Humic acid ameliorates nanoparticle-induced developmental toxicity in zebrafish
Received
16th September 2016
, Accepted 4th November 2016
First published on 9th November 2016
Abstract
Many aquatic toxicity experiments are not performed under realistic environmental conditions. We examined several inorganic and organic nanoparticle (NP) formulations (citrate-capped silver NPs, carboxylic acid functionalized single-walled carbon nanotubes, mercaptoundecanoic acid functionalized cadmium selenide NPs, poly(acrylic acid) (PAA)-coated zinc oxide [ZnO] NPs, Nile red-loaded PAA nanocapsules, and uncoated sphere- and leaf-shaped ZnO NPs) to determine whether the presence of humic acid (HA) affects the physicochemical properties (e.g. size, zeta potential, and particle dissolution) of NPs in suspension and ameliorates noted effects on zebrafish (Danio rerio) development. We investigated the toxicological effects of these NPs with and without HA addition during early stages of zebrafish development by measuring survival, hatching success, length, head-tail angle, movement, and protease activity in the chorionic fluid, as well as NP interaction with the chorion. Though NP-induced effects on survival were not mitigated by the presence of HA, hatching inhibition and reduced head-tail angle in developing zebrafish caused by certain NPs were restored to near control values by HA addition. Interestingly, despite the ameliorating effects noted with the addition of HA, combined NP and HA treatments still resulted in reduced enzyme activity and NP interaction with the zebrafish embryo. We suggest that observed effects were NP-specific and not attributed to ionic metal species. In the interest of performing more environmentally representative toxicity studies, HA should be included in standardized laboratory nanotoxicity tests since it alters certain biological effects.
Environmental significance
There is a growing concern as to whether current nanotoxicity testing methods represent realistic environmental conditions. Natural organic matter is a ubiquitous component of natural waters that can alter the fate, bioavailability, and toxicity of nanoparticles in the environment. We characterized a variety of aqueous nanoparticle suspensions and examined their effects using early-stage zebrafish (Danio rerio); endpoints including survival, hatching success, length, head-tail angle, movement, and protease activity in chorionic fluid were assessed. This study provides evidence that humic acid ameliorates hatching inhibition and reduced head-tail angle caused by nanoparticles. However, the presence of humic acid did not influence nanoparticle-induced effects on survival, enzyme activity, or the potential for nanoparticle interaction with the embryo.
|
1 Introduction
Accidental or deliberate release of engineered nanoparticles (NPs) into natural waters may pose a risk to aquatic ecosystem health.1–3 Given that evidence supporting NP-induced negative effects on aquatic organisms is mounting, there is elevated concern regarding whether current laboratory testing methods adequately represent realistic environmental conditions.4 Although there are an increasing number of studies conducted under environmental conditions (e.g. mesocosms) and that consider variable water chemistry, the majority of current research is performed under standard laboratory conditions.5 Some NPs may be less toxic under natural conditions than in pristine culture experiments while others could become more biologically available and toxic due to abiotic and biotic transformations in complex ecosystems.6 Determining which environmental factors may mitigate or exacerbate NP toxicity, and establishing whether conditions in current standardized laboratory tests are representative of the real environment is essential. Furthermore, most testing protocols need to be evaluated and adjusted to ensure their suitability for use with NPs.
A robust risk assessment requires a fuller understanding of the fate, behavior, and toxicity of NPs in physicochemically complex and highly variable natural waters.7 Abiotic factors including ionic strength, pH, and natural organic matter (NOM) have been shown to affect the colloidal stability of engineered NPs released in natural environments.8,9 NOM has been reported to decrease the severity of biological effects resulting from exposure to organic chemicals including diazinon and pentachlorophenol10 and metals including silver (Ag) and cadmium (Cd).11,12 Metal ion release is also a key factor in metal-oxide NP toxicity and Suwannee River fulvic acid can modulate zinc oxide (ZnO) NP dissolution by covering the surface of the NP and by complexing zinc ions released into the surrounding medium.13 NOM can alter the uptake, bioavailability, and toxicity of various substances,10 but is itself redox active14 and has the potential to negatively affect aquatic organisms.15–17
An important component of natural water sources is humic acid (HA), a type of NOM that results from the decay of organic matter.14 Humic substances are ubiquitous in aquatic environments and have several unique characteristics.14 The molecular weights and surface functional groups of HAs can vary considerably,14 depending on the origin of the components and the environmental conditions that shape its formation.18 It is well known that HA can interact directly with NPs forming an ecocorona that alters the effective surface charge of the particle and prevents aggregation through repulsive electrostatic and/or steric interactions.19 Few studies have addressed the biological impact of NPs on fishes in the presence of HA, and these have focused mostly on potential interactions with titanium dioxide NPs20 or carbon-based nanomaterials.21 Fish during early developmental stages are sensitive to various toxicants, including metals,22 and are thus useful test organisms for assessing aquatic hazard.23 The use of zebrafish (Danio rerio) embryos for acute toxicity testing is efficient, cost-effective, and well-developed.23 In previous laboratory studies,24 we have identified a variety of metal-based and non-metallic NPs that inhibit zebrafish hatch, which can lead to mortality.25 In the present study, we tested seven different NP formulations (citrate-capped silver NPs, carboxylic acid functionalized single-walled carbon nanotubes, mercaptoundecanoic acid functionalized cadmium selenide NPs, poly(acrylic acid) (PAA)-coated zinc oxide [ZnO] NPs, Nile red-loaded PAA nanocapsules, and uncoated sphere- and leaf-shaped ZnO NPs) to determine whether the presence of HA could alter survival, hatching success, length, head-tail angle, movement, and protease activity (chorionic fluid) in early-stage zebrafish, as well as NP interactions with the chorion. An extensive assessment of multiple NP formulations could better direct risk assessment procedures and failure to consider HA in toxicity testing methods could lead to substantial underestimation or exaggeration of the risk associated with the release of NPs into aquatic systems.
2 Materials and methods
2.1 Nanoparticle synthesis and stock preparation
Seven NP formulations were synthesized as follows: 1. A citrate-capped Ag NP stock suspension (4 g L−1) was produced as described by Schultz et al.26 2. Non-functionalized single-walled carbon nanotubes (SWCNTs) were synthesized as described by Kim et al.,27,28 and then oxidized in a HNO3/H2SO4 mixture for 2 h at 30 °C as reported by Price et al.,29 yielding a 1 g L−1 stock suspension of carboxylic acid functionalized SWCNTs. 3. Cadmium selenide (CdSe) NPs were manufactured as described by Zhong et al.30 and functionalized with mercaptoundecanoic acid. CdSe NPs were dialyzed overnight with a Slide-A-Lyzer dialysis cassette (2 K molecular weight cutoff [MWCO], ThermoFisher Scientific) to remove metal impurities and the 0.591 g L−1 stock suspension was stored under argon to prevent oxidation. 4. Poly(acrylic acid) (PAA)-coated ZnO NPs (10 g L−1; henceforth referred to as ZnO) and 5. Powdered Nile red-loaded PAA nanocapsules (NR-NCs) were provided by Vive Crop Protection Inc. (Toronto, Canada). Uncoated 6. Sphere (sph)- and 7. Leaf-shaped ZnO NPs in powder form were prepared as described in Heng et al.31 All NP stock suspensions were prepared in double-distilled water (ddH2O; Millipore) and stored at room temperature in the dark unless otherwise indicated. Stock suspensions were diluted in E3 medium (5 mM NaCl, 0.17 mM KCl, 0.33 mM CaCl2·2H2O, 0.33 mM MgSO4·7H2O) immediately prior to in vivo exposure. Suwannee River HA (International Humic Substances Society) was hydrated in E3 medium overnight in the dark and made fresh every two weeks (1 g L−1).
2.2 Nanoparticle characterization
All of the NPs used in this study, except ZnO, have been previously reported to affect hatching success and full characterizations including hydrodynamic diameter, zeta (ζ)-potential, and dissolution of particles suspended in dechlorinated tap water or ddH2O are available in those studies.24,25,32 Hydrodynamic diameter, polydispersity index and ζ-potential of 10, 100, or 200 mg L−1 NPs prepared in either E3 medium or E3 medium containing 10 mg L−1 HA (henceforth referred to as NPs or NPs + HA, respectively) to represent exposure conditions were measured in triplicate using a Malvern Instrument Zetasizer Nano ZS equipped with a 633 nm laser (Westborough, MA) and measured in 173° backscatter mode. Dissolution of ZnO NPs (ZnO, ZnO sph, and ZnO leaf) in the absence and presence of HA was measured using a procedure adapted from Felix et al.32 and Ong et al.25 Briefly, 2 mL samples were collected initially (0 h) from polypropylene beakers (Nalgene) containing 250 mL ddH2O; beakers were submerged in 1% HNO3 (70%, ACS reagent, Sigma-Aldrich) overnight and washed thoroughly. Slide-A-Lyzer dialysis cassettes (2 K MWCO, ThermoFisher Scientific) were injected with either 0.5 mL ZnO NP stock suspension (100 mg L−1) or control (E3 medium or E3 medium + HA). Each cassette was suspended in individual covered beakers using float buoys, stirred with a constant slow rotation for 30 min to ensure equilibration, and then transferred to a separate beaker filled with 250 mL fresh ddH2O for a 48 h dialysis period. All 2 mL samples including ZnO NP stock suspensions and those removed from the diluent at 0, 24 and 48 h were acidified with 60 μL 10 M HNO3 (≥69%, TraceSELECT, Sigma-Aldrich) and stored in the dark at room temperature until analysis. Ionic Zn metal present in stock suspensions and particle dissolution over 48 h was measured using an Agilent 240-FS atomic absorption spectrometer (AAS) outfitted with a GTA 120 (graphite tube analyzer, Agilent, USA). Pyrolytically coated, partitioned graphite tubes were used for analysis. A calibration curve was constructed by measuring the absorbance of standard Zn solutions (1.5, 3, and 6 μg L−1) prepared in 5% traceSELECT HNO3 (Sigma) and ddH2O, and by using the new rational-method algorithm. Samples were injected in 10 μL volumes and read in duplicate at 213.9 nm using a slit width of 1.0 nm under an argon environment. A high intensity, coded, multi-element UltrAA lamp (Agilent, USA) was used with a lamp booster, a lamp current of 5.0 mA, and all signals were deuterium background corrected. Instrument gain was 42% at the start of the run. Resloping of the calibration curve was completed every 10 samples and recalibration was completed every 20 samples. Samples with concentrations outside the available calibration curve, i.e. >6 μg L−1 Zn, were either auto-diluted by the instrument or diluted by the analyst and then the appropriate dilution factor was applied. Blanks, duplicates, and a certified reference material (SLRS-6, River water, National Research Council of Canada; NRC) were used for quality assurance/quality control. The certified reference material had a certified Zn concentration of 1.76 ± 0.12 μg L−1 and readings were within 10% of this value. The estimated detection limit was >0.1 μg L−1 Zn.
2.3 Zebrafish maintenance and embryo collection
Zebrafish embryos were collected from breeding pairs of wild-type AB or ISL1-GFP-Wik adult zebrafish (aged 6 to 18 months) raised in the Aquatics Facility at the University of Alberta. Adult fish were kept in 30 L tanks with continuous water flow (conductivity: 1350 ± 100 μS cm−1, pH: 7.7 ± 0.2, temperature: 28.5 ± 0.7 °C, general water hardness: 100 ppm, carbonate hardness: 10 ppm, salinity: 0.5 ppt) on a 14 h light/10 h dark cycle. On nights prior to embryos being required, five adult zebrafish (male-to-female ratio of 2
:
3) were placed in 1 L breeding tanks containing fresh water. Within 10 min of the lights turning on in the morning, the water was changed, and embryos were collected shortly thereafter.
2.4 Survival, hatch, length, and head-tail angle observations
After cleavage stage (0.75–2 h),33 embryos were collected and rinsed with E3 medium. Embryos from the same breeding adults were sorted into groups of 30 embryos in a 6-well polystyrene plate (Corning). The extra liquid was removed and 5 mL of treatment suspension was vortexed for 15 s and added to each well. Treatments were as follows: 10 mg L−1 Ag NPs, 200 mg L−1 SWCNTs, 10 mg L−1 CdSe NPs, 100 mg L−1 ZnO NPs, 100 mg L−1 NR-NCs, 100 mg L−1 ZnO sph NPs, and 100 mg L−1 ZnO leaf NPs, with and without 10 mg L−1 HA addition. Controls (E3 medium or E3 medium + HA) were run internally on each plate, and plates were only used in analyses if control survival was ≥80%.
Survival was monitored at 24, 48, 72, and 96 hours post-fertilization (hpf), and dead embryos and larvae, identified by an opaque mass within the chorion or no apparent heartbeat,34 were removed at each time point. Hatching success was recorded at 48, 72 and 96 hpf. The length and head-tail angle of larvae were measured as described by Kimmel et al.33 at 24, 48, and 72 hpf. For these endpoints, three embryos/treatment were randomly selected, measured using a light microscope (Zeiss Observer HB050), and evaluated with digital imaging software (AxioVs40 software, v. 4.7.2). Survival, hatching success, length, and head-tail angle measurements were repeated six times/treatment.
2.5 Spontaneous movement and touch response
Groups of ten 48 hpf embryos, exposed in the same manner as above (see section 2.4), were videotaped for 10 min intervals and spontaneous movements (i.e. any distinguishable movement) were manually counted. Spontaneous movement trials were repeated three times. Touch response was monitored at 72 hpf; any embryos that were still within the chorion were manually dechorionated with forceps and left undisturbed for 1 h. Fish were gently touched at the midsection with a piece of monofilament fishing line and if the fish responded and swam any distance, they were considered responsive. Experiments were repeated three times/treatment and 50 fish/replicate were tested for touch response.
2.6 Protease assays
Protease activity was measured in the chorionic fluid of embryonic zebrafish exposed to Ag, CdSe, ZnO, ZnO sph, and ZnO leaf NPs to determine if the addition of HA modulates hatching enzyme activity. After 48 hpf, embryos were observed and when approximately 5% of embryos had hatched ∼400 embryos were collected and transferred to 2 mL E3 medium. Nitrogen was bubbled through the water to create an anoxic environment thereby inducing hatch. When approximately 80% of the fish had hatched, the fluid was filtered through a fine mesh and syringe, and immediately frozen at −80 °C. A G-Biosciences protease kit (catalogue #786-028) was used to assess protease activity. Thawed chorionic fluid was incubated with E3 medium, HA, NPs, or NPs + HA at 28.5 °C for 4 h as per kit instructions. Control wells containing chorionic fluid without NPs were run alongside each treatment. To test for NP-assay interference, we performed controls for NP interference (i.e. NPs were added to assay components and monitored for changes in optical density without chorionic fluid). We confirmed that none of the NPs interfered with the assay, with the exception of SWCNTs, which had high intrinsic absorbance that affected the final absorbance reading and was therefore not included in this experiment (data not shown). Each treatment was run in duplicate on each plate and repeated, at least, three times.
2.7 Nanoparticle–humic acid interaction with zebrafish embryos
To visually investigate whether HA plays a role in NP sorption to early-stage zebrafish, 1–2 hpf transgenic embryos expressing green fluorescent protein (ISL1-GFP-Wik) were exposed to 100 mg L−1 NR-NCs in the presence or absence of HA for 24 h at 28.5 ± 1 °C in the dark. After this exposure period, embryos were prepared for confocal imaging according to the procedure described by Felix et al.32 with green fluorescent protein (GFP) excited by an argon laser (λ = 488 nm) and the emission captured with a 525/50 nm band-pass emission filter. We chose NR-NCs because their PAA composition (without Nile red) is identical to the PAA coating encapsulating the ZnO NPs examined in our study;32 unfortunately, visualization of the other NPs used in this experiment was not possible using laser scanning confocal microscopy.
2.8 Statistical analysis
A one-way analysis of variance (ANOVA) was performed followed by post hoc Tukey’s Multiple Comparison Test. Results were considered statistically significant if p < 0.05. All values are reported as means ± standard error of the mean. GraphPad Prism v.6.0 was used to perform statistical analyses and to create graphs.
3 Results and discussion
3.1 Physicochemical properties of nanoparticles with and without humic acid addition
Various environmental factors influence the bioavailability and toxicity of NPs, and these factors are often not considered in laboratory experiments. Ubiquitous in all bodies of water are HAs, and in this study, we demonstrate that 10 mg L−1 HA alters both the physicochemical characteristics of NPs and their effects on zebrafish embryos. We chose 10 mg L−1 HA based on findings of Gao et al.35 who demonstrated that Ag NPs were more dispersed in the presence of <10 mg L−1 total organic carbon and became agglomerated when >10 mg L−1 NOM was added. The hydrodynamic diameters of all NP suspensions tested, except CdSe NPs, were reduced when HA was added and showed mid-range (0.08–0.7) to high polydispersity (>0.7) (Table 1). The CdSe NP agglomerate sizes increased from 39 ± 19.2 nm to 114 ± 38.8 nm in the presence of HA and were very polydispersed (Table 1). The interaction between HA and NP surfaces, indicated by their change in mean hydrodynamic diameter and decreased polydispersity index (i.e. more monodispersed aggregates) (Table 1), likely prevents binding of the NPs to membranes and thus limits NP damage.36,37 Membrane–NP interaction can induce injury via oxidative damage or reduction of membrane potential, and allow for uptake of NPs in embryonic fish.38,39 NOM can adsorb to, and coat, carbon40,41 and metallic42–44 NPs, and may even replace the engineered capping agents.44 As a consequence, surface properties are modified and alter NP–membrane interactions both sterically and electrostatically.43,45
Table 1 Physicochemical characteristics of 10, 100, and 200 mg L−1 NPs in the absence and presence of 10 mg L−1 Suwannee River HA
Treatment |
Hydrodynamic diameter (nm) |
Polydispersity index |
ζ-Potential (mV) |
Ag 10 |
93 ± 1.0 |
0.23 |
−19 ± 0.3 |
Ag 10 + HA |
86 ± 6.1 |
0.27 |
−18 ± 0.4 |
SWCNT 200 |
385 ± 48.7 |
0.94 |
−18 ± 0.1 |
SWCNT 200 + HA |
189 ± 69.9 |
0.75 |
−24 ± 0.2 |
CdSe 10 |
39 ± 19.2 |
1.00 |
−13 ± 0.4 |
CdSe 10 + HA |
114 ± 38.8 |
0.93 |
−10 ± 0.2 |
ZnO 100 |
19 ± 0.3 |
0.20 |
−20 ± 1.5 |
ZnO 100 + HA |
13 ± 0.2 |
0.28 |
−14 ± 0.5 |
ZnO sph 100 |
272 ± 41.3 |
0.92 |
+2 ± 0.1 |
ZnO sph 100 + HA |
202 ± 8.0 |
0.54 |
−24 ± 0.2 |
ZnO leaf 100 |
— |
1.00 |
−13 ± 1.9 |
ZnO leaf 100 + HA |
261 ± 9.4 |
0.35 |
−25 ± 0.7 |
The ζ-potentials of all NP suspensions tested ranged between +2 ± 0.1 mV for ZnO sph NPs to −25 ± 0.7 mV for ZnO leaf NPs (Table 1). In agreement with the findings of Liu et al.,46 we noted minimal change in the negative ζ-potentials of citrate-capped Ag NPs in the presence (−18 ± 0.4 mV) and absence (−19 ± 0.3 mV) of HA. Citrate is generally considered to have a low affinity for the Ag NP surface; therefore, the high concentration of HA employed in this study may have effectively stabilized the citrate-capped Ag NPs by displacing citrate from the NP surface.47 Suwannee River NOM has been shown to improve the colloidal stability of mercaptoundecanoic acid-coated gold NPs in destabilizing Ca2+ and Mg2+ solutions.46 The ζ-potentials of certain NPs became more negative in the presence of HA, as seen with the ZnO sph (+2 ± 0.1 to −24 ± 0.2 mV) and ZnO leaf (−13 ± 1.9 to −25 ± 0.7 mV) NPs (Table 1), which likely increased the electrostatic repulsion between the NPs and negatively charged membranes.36 The negative ζ-potential of the ZnO NPs without HA (−20 ± 1.5 mV; Table 1) and their stable PAA coating provide both electrostatic and steric repulsions, which likely accounted for some of the toxicity differences between spherical ZnO NPs and uncoated ZnO sph- and leaf-shaped NPs noted below.
Natural organic compounds have been shown to both prevent or promote the dissolution of ZnO NPs by blocking the interactions between surface zinc ions (Zn2+) and water molecules or by complexing Zn2+ released into the aqueous phase, respectively.13 Aromatic carboxylic and phenolic functional groups found in HAs are known to have high Zn metal complexation capacities.48 The time-dependent effect of HA on the dissolution of PAA-coated and uncoated ZnO NPs is depicted in Fig. 1. Data are reported as the percentage of free Zn2+, relative to the total Zn metal composition of the ZnO NP stock suspension, released during a 48 h dialysis period. Soluble ZnO NPs were selected (instead of the other types of NPs examined in this study) because of their redox-independent dissolution49 and the reported effects of water chemistry (e.g. pH, ionic strength, and HA) on dissolution.50 E3 medium and E3 medium + HA controls had nominal concentrations of Zn metal (≤9.3 μg L−1). Measurements of ZnO NP dissolution indicate that Zn2+ were liberated into the diluent and that HA, under the experimental conditions used, increased dissolved Zn concentrations over time (Fig. 1). For example, the percentages of free Zn2+ released from ZnO sph (32.8%), ZnO leaf (57.1%), and ZnO (13.9%) NPs in the presence of HA at 48 h were noticeably higher than the amount of Zn metal dissolved from these respective NPs (7.9, 2.0, and 11.4%) without HA addition (Fig. 1). Bian et al.50 reported a significant increase in the dissolution of 4 nm ZnO NPs in the presence of HA (100 mg L−1 initial mass concentration) at high pHs (9 and 11) and a similar non-significant trend at low pHs (1, 3, and 6), and our results support their findings. Furthermore, Jiang et al.49 noted a positive correlation between dissolved Zn and Suwannee River HA concentrations, and suggested that aromatic carbon content played a key role in determining NOM-promoted dissolution of spherical-shaped, (3-aminopropyl)triethoxysilane-coated ZnO NPs suspended in buffered potassium chloride solution. Overall, the above findings indicate that HA affects the physicochemical properties of certain NPs and in our particular set of experiments, promotes enhanced dissolution.
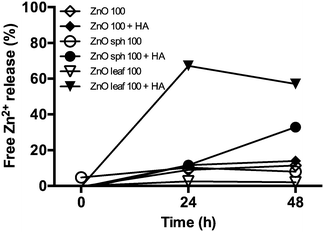 |
| Fig. 1 Time-dependent measurements of ZnO NP dissolution in the absence and presence of 10 mg L−1 Suwannee River HA. Data are presented as percent free Zn2+, relative to E3 medium or HA control, released into diluent from 100 mg L−1 ZnO, ZnO sph, or ZnO leaf NP stock suspensions at 0, 24, and 48 h dialysis. | |
3.2 Effect of humic acid on survival of nanoparticle exposed zebrafish
Ionic metal dissolution from NPs is often associated with adverse biological effects,4 and may also contribute to NP toxicity. Metals are known to cause perturbations of enzyme activity and osmoregulation, increases in oxidative stress, and activation of detoxification processes, resulting in reduced energy allocation to developmental processes.22 The HA itself may also contain adsorbed metals, including Fe, Al, Cu, Mn, Ba, and Zn,51 that cause toxicity via oxidative stress.52 However, 10 mg L−1 HA in E3 medium did not affect survival, hatching success, length, head-tail angle, or spontaneous movement in this study (Fig. 2–6), thus allowing for focus on NP–HA interactions and their biological effects. Zebrafish exposed to Ag NPs, SWCNTs, CdSe NPs, and ZnO NPs both in the absence and presence of HA did not impact survival over the 96 h exposure period and were not significantly different from the E3 medium or HA controls (Fig. 2). We expect that surface functionalization played a role in these non-significant findings as exposure to uncoated ZnO sph and ZnO leaf NPs decreased survival to 56 ± 13% and 30 ± 16%, respectively (Fig. 2). Even with the addition of HA, survival resulting from exposure to both ZnO sph (51 ± 12%) NPs and ZnO leaf (43 ± 10%) NPs were still significantly lower than the HA control (91 ± 1%; Fig. 2). In general, NOM has been shown to increase survival in NP-exposed non-vertebrate aquatic organisms.7,35,36,43,53 However, the addition of NOM in NP-exposed piscine studies has produced mixed results; increased survival,21 no effect on survival,21 and decreased survival20 have all been reported.
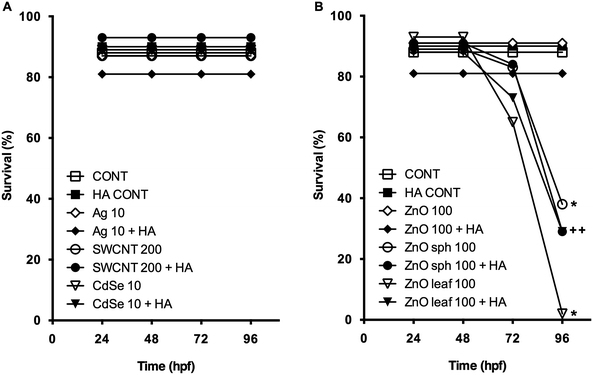 |
| Fig. 2 Survival (%) of zebrafish exposed to (A) 10 mg L−1 Ag NPs, 200 mg L−1 SWCNTs, 10 mg L−1 CdSe NPs, (B) 100 mg L−1 ZnO NPs, 100 mg L−1 ZnO sph NPs, or 100 mg L−1 ZnO leaf NPs in the absence (open symbols) and presence (closed symbols) of 10 mg L−1 HA at 24, 48, 72, and 96 hpf. An asterisk (*) denotes p < 0.05 when compared to E3 medium control. A plus sign (+) denotes p < 0.05 compared to HA control. | |
3.3 Effect of humic acid on hatching success of nanoparticle exposed zebrafish
The most apparent effect of the addition of HA was the reversal of the hatch inhibition in embryos exposed to ZnO sph and ZnO leaf NPs. Previous studies have suggested that ZnO NPs inhibit zebrafish hatch by interfering with the function of the Zn metalloprotease hatching enzyme.25,54 Without HA, 97 ± 1% of E3 medium control embryos had hatched by 72 h, and 100 ± 0% by 96 h, whereas only 13 ± 7% of ZnO sph and 12 ± 11% of ZnO leaf exposed zebrafish had hatched by 96 h (Fig. 3). Hatching success was restored to 98 ± 2% in ZnO sph + HA exposed embryos, and increased to 70 ± 12% in ZnO leaf + HA embryos (Fig. 3). However, HA did not completely reverse the effects of ZnO leaf hatch inhibition; hatching success was improved (70 ± 12%) but remained lower than HA control embryos (100%). Although HA was shown to enhance ZnO NP dissolution, the Zn metal released was bound and not bioavailable. Therefore, inhibition of hatch was likely a NP-specific effect. After hatch, the embryo is directly exposed to NPs, and the beneficial effects of HA may be diminished after the chorion has been breached.
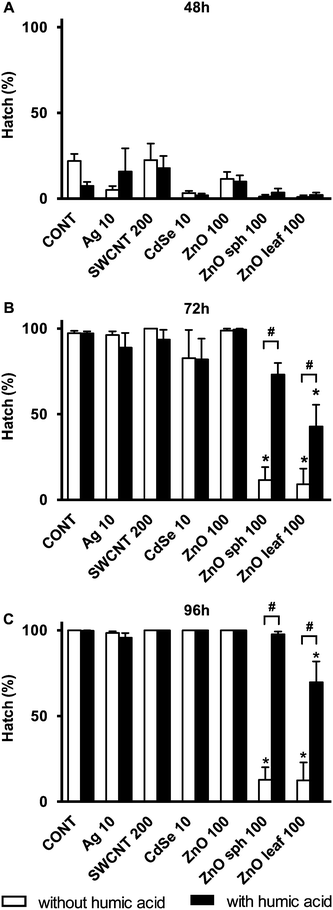 |
| Fig. 3 Hatch (%) of zebrafish exposed to 10 mg L−1 Ag NPs, 200 mg L−1 SWCNTs, 10 mg L−1 CdSe NPs, 100 mg L−1 ZnO NPs, 100 mg L−1 ZnO sph NPs, or 100 mg L−1 ZnO leaf NPs in the absence (white bars) and presence (black bars) of 10 mg L−1 HA at (A) 48, (B) 72, and (C) 96 h. An asterisk (*) denotes p < 0.05 when compared to E3 medium control. A number sign (#) denotes p < 0.05 between NP exposure with and without HA. | |
3.4 Effect of humic acid on length and head-tail angle of nanoparticle exposed zebrafish
Several nanotoxicological studies using zebrafish as a model system have suggested that observed developmental toxicities such as reduced growth, altered axial curvature and impaired movement were NP-specific and not solely attributed to the dissolution of metal-based NPs and the subsequent release of free metal ions.25,54–56 In agreement with Ong et al.,25 the NPs used in this study did not affect the length of zebrafish larvae (Fig. 4). ZnO sph and leaf NPs both slowed the uncurling of embryos at 72 h measuring 147 ± 2° and 145 ± 2°, respectively (Fig. 5); a straightened control larva being ∼152°.33 In all of these cases, the addition of HA ameliorated these effects; head-tail angle of larvae exposed to ZnO sph and leaf NPs + HA were restored to 153 ± 2° and 151 ± 2°, respectively (Fig. 5). The latter effect cannot be explained exclusively by Zn2+ release because ZnO NP + HA treatments contained a higher percentage of Zn metal compared to those without HA addition (Fig. 1). Since ZnO sph and leaf NPs both slowed embryo and larval development slightly in the absence of HA (Fig. 5), we conclude that the NPs themselves likely contributed to the noted hatching delay (Fig. 3) and affected larval straightening, which could hinder movement. However, we ruled out the detrimental effect of NPs and HA on movement; spontaneous movement (Fig. 6) and touch response (100% of larvae responded to touch; data not shown) were not altered. Our results indicate that in natural environments fish embryo development may not be affected by NPs due to the presence of HA.
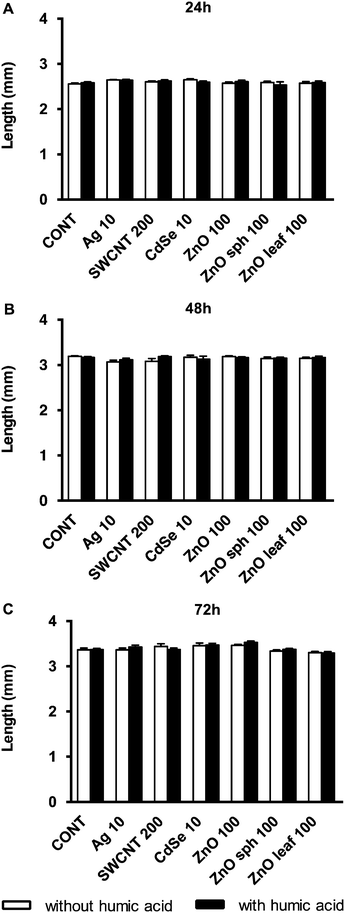 |
| Fig. 4 Length (mm) of zebrafish exposed to 10 mg L−1 Ag NPs, 200 mg L−1 SWCNTs, 10 mg L−1 CdSe NPs, 100 mg L−1 ZnO NPs, 100 mg L−1 ZnO sph NPs, or 100 mg L−1 ZnO leaf NPs in the presence (black bars) and absence (white bars) of 10 mg L−1 HA at (A) 24, (B) 48, and (C) 72 h. | |
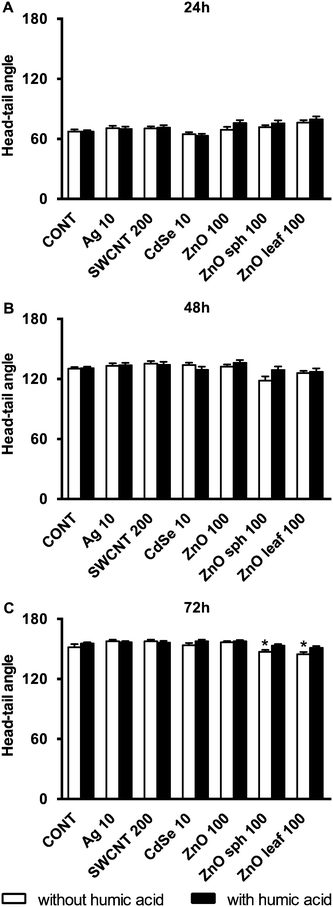 |
| Fig. 5 Head-tail angle of zebrafish exposed to 10 mg L−1 Ag NPs, 200 mg L−1 SWCNTs, 10 mg L−1 CdSe NPs, 100 mg L−1 ZnO NPs, 100 mg L−1 ZnO sph NPs, or 100 mg L−1 ZnO leaf NPs in the presence (black bars) and absence (white bars) of 10 mg L−1 HA at (A) 24, (B) 48, and (C) 72 h. An asterisk (*) denotes p < 0.05 when compared to E3 medium or HA controls. | |
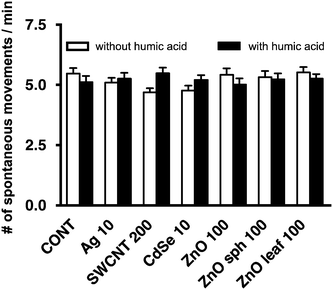 |
| Fig. 6 Spontaneous movements/min of 48 hpf embryos after exposure to 10 mg L−1 Ag NPs, 200 mg L−1 SWCNTs, 10 mg L−1 CdSe NPs, 100 mg L−1 ZnO NPs, 100 mg L−1 ZnO sph NPs, or 100 mg L−1 ZnO leaf NPs in the absence (white bars) and presence (black bars) of 10 mg L−1 HA measured over 10 min intervals (p > 0.05). | |
3.5 Effect of humic acid on protease activity of nanoparticle exposed zebrafish
Zebrafish hatch is dependent on both the movement of the embryo and the release of zinc metalloprotease zebrafish hatching enzymes.57 Inhibition of hatch is likely partially attributed to NP–hatching enzyme interactions.25 Adsorption and coating of NPs by HA could prevent this interaction from occurring, and the different shapes of the ZnO NPs may affect this affinity.21 Ong et al.25 reported a strong correlation (R2 = 0.76) between protease activity and zebrafish hatch. In isolated protease experiments, certain NPs were found to reduce enzymatic activity (Fig. 7). All of the zinc-based NPs, ZnO, ZnO sph, and ZnO leaf reduced protease activity to 16 ± 7%, 39 ± 16% and 31 ± 14% of E3 medium control activity. However, NP-induced enzyme inhibition was not remediated with the addition of HA, and ZnO + HA (18 ± 6%), ZnO sph + HA (32 ± 15%), and ZnO leaf + HA (29 ± 11%) treatments did not change protease activity (Fig. 7). While this finding suggests that enzyme inhibition may not be the main mechanism by which HA is abrogating hatch inhibition, many complex interactions are occurring during in chemico experiments (e.g. adsorption to the chorion, settling of NPs onto the surface of the chorion, interaction with other proteins, etc.), and this may not be fully represented in vitro. Further experiments exploring isolated hatching enzyme and its activity in the presence of NPs and NOM need to be performed.
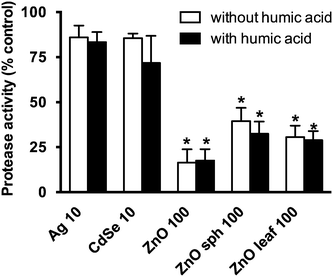 |
| Fig. 7 Protease activity expressed as the percentage of E3 medium or HA control. Zebrafish embryos were exposed to 10 mg L−1 Ag NPs, 10 mg L−1 CdSe NPs, 100 mg L−1 ZnO NPs, 100 mg L−1 ZnO sph NPs, or 100 mg L−1 ZnO leaf NPs in the absence (white bars) and presence (black bars) of 10 mg L−1 HA for 48 h. An asterisk (*) denotes p < 0.05 when compared to E3 medium or HA control. | |
3.6 Effect of humic acid on nanoparticle interaction with zebrafish embryos
Though the chorion may minimize NP–embryo interaction during early developmental stages through adsorption or by acting as a physical barrier to NP entry, it is unknown how NOM may affect the transport of NPs through the pores of the chorion that are ∼170 nm2 in size.58 Nanocapsules (without Nile red) dispersed in dechlorinated tap water (100 mg L−1) were previously reported to be 31.64 ± 0.12 nm in size;32 therefore it is likely that an individual particle or small agglomerates passively traverse chorion pore canals, although active mechanisms cannot be ruled out. To visualize NP–HA interaction with zebrafish embryos, we exposed GFP expressing embryos to NR-NCs and HA for 24 h and subsequently observed the presence or absence of Nile red fluorescence by laser scanning confocal microscopy. Embryos labeled with NR-NCs in the presence and absence of HA showed staining on both the surface of the chorion and on the surface of the developing zebrafish within the chorion (Fig. 8). Felix et al.32 observed similar staining patterns following exposure of wild-type zebrafish embryos to NR-NCs. However, the NR-NCs located on the external larval surface could not be quantitatively distinguished from those that may have been internalized by the animal using in vivo methods. Using inductively coupled plasma-mass spectroscopy, Felix et al.32 also reported a nominal concentration of free Zn metal (0.08 ± 0.5 mg L−1) present in the non-fluorescent PAA nanocapsule stock suspension (10 g L−1). However, mass spectrometry techniques such as laser ablation-inductively coupled mass spectrometry or time-of-flight secondary ion mass spectrometry cannot be employed in the present study to quantify NR-NC interaction with zebrafish embryos because these particles lack a metal-oxide core and the amount of Zn metal would likely be below instrument detection limit at the exposure concentration employed in this study. We also observed GFP fluorescence and no apparent autofluorescence in control embryos exposed to E3 medium (Fig. 8B) and HA (Fig. 8F) alone, as well as co-localization of GFP with lipid droplets59 in the absence (Fig. 8J) and presence (Fig. 8N) of HA, as indicated by double staining patterns with the lipophilic dye, Nile red. Our results clearly demonstrate that the presence of HA does not impair the NPs from passing through the chorion.
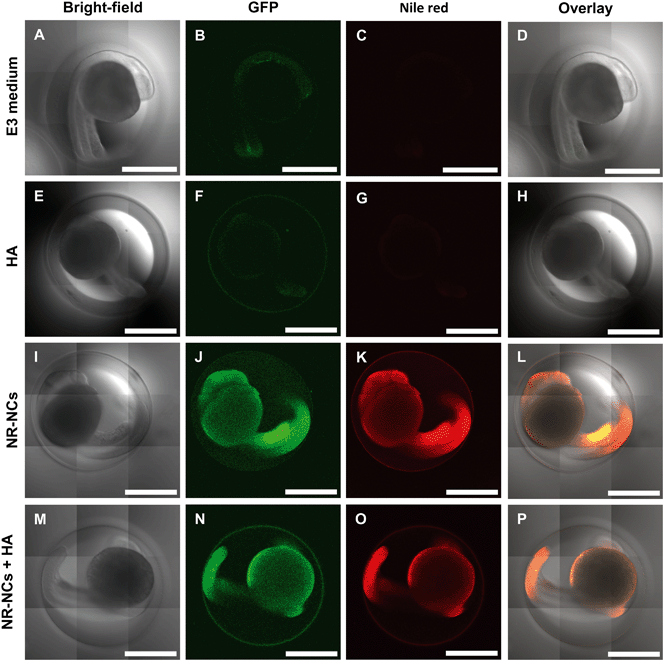 |
| Fig. 8 Bright-field (A, E, I, M), GFP (λ = 488 nm) fluorescence (B, F, J, N), Nile red (λ = 561 nm) fluorescence (C, G, K, O), and overlay (D, H, L, P) laser scanning confocal micrographs of 24 hpf zebrafish. Embryos were exposed to E3 medium (A–D), 10 mg L−1 HA (E–H), 100 mg L−1 NR-NCs (I–L), or NR-NCs in the presence of HA (M–P) for 24 h. Scale bars are 0.5 mm. | |
4 Conclusions
Although this study suggests that HA may diminish some of the negative developmental effects of NPs on embryonic zebrafish by enhancing particle dissolution, ultimately, the presence of HA did not abrogate the lethality caused by uncoated ZnO NP exposure. The presence of HA evidently affects NP toxicity; therefore, the need for nanotoxicity testing under environmentally realistic conditions is strengthened. Laboratory studies using pristine water to evaluate NP effects may not be representative of natural environments. We recommend using natural waters obtained from local sources containing NOM as well as synthetic media to disperse NPs in future aquatic nanotoxicological studies. Predictive water quality models will have to include NOM as it affects the physicochemical characteristics and behavior of NPs. Furthermore, analytical techniques designed to quantify fluorescent polymeric NP uptake in vivo are needed. The stabilization of NPs in aquatic systems due to NOM may also be of concern due to their increased mobility.60 Different aquatic sources of NOM can result in differential toxicity7,40,41,53 and different concentrations of HA can affect aggregation state and toxicity.35 Factors such as pH,41 ionic strength,61 salts,62 and sunlight63–65 can also play a role in the degree of toxