutr., 2013, 53, 497–506 CrossRef CAS PubMed
.
- M. Miao, H. Jiang, B. Jiang, Y. G. Li, S. W. Cui and T. Zhang, Structure elucidation of catechins for modulation of starch digestion, Lwt–Food Sci. Technol., 2014, 57, 188–193 CrossRef CAS
.
- L. Sun, F. J. Warren, G. Netzel and M. J. Gidley, 3 or 3′-Galloyl substitution plays an important role in association of catechins and theaflavins with porcine pancreatic α-amylase: The kinetics of inhibition of α-amylase by tea polyphenols, J. Funct. Foods, 2016, 26, 144–156 CrossRef CAS
.
- J. Villa-Rodriguez, A. Kerimi, L. Abranko and G. Williamson, German Chamomile (Matricaria chamomilla) Extract and Its Major Polyphenols Inhibit Intestinal alpha-glycosidases in vitro, FASEB J., 2015, 29, LB323 CrossRef PubMed
.
- G. Williamson, Possible effects of dietary polyphenols on sugar absorption and digestion, Mol. Nutr. Food Res., 2013, 57, 48–57 CAS
.
- U. Wenzel, Flavonoids as drugs at the small intestinal level, Curr. Opin. Pharmacol., 2013, 13, 864–868 CrossRef CAS PubMed
.
- A. S. Boath, D. Stewart and G. J. McDougall, Berry components inhibit alpha-glucosidase in vitro: Synergies between acarbose and polyphenols from black currant and rowanberry, Food Chem., 2012, 135, 929–936 CrossRef CAS PubMed
.
- T. Satoh, M. Igarashi, S. Yamada, N. Takahashi and K. Watanabe, Inhibitory effect of black tea and its combination with acarbose on small intestinal alpha-glucosidase activity, J. Ethnopharmacol., 2015, 161, 147–155 CrossRef CAS PubMed
.
- S. A. Adefegha, G. Oboh, O. S. Omojokun, T. O. Jimoh and S. I. Oyeleye, In vitro antioxidant activities of African birch (Anogeissus leiocarpus) leaf and its effect on the α-amylase and α-glucosidase inhibitory properties of acarbose, J. Taibah Univ. Med. Sci., 2016, 11, 236–242 Search PubMed
.
- A. L. de la Garza, F. I. Milagro, N. Boque, J. Campion and J. A. Martinez, Natural Inhibitors of Pancreatic Lipase as New Players in Obesity Treatment, Planta Med., 2011, 77, 773–785 CrossRef CAS PubMed
.
- G. D. Kolovou, D. P. Mikhailidis, B. G. Nordestgaard, H. Bilianou and G. Panotopoulos, Definition of Postprandial Lipaemia, Curr. Vasc. Pharmacol., 2011, 9, 292–301 CrossRef CAS PubMed
.
- N. Yuda, M. Tanaka, M. Suzuki, Y. Asano, H. Ochi and K. Iwatsuki, Polyphenols Extracted from Black Tea (Camellia sinensis) Residue by Hot-Compressed Water and Their Inhibitory Effect on Pancreatic Lipase in vitro, J. Food Sci., 2012, 77, H254–H261 CrossRef PubMed
.
- Y. T. Zhu, X. Y. Ren, L. Yuan, Y. M. Liu, J. Liang and X. Liao, Fast identification of lipase inhibitors in oolong tea by using lipase functionalised Fe3O4 magnetic nanoparticles coupled with UPLC-MS/MS, Food Chem., 2015, 173, 521–526 CrossRef CAS PubMed
.
- P. Worsztynowicz, M. Napierala, W. Bialas, W. Grajek and M. Olkowicz, Pancreatic alpha-amylase and lipase inhibitory activity of polyphenolic compounds present in the extract of black chokeberry (Aronia melanocarpa L.), Process Biochem., 2014, 49, 1457–1463 CrossRef CAS
.
- E. Thomas, S. Manuel, F. Sigrid and K. Klaus,
In vitro Inhibition of Human Lipase PS by Polyphenols From Kiwi Fruit, J. Food Res., 2014, 3(4), 71–77 CrossRef CAS
.
- Y. H. S. Wu, C. H. Chiu, D. J. Yang, Y. L. Lin, J. K. Tseng and Y. C. Chen, Inhibitory effects of litchi (Litchi chinensis Sonn.) flower-water extracts on lipase activity and diet-induced obesity, J. Funct. Foods, 2013, 5, 923–929 CrossRef
.
- Y. L. Yu, X. M. Sun and F. Gao, Inhibitory Effect of Polyphenols Extracts from Peanut Shells on the Activity of Pancreatic Lipase in vitro, Asian J. Chem., 2014, 26, 3401–3403 CAS
.
- M. Kobayashi, M. Ichitani, Y. Suzuki, T. Unno, T. Sugawara, T. Yamahira, M. Kato, T. Takihara, Y. Sagesaka, T. Kakuda and I. Ikeda, Black-Tea Polyphenols Suppress Postprandial Hypertriacylglycerolemia by Suppressing Lymphatic Transport of Dietary Fat in Rats, J. Agric. Food Chem., 2009, 57, 7131–7136 CrossRef CAS PubMed
.
- G. Ramirez, A. Zamilpa, M. Zavala, J. Perez, D. Morales and J. Tortoriello, Chrysoeriol and other polyphenols from Tecoma stans with lipase inhibitory activity, J. Ethnopharmacol., 2016, 185, 1–8 CrossRef CAS PubMed
.
- X. L. Wu, W. Y. He, H. P. Zhang, Y. Li, Z. G. Liu and Z. D. He, Acteoside: A lipase inhibitor from the Chinese tea Ligustrum purpurascens kudingcha, Food Chem., 2014, 142, 306–310 CrossRef CAS PubMed
.
- M. P. Raghavendra, P. R. Kumar and V. Prakash, Mechanism of inhibition of rice bran lipase by polyphenols - A case study with chlorogenic acid and caffeic acid, J. Food Sci., 2007, 72, E412–E419 CrossRef CAS PubMed
.
- Q. You, F. Chen, X. Wang, P. J. G. Luo and Y. M. Jiang, Inhibitory Effects of Muscadine Anthocyanins on alpha-Glucosidase and Pancreatic Lipase Activities, J. Agric. Food Chem., 2011, 59, 9506–9511 CrossRef CAS PubMed
.
- F. Martins, T. M. Noso, V. B. Porto, A. Curiel, A. Gambero, D. H. M. Bastos, M. L. Ribeiro and P. d. O. Carvalho, Maté Tea Inhibits In Vitro Pancreatic Lipase Activity and Has Hypolipidemic Effect on High-fat Diet-induced Obese Mice, Obesity, 2010, 18, 42–47 CrossRef CAS PubMed
.
- T. Buchholz and M. F. Melzig, Polyphenolic Compounds as Pancreatic Lipase Inhibitors, Planta Med., 2015, 81, 771–783 CrossRef CAS PubMed
.
- A. T. M. A. Rahim, Y. Takahashi and K. Yamaki, Mode of pancreatic lipase inhibition activity in vitro by some flavonoids and non-flavonoid polyphenols, Food Res. Int., 2015, 75, 289–294 CrossRef CAS
.
- M. T. García-Conesa, Dietary Polyphenols against Metabolic Disorders: How Far Have We Progressed in the Understanding of the Molecular Mechanisms of Action of These Compounds?, Crit. Rev. Food Sci. Nutr., 2015 DOI:10.1080/10408398.2014.980499
.
- Y. Li, F. Q. Lu, Y. Feng, Z. D. He and X. L. Wu, Binding interaction between (−)-epigallocatechin-3-gallate (EGCG) of green tea and pepsin, Acta Aliment., 2016, 45, 129–140 CrossRef CAS
.
- X. Wu, W. He, W. Wang, X. Luo, H. Cao, L. Lin, K. Feng and Z. Liu, Investigation of the interaction between (−)-epigallocatechin-3-gallate with trypsin and α-chymotrypsin, Int. J. Food Sci. Technol., 2013, 48, 2340–2347 CrossRef CAS
.
- F. C. Cui, K. C. Yang and Y. Q. Li, Investigate the Binding of Catechins to Trypsin Using Docking and Molecular Dynamics Simulation, PLoS One, 2015, 10(5), e0125848 Search PubMed
.
- H. Z. Xiao, B. G. Liu, H. Z. Mo and G. Z. Liang, Comparative evaluation of tannic acid inhibiting alpha-glucosidase and trypsin, Food Res. Int., 2015, 76, 605–610 CrossRef CAS
.
- X. L. Wu, W. P. Wang, T. Zhu, T. Liang, F. Q. Lu, W. Y. He, H. P. Zhang, Z. G. Liu, S. H. He, K. P. Gao and Z. D. He, Phenylpropanoid glycoside inhibition of pepsin, trypsin and alpha-chymotrypsin enzyme activity in Kudingcha leaves from Ligustrum purpurascens, Food Res. Int., 2013, 54, 1376–1382 CrossRef CAS
.
- M. Stojadinovic, J. Radosavljevic, J. Ognjenovic, J. Vesic, I. Prodic, D. Stanic-Vucinic and T. C. Velickovic, Binding affinity between dietary polyphenols and beta-lactoglobulin negatively correlates with the protein susceptibility to digestion and total antioxidant activity of complexes formed, Food Chem., 2013, 136, 1263–1271 CrossRef CAS PubMed
.
- T. Goto, M. Horita, H. Nagai, A. Nagatomo, N. Nishida, Y. Matsuura and S. Nagaoka, Tiliroside, a glycosidic flavonoid, inhibits carbohydrate digestion and glucose absorption in the gastrointestinal tract, Mol. Nutr. Food Res., 2012, 56, 435–445 CAS
.
- O. Kwon, P. Eck, S. L. Chen, C. P. Corpe, J. H. Lee, M. Kruhlak and M. Levine, Inhibition of the intestinal glucose transporter GLUT2 by flavonoids, FASEB J., 2007, 21, 366–377 CrossRef CAS PubMed
.
- J. Song, O. Kwon, S. L. Chen, R. Daruwala, P. Eck, J. B. Park and M. Levine, Flavonoid inhibition of sodium-dependent vitamin C transporter 1 (SVCT1) and glucose transporter isoform 2 (GLUT2), intestinal transporters for vitamin C and glucose, J. Biol. Chem., 2002, 277, 15252–15260 CrossRef CAS PubMed
.
- Y. Kobayashi, M. Suzuki, H. Satsu, S. Arai, Y. Hara, K. Suzuki, Y. Miyamoto and M. Shimizu, Green tea polyphenols inhibit the sodium-dependent glucose transporter of intestinal epithelial cells by a competitive mechanism, J. Agric. Food Chem., 2000, 48, 5618–5623 CrossRef CAS PubMed
.
- R. Cermak, S. Landgraf and S. Wolffram, Quercetin glucosides inhibit glucose uptake into brush-border-membrane vesicles of porcine jejunum, Br. J. Nutr., 2007, 91, 849–855 CrossRef PubMed
.
- D. Feng, L. Ohlsson and R.-D. Duan, Curcumin inhibits cholesterol uptake in Caco-2 cells by down-regulation of NPC1L1 expression, Lipids Health Dis., 2010, 9, 1–5 CrossRef PubMed
.
- P. Kumar, P. Malhotra, K. Ma, A. Singla, O. Hedroug, S. Saksena, P. K. Dudeja, R. K. Gill and W. A. Alrefai, SREBP2 mediates the modulation of intestinal NPC1L1 expression by curcumin, Am. J. Physiol. Gastr. L, 2011, 301, G148–G155 CrossRef CAS PubMed
.
- B. Kim, Y. Park, C. J. Wegner, B. W. Bolling and J. Y. Lee, Polyphenol-rich black chokeberry (Aronia melanocarpa) extract regulates the expression of genes critical for intestinal cholesterol flux in Caco-2 cells, J. Nutr. Biochem., 2013, 24, 1564–1570 CrossRef CAS PubMed
.
- Z. Z. Ge, W. Zhu, J. M. Peng, X. Y. Deng and C. M. Li, Persimmon tannin regulates the expression of genes critical for cholesterol absorption and cholesterol efflux by LXR alpha independent pathway, J. Funct. Foods, 2016, 23, 283–293 CrossRef CAS
.
- M. Estudante, J. G. Morais, G. Soveral and L. Z. Benet, Intestinal drug transporters: An overview, Adv. Drug Delivery Rev., 2013, 65, 1340–1356 CrossRef CAS PubMed
.
- P. Matsson and C. A. Bergstrom, Computational modeling to predict the functions and impact of drug transporters, In Silico Pharmacol., 2015, 3, 8 CrossRef PubMed
.
- G. R. Velderrain-Rodriguez, H. Palafox-Carlos, A. Wall-Medrano, J. F. Ayala-Zavala, C. Y. O. Chen, M. Robles-Sanchez, H. Astiazaran-Garcia, E. Alvarez-Parrilla and G. A. Gonzalez-Aguilar, Phenolic compounds: their journey after intake, Food Funct., 2014, 5, 189–197 CAS
.
- Z. Li, H. Jiang, C. M. Xu and L. W. Gu, A review: Using nanoparticles to enhance absorption and bioavailability of phenolic phytochemicals, Food Hydrocolloids, 2015, 43, 153–164 CrossRef CAS
.
- J. M. Anderson, Molecular structure of tight junctions and their role in epithelial transport, News Physiol. Sci., 2001, 16, 126–130 CAS
.
- T. Matsui, Condensed catechins and their potential health-benefits, Eur. J. Pharmacol., 2015, 765, 495–502 CrossRef CAS PubMed
.
- F. Martel, R. Monteiro and C. Calhau, Effect of polyphenols on the intestinal and placental transport of some bioactive compounds, Nutr. Res. Rev., 2010, 23, 47–64 CrossRef CAS PubMed
.
-
Q. Wang and M. Li, Presystemic and First-Pass Metabolism, 2016 Search PubMed
.
- M. D. Contreras, I. Borras-Linares, M. Herranz-Lopez, V. Micol and A. Segura-Carretero, Further exploring the absorption and enterocyte metabolism of quercetin forms in the Caco-2 model using nano-LC-TOF-MS, Electrophoresis, 2016, 37, 998–1006 CrossRef CAS PubMed
.
- W. Zhou, J. J. Shan, S. C. Wang, B. C. Cai and L. Q. Di, Transepithelial transport of phenolic acids in Flos Lonicerae Japonicae in intestinal Caco-2 cell monolayers, Food Funct., 2015, 6, 3072–3080 CAS
.
- S. Kobayashi, M. Shinohara, T. Nagai and Y. Konishi, Transport mechanisms for soy isoflavones and microbial metabolites dihydrogenistein and dihydrodaidzein across monolayers and membranes, Biosci., Biotechnol., Biochem., 2013, 77, 2210–2217 CrossRef CAS PubMed
.
-
Cheminformatics, Molinspiration, http://www.molinspiration.com/ (accessed June 1st, 2016).
- A. Goncalves, S. Roi, M. Nowicki, A. Dhaussy, A. Huertas, M. J. Amiot and E. Reboul, Fat-soluble vitamin intestinal absorption: Absorption sites in the intestine and interactions for absorption, Food Chem., 2015, 172, 155–160 CrossRef CAS PubMed
.
- J. Iqbal and M. M. Hussain, Intestinal lipid absorption, Am. J. Physiol. Endocrinol. Metab., 2009, 296, E1183–E1194 CrossRef CAS PubMed
.
- A. Soler, M. P. Romero, A. Macia, S. Saha, C. S. M. Furniss, P. A. Kroon and M. J. Motilva, Digestion stability and evaluation of the metabolism and transport of olive oil phenols in the human small-intestinal epithelial Caco-2/TC7 cell line, Food Chem., 2010, 119, 703–714 CrossRef CAS
.
- H. Rastogi and S. Jana, Evaluation of physicochemical properties and intestinal permeability of six dietary polyphenols in human intestinal colon adenocarcinoma Caco-2 cells, Eur. J. Drug Metab. Pharmacokinet., 2016, 41, 33–43 CrossRef CAS PubMed
.
- L. Poquet, M. N. Clifford and G. Williamson, Transport and metabolism of ferulic acid through the colonic epithelium, Drug Metab. Dispos., 2008, 36, 190–197 CrossRef CAS PubMed
.
- R. Campos-Vega, K. Vazquez-Sanchez, D. Lopez-Barrera, G. Loarca-Pina, S. Mendoza-Diaz and B. D. Oomah, Simulated gastrointestinal digestion and in vitro colonic fermentation of spent coffee (Coffea arabica L): Bioaccessibility and intestinal permeability, Food Res. Int., 2015, 77, 156–161 CrossRef CAS
.
- J. P. Piwowarski, S. Granica, M. Zwierzynska, J. Stefanska, P. Schopohl, M. F. Melzig and A. K. Kiss, Role of human gut microbiota metabolism in the anti-inflammatory effect of traditionally used ellagitannin-rich plant materials, J. Ethnopharmacol., 2014, 155, 801–809 CrossRef CAS PubMed
.
- L. Marin, E. M. Miguelez, C. J. Villar and F. Lombo, Bioavailability of dietary polyphenols and gut microbiota metabolism: antimicrobial properties, BioMed Res. Int., 2015, 2015, 905215 Search PubMed
.
- D. J. McClements and H. Xiao, Potential biological fate of ingested nanoemulsions:
influence of particle characteristics, Food Funct., 2012, 3, 202–220 CAS
.
- Y. Konishi, Y. Hitomi and E. Yoshioka, Intestinal absorption of p-coumaric and gallic acids in rats after oral administration, J. Agric. Food Chem., 2004, 52, 2527–2532 CrossRef CAS PubMed
.
- Y. Konishi and S. Kobayashi, Transepithelial transport of rosmarinic acid in intestinal Caco-2 cell monolayers, Biosci., Biotechnol., Biochem., 2005, 69, 583–591 CrossRef CAS PubMed
.
- R. H. Stephens, J. Tanianis-Hughes, N. B. Higgs, M. Humphrey and G. Warhurst, Region-dependent modulation of intestinal permeability by drug efflux transporters: In vitro studies in mdr1a(-/-) mouse intestine, J. Pharmacol. Exp. Ther., 2002, 303, 1095–1101 CrossRef CAS PubMed
.
- T. Suzuki and H. Hara, Role of flavonoids in intestinal tight junction regulation, J. Nutr. Biochem., 2011, 22, 401–408 CrossRef CAS PubMed
.
- N. M. A. Hassimotto, M. I. Genovese and F. M. Lajolo, Absorption and metabolism of cyanidin-3-glucoside and cyanidin-3-rutinoside extracted from wild mulberry (Morus nigra L.) in rats, Nutr. Res., 2008, 28, 198–207 CrossRef CAS PubMed
.
- C. Braicu, M. R. Ladomery, V. S. Chedea, A. Irimie and L. Berindan-Neagoe, The relationship between the structure and biological actions of green tea catechins, Food Chem., 2013, 141, 3282–3289 CrossRef CAS PubMed
.
- T. Iwanaga, K. Takebe, I. Kato, S. I. Karaki and A. Kuwahara, Cellular expression of monocarboxylate transporters (MCT) in the digestive tract of the mouse, rat, and humans, with special reference to slc5a8, Biomed. Res., 2006, 27, 243–254 CrossRef CAS PubMed
.
- K. Ziegler, A. Kerimi, L. Poquet and G. Williamson, Butyric acid increases transepithelial transport of ferulic acid through upregulation of the monocarboxylate transporters SLC16A1 (MCT1) and SLC16A3 (MCT4), Arch. Biochem. Biophys., 2016, 599, 3–12 CrossRef CAS PubMed
.
- J. H. Lee, J. E. Lee, Y. Kim, H. Lee, H. J. Jun and S. J. Lee, Multidrug and Toxic Compound Extrusion Protein-1 (MATE1/SLC47A1) Is a Novel Flavonoid Transporter, J. Agric. Food Chem., 2014, 62, 9690–9698 CrossRef CAS PubMed
.
- W. V. De Castro, S. Mertens-Talcott, H. Derendorf and V. Butterweck, Effect of grapefruit juice, naringin, naringenin, and bergamottin on the intestinal carrier-mediated transport of talinolol in rats, J. Agric. Food Chem., 2008, 56, 4840–4845 CrossRef CAS PubMed
.
- R. Monteiro, C. Calhau, F. Martel, P. G. de Pinho and I. Azevedo, Intestinal uptake of MPP+ is differently affected by red and white wine, Life Sci., 2005, 76, 2483–2496 CrossRef CAS PubMed
.
- Y. Mimura, T. Yasujima, K. Ohta, K. Inoue and H. Yuasa, Functional identification of organic cation transporter 1 as an atenolol transporter sensitive to flavonoids, Biochem. Biophys. Rep., 2015, 2, 166–171 Search PubMed
.
- Y. Y. Yeap, N. L. Trevaskis, T. Quach, P. Tso, W. N. Charman and C. J. H. Porter, Intestinal Bile Secretion Promotes Drug Absorption from Lipid Colloidal Phases via Induction of Supersaturation, Mol. Pharmaceutics, 2013, 10, 1874–1889 CrossRef CAS PubMed
.
- K. Murota, S. Shimizu, S. Miyamoto, T. Izumi, A. Obata, M. Kikuchi and J. Terao, Unique uptake and transport of isoflavone aglycones by human intestinal Caco-2 cells: Comparison of isoflavonoids and flavonoids, J. Nutr., 2002, 132, 1956–1961 CAS
.
- I. Zanotti, M. Dall'Asta, P. Mena, L. Mele, R. Bruni, S. Ray and D. Del Rio, Atheroprotective effects of (poly)phenols: a focus on cell cholesterol metabolism, Food Funct., 2015, 6, 13–31 CAS
.
- M. Nait Chabane, A. Al Ahmad, J. Peluso, C. D. Muller and G. Ubeaud, Quercetin and naringenin transport across human intestinal Caco-2 cells, J. Pharm. Pharmacol., 2009, 61, 1473–1483 CrossRef PubMed
.
- L. S. Kaminsky and Q. Y. Zhang, The small intestine as a xenobiotic-metabolizing organ, Drug Metab.
Dispos., 2003, 31, 1520–1525 CrossRef CAS PubMed
.
- S. A. Peters, C. R. Jones, A.-L. Ungell and O. J. D. Hatley, Predicting Drug Extraction in the Human Gut Wall: Assessing Contributions from Drug Metabolizing Enzymes and Transporter Proteins using Preclinical Models, Clin. Pharmacokinet., 2016, 55, 673–696 CrossRef CAS PubMed
.
- M. Ulvestad, I. B. Skottheim, G. S. Jakobsen, S. Bremer, E. Molden, A. Asberg, J. Hjelmesaeth, T. B. Andersson, R. Sandbu and H. Christensen, Impact of OATP1B1, MDR1, and CYP3A4 Expression in Liver and Intestine on Interpatient Pharmacokinetic Variability of Atorvastatin in Obese Subjects, Clin. Pharmacol. Ther., 2013, 93, 275–282 CrossRef CAS PubMed
.
- A. Galetin, M. Gertz and J. B. Houston, Contribution of Intestinal Cytochrome P450-Mediated Metabolism to Drug-Drug Inhibition and Induction Interactions, Drug Metab. Pharmacokinet., 2010, 25, 28–47 CrossRef CAS PubMed
.
- A. de Boer, F. van Hunsel and A. Bast, Adverse food-drug interactions, Regul. Toxicol. Pharmacol., 2015, 73, 859–865 CrossRef CAS PubMed
.
- Y. Mano, Y. Sugiyama and K. Ito, Use of a Physiologically Based Pharmacokinetic Model for Quantitative Prediction of Drug-Drug Interactions via CYP3A4 and Estimation of the Intestinal Availability of CYP3A4 Substrates, J. Pharm. Sci., 2015, 104, 3183–3193 CrossRef CAS PubMed
.
- Y. Kimura, H. Ito, R. Ohnishi and T. Hatano, Inhibitory effects of polyphenols on human cytochrome P450 3A4 and 2C9 activity, Food Chem. Toxicol., 2010, 48, 429–435 CrossRef CAS PubMed
.
- T. M. Vijayakumar, R. M. Kumar, A. Agrawal, G. P. Dubey and K. Ilango, Comparative inhibitory potential of selected dietary bioactive polyphenols, phytosterols on CYP3A4 and CYP2D6 with fluorometric high-throughput screening, J. Food Sci. Technol. Mys, 2015, 52, 4537–4543 CrossRef CAS PubMed
.
- Q. H. Pu, L. Shi and C. Yu, Time-dependent inhibition of CYP3A4 by gallic acid in human liver microsomes and recombinant systems, Xenobiotica, 2015, 45, 213–217 CrossRef CAS PubMed
.
- P. M. de Magalhaes, I. Dupont, A. Hendrickx, A. Joly, T. Raas, S. Dessy, T. Sergent and Y. J. Schneider, Anti-inflammatory effect and modulation of cytochrome P450 activities by Artemisia annua tea infusions in human intestinal Caco-2 cells, Food Chem., 2012, 134, 864–871 CrossRef PubMed
.
- M. T. Holmberg, A. Tornio, H. Hyvarinen, M. Neuvonen, P. J. Neuvonen, J. T. Backman and M. Niemi, Effect of grapefruit juice on the bioactivation of prasugrel, Br. J. Clin. Pharmacol., 2015, 80, 139–145 CrossRef CAS PubMed
.
- J. W. Lee, J. K. Morris and N. J. Wald, Grapefruit Juice and Statins, Am. J. Med., 2016, 129, 26–29 CrossRef CAS PubMed
.
- Y. Akamine, M. Miura, H. Komori, I. Tamai, I. Ieiri, N. Yasui-Furukori and T. Uno, The change of pharmacokinetics of fexofenadine enantiomers through the single and simultaneous grapefruit juice ingestion, Drug Metab. Pharmacokinet, 2015, 30, 352–357 CrossRef CAS PubMed
.
- J. Q. Hu, D. W. Shang, X. W. Xu, X. L. He, X. J. Ni, M. Zhang, Z. Z. Wang, C. Qiu, S. H. Deng, H. Y. Lu, X. Q. Zhu, W. C. Huang and Y. G. Wen, Effect of grapefruit juice and food on the pharmacokinetics of pirfenidone in healthy Chinese volunteers: a diet-drug interaction study, Xenobiotica, 2016, 46, 516–521 CrossRef CAS PubMed
.
- J. S. Choi, Y. J. Piao and K. W. Kang, Effects of Quercetin on the Bioavailability of Doxorubicin in Rats: Role of CYP3A4 and P-gp Inhibition by Quercetin, Arch. Pharmacal Res., 2011, 34, 607–613 CrossRef CAS PubMed
.
- S. J. Choi, S. C. Shin and J. S. Choi, Effects of Myricetin on the Bioavailability of Doxorubicin for Oral Drug Delivery in Rats: Possible Role of CYP3A4 and P-glycoprotein Inhibition by Myricetin, Arch. Pharmacal Res., 2011, 34, 309–315 CrossRef CAS PubMed
.
- V. R. Challa, P. R. Babu, S.
R. Challa, B. Johnson and C. Maheswari, Pharmacokinetic interaction study between quercetin and valsartan in rats and in vitro models, Drug Dev. Ind. Pharm., 2013, 39, 865–872 CrossRef CAS PubMed
.
- N. Ikarashi, S. Ogawa, R. Hirobe, Y. Kusunoki, R. Kon, W. Ochiai and K. Sugiyama, High-dose green tea polyphenol intake decreases CYP3A expression in a liver-specific manner with increases in blood substrate drug concentrations, Eur. J. Pharm. Sci., 2016, 89, 137–145 CrossRef CAS PubMed
.
- Y. A. Cho, J. S. Choi and J. P. Burm, Effects of the antioxidant baicalein on the pharmacokinetics of nimodipine in rats: a possible role of P-glycoprotein and CYP3A4 inhibition by baicalein, Pharmacol. Rep., 2011, 63, 1066–1073 CrossRef CAS PubMed
.
- T. Wu, J. Wu, P. Gao, D. Xiang, D. Liu and H. Song, Effects of caffeine, tea polyphenol and daidzein on the pharmacokinetics of lansoprazole and its metabolites in rats, Braz. J. Pharm. Sci., 2015, 51, 893–899 CrossRef
.
- J. H. Lin and A. Y. H. Lu, Inhibition and induction of cytochrome P450 and the clinical implications, Clin. Pharmacokinet., 1998, 35, 361–390 CrossRef CAS PubMed
.
- O. F. Iwuchukwu, R. J. Tallarida and S. Nagar, Resveratrol in combination with other dietary polyphenols concomitantly enhances antiproliferation and UGT1A1 induction in Caco-2 cells, Life Sci., 2011, 88, 1047–1054 CrossRef CAS PubMed
.
- P. M. Dai, F. F. Luo, Y. Wang, H. Y. Jiang, L. P. Wang, G. Y. Zhang, L. J. Zhu, M. Hu, X. C. Wang, L. L. Lu and Z. Q. Liu, Species- and gender-dependent differences in the glucuronidation of a flavonoid glucoside and its aglycone determined using expressed UGT enzymes and microsomes, Biopharm. Drug Dispos., 2015, 36, 622–635 CrossRef CAS PubMed
.
- K. W. Bock, Human UDP-glucuronosyltransferases: Feedback loops between substrates and ligands of their transcription factors, Biochem. Pharmacol., 2012, 84, 1000–1006 CrossRef CAS PubMed
.
- Y. Hiura, H. Satsu, M. Hamada and M. Shimizu, Analysis of flavonoids regulating the expression of UGT1A1 via xenobiotic receptors in intestinal epithelial cells, Biofactors, 2014, 40, 336–345 CrossRef CAS PubMed
.
- L. Actis-Goretta, A. Lévèques, M. Rein, A. Teml, C. Schäfer, U. Hofmann, H. Li, M. Schwab, M. Eichelbaum and G. Williamson, Intestinal absorption, metabolism, and excretion of (–)-epicatechin in healthy humans assessed by using an intestinal perfusion technique, Am. J. Clin. Nutr., 2013, 98, 924–933 CrossRef CAS PubMed
.
- N. Stachel and G. Skopp, Identification and characterization of sulfonyltransferases catalyzing ethyl sulfate formation and their inhibition by polyphenols, Int. J. Legal Med., 2016, 130, 139–146 CrossRef PubMed
.
- J. Odenthal, B. W. H. van Heumen, H. M. J. Roelofs, R. H. M. te Morsche, B. Marian, F. M. Nagengast and W. H. M. Peters, The Influence of Curcumin, Quercetin, and Eicosapentaenoic Acid on the Expression of Phase II Detoxification Enzymes in the Intestinal Cell Lines HT-29, Caco-2, HuTu 80, and LT97, Nutr. Cancer, 2012, 64, 856–863 CrossRef CAS PubMed
.
- I. Gulcin, A. Scozzafava, C. T. Supuran, Z. Koksal, F. Turkan, S. Cetinkaya, Z. Bingol, Z. Huyut and S. H. Alwasel, Rosmarinic acid inhibits some metabolic enzymes including glutathione S-transferase, lactoperoxidase, acetylcholinesterase, butyrylcholinesterase and carbonic anhydrase isoenzymes, J. Enzyme Inhib. Med. Chem., 2016, 1–5 Search PubMed
.
- U. Lewandowska, J. Fichna and S. Gorlach, Enhancement of anticancer potential of polyphenols by covalent modifications, Biochem. Pharmacol., 2016, 109, 1–13 CrossRef CAS PubMed
.
- J. Fan and I. A. M. de Lannoy, Pharmacokinetics, Biochem. Pharmacol., 2014, 87, 93–120 CrossRef CAS PubMed
.
- Y. Li, C. Liu, Y. Zhang, S. Mi and N. Wang, Pharmacokinetics of ferulic acid and potential interactions with Honghua and clopidogrel in rats, J. Ethnopharmacol., 2011, 137, 562–567 CrossRef CAS PubMed
.
- Z. Ouyang, M. Zhao, J. Tang and L. Pan, In vivo pharmacokinetic comparisons of ferulic acid and puerarin after oral administration of monomer, medicinal substance aqueous extract and Nao-De-Sheng to rats, Pharmacogn. Mag., 2012, 8, 256–262 CrossRef PubMed
.
- Y. Y. Xie, X. M. Wang, S. H. Wang, Y. M. Wang, H. F. Tian, Y. S. Yuan, H. Y. Li, Q. L. Liang and G. A. Luo, Metabolism and pharmacokinetics of major polyphenol components in rat plasma after oral administration of total flavonoid tablet from Anemarrhenae Rhizoma, J. Chromatogr. B: Anal. Technol. Biomed. Life Sci., 2015, 1–5 Search PubMed
.
- A. K. Kammalla, M. K. Ramasamy, J. Chintala, G. P. Dubey, A. Agrawal and I. Kaliappan, Comparative pharmacokinetic interactions of Quercetin and Rutin in rats after oral administration of European patented formulation containing Hipphophae rhamnoides and Co-administration of Quercetin and Rutin, Eur. J. Drug Metab. Pharmacokinet., 2015, 40, 277–284 CrossRef CAS PubMed
.
- H. F. Su, Q. Lin, X. Y. Wang, Y. Fu, T. Gong, X. Sun and Z. R. Zhang, Absorptive interactions of concurrent oral administration of (+)-catechin and puerarin in rats and the underlying mechanisms, Acta Pharmacol. Sin., 2016, 37, 545–554 CrossRef CAS PubMed
.
- J. Duan, Y. Dang, H. Meng, H. Wang, P. Ma, G. Li, T. Wu and Y. Xie, A comparison of the pharmacokinetics of three different preparations of total flavones of Hippophae rhamnoides in beagle dogs after oral administration, Eur. J. Drug Metab. Pharmacokinet., 2016, 41, 239–249 CrossRef CAS PubMed
.
- B. A. Acosta-Estrada, J. A. Gutierrez-Uribe and S. O. Serna-Saldivar, Bound phenolics in foods, a review, Food Chem., 2014, 152, 46–55 CrossRef CAS PubMed
.
- N. Mateo Anson, R. van den Berg, R. Havenaar, A. Bast and G. R. M. M. Haenen, Bioavailability of ferulic acid is determined by its bioaccessibility, J. Cereal Sci., 2009, 49, 296–300 CrossRef CAS
.
- T. D. Costa, H. Rogez and R. D. Pena, Adsorption capacity of phenolic compounds onto cellulose and xylan, Food Sci. Technol., 2015, 35, 314–320 Search PubMed
.
- G. Velderrain-Rodríguez, A. Quirós-Sauceda, G. Mercado-Mercado, J. F. Ayala-Zavala, H. Astiazarán-García, R. M. Robles-Sánchez, A. Wall-Medrano, S. Sayago-Ayerdi and G. A. González-Aguilar, Effect of dietary fiber on the bioaccessibility of phenolic compounds of mango, papaya and pineapple fruits by an in vitro digestion model, Food Sci. Technol., 2016, 1–7 Search PubMed
.
- V. Ferraro, A. R. Madureira, B. Sarmento, A. Gomes and M. E. Pintado, Study of the interactions between rosmarinic acid and bovine milk whey protein alpha-Lactalbumin, beta-Lactoglobulin and Lactoferrin, Food Res. Int., 2015, 77, 450–459 CrossRef CAS
.
- M. Martinez-Huelamo, S. Tulipani, R. Estruch, E. Escribano, M. Illan, D. Corella and R. M. Lamuela-Raventos, The tomato sauce making process affects the bioaccessibility and bioavailability of tomato phenolics: a pharmacokinetic study, Food Chem., 2015, 173, 864–872 CrossRef CAS PubMed
.
- R. P. Feliciano, A. Boeres, L. Massacessi, G. Istas, M. R. Ventura, C. Nunes Dos Santos, C. Heiss and A. Rodriguez-Mateos, Identification and quantification of novel cranberry-derived plasma and urinary (poly)phenols, Arch. Biochem. Biophys., 2016, 599, 31–41 CrossRef CAS PubMed
.
- A. Stalmach, W. Mullen, D. Barron, K. Uchida, T. Yokota, C. Cavin, H. Steiling, G. Williamson and A. Crozier, Metabolite profiling of hydroxycinnamate derivatives in plasma and urine after the ingestion of coffee by humans: identification of biomarkers of coffee consumption, Drug Metab. Dispos., 2009, 37, 1749–1758 CrossRef CAS PubMed
.
- S. Wang, R. Su, S. Nie, M. Sun, J. Zhang, D. Wu and N. Moustaid-Moussa, Application of nanotechnology in improving
bioavailability and bioactivity of diet-derived phytochemicals, J. Nutr. Biochem., 2014, 25, 363–376 CrossRef CAS PubMed
.
- O. C. Farokhzad and R. Langer, Impact of nanotechnology on drug delivery, ACS Nano, 2009, 3, 16–20 CrossRef CAS PubMed
.
- S. H. Thilakarathna and H. Rupasinghe, Flavonoid bioavailability and attempts for bioavailability enhancement, Nutrients, 2013, 5, 3367–3387 CrossRef PubMed
.
- S. Haratifar, K. A. Meckling and M. Corredig, Bioefficacy of tea catechins encapsulated in casein micelles tested on a normal mouse cell line (4D/WT) and its cancerous counterpart (D/v-src) before and after in vitro digestion, Food Funct., 2014, 5, 1160–1166 CAS
.
- A. Guri, S. Haratifar and M. Corredig, Bioefficacy of tea catechins associated with milk caseins tested using different in vitro digestion models, Food Dig., 2014, 5, 8–18 CrossRef CAS
.
- A. K. Jain, K. Thanki and S. Jain, Co-encapsulation of Tamoxifen and Quercetin in Polymeric Nanoparticles: Implications on Oral Bioavailability, Antitumor Efficacy, and Drug-Induced Toxicity, Mol. Pharmaceutics, 2013, 10, 3459–3474 CrossRef CAS PubMed
.
- M. J. Rein, M. Renouf, C. Cruz-Hernandez, L. Actis-Goretta, S. K. Thakkar and M. da Silva Pinto, Bioavailability of bioactive food compounds: a challenging journey to bioefficacy, Br. J. Clin. Pharmacol., 2013, 75, 588–602 CAS
.
- L.-Q. Zou, W. Liu, W.-L. Liu, R.-H. Liang, T. Li, C.-M. Liu, Y.-L. Cao, J. Niu and Z. Liu, Characterization and bioavailability of tea polyphenol nanoliposome prepared by combining an ethanol injection method with dynamic high-pressure microfluidization, J. Agric. Food Chem., 2014, 62, 934–941 CrossRef CAS PubMed
.
- H. D. Silva, M. Â. Cerqueira and A. A. Vicente, Nanoemulsions for food applications: development and characterization, Food Bioprocess Technol., 2012, 5, 854–867 CrossRef CAS
.
- D. J. McClements, Nanoemulsions versus microemulsions: terminology, differences, and similarities, Soft Matter, 2012, 8, 1719–1729 RSC
.
- T. Baccarin, M. Mitjans, E. Lemos-Senna and M. P. Vinardell, Protection against oxidative damage in human erythrocytes and preliminary photosafety assessment of Punica granatum seed oil nanoemulsions entrapping polyphenol-rich ethyl acetate fraction, Toxicol. in Vitro, 2015, 30, 421–428 CrossRef CAS PubMed
.
- M. Nasr, Development of an optimized hyaluronic acid-based lipidic nanoemulsion co-encapsulating two polyphenols for nose to brain delivery, Drug Delivery, 2016, 23, 1444–1452 CrossRef CAS PubMed
.
- S. Rocha, R. Generalov, M. do Carmo Pereira, I. Peres, P. Juzenas and M. A. Coelho, Epigallocatechin gallate-loaded polysaccharide nanoparticles for prostate cancer chemoprevention, Nanomedicine, 2011, 6, 79–87 CrossRef CAS PubMed
.
- M. Singh, P. Bhatnagar, A. K. Srivastava, P. Kumar, Y. Shukla and K. C. Gupta, Enhancement of cancer chemosensitization potential of cisplatin by tea polyphenols poly (lactide-co-glycolide) nanoparticles, J. Biomed. Nanotechnol., 2011, 7, 202–202 CrossRef CAS PubMed
.
- Q. Huang, H. Yu and Q. Ru, Bioavailability and delivery of nutraceuticals using nanotechnology, J. Food Sci., 2010, 75, R50–R57 CrossRef CAS PubMed
.
- S. J. Hossain, H. Kato, H. Aoshima, T. Yokoyama, M. Yamada and Y. Hara, Polyphenol-induced inhibition of the response of Na+/glucose cotransporter expressed in Xenopus oocytes, J. Agric. Food Chem., 2002, 50, 5215–5219 CrossRef CAS PubMed
.
- D. Kaushik, K. O'Fallon, P. M. Clarkson, C. P. Dunne, K. R. Conca and B. Michniak-Kohn, Comparison of quercetin pharmacokinetics following oral supplementation in humans, J. Food Sci., 2012, 77, H231–H238 CrossRef CAS PubMed
.
- C. Gan, L. Liu, Y. Du, L. Wang, M. Gao, L. Wu and C. Yang, Simultaneous determination and pharmacokinetic study of four phenol compounds in rat plasma by ultra-high performance liquid chromatography with tandem mass spectrometry after oral administration of Echinacea purpurea extract, J. Sep. Sci., 2016, 39, 1628–1637 CrossRef CAS PubMed
.
- A. Stalmach, C. A. Edwards, J. D. Wightman and A. Crozier, Gastrointestinal stability and bioavailability of (poly)phenolic compounds following ingestion of Concord grape juice by humans, Mol. Nutr. Food Res., 2012, 56, 497–509 CAS
.
- E. U. Graefe, J. Wittig, S. Mueller, A. K. Riethling, B. Uehleke, B. Drewelow, H. Pforte, G. Jacobasch, H. Derendorf and M. Veit, Pharmacokinetics and bioavailability of quercetin glycosides in humans, J. Clin. Pharmacol., 2001, 41, 492–499 CrossRef CAS PubMed
.
|
This journal is © The Royal Society of Chemistry 2017 |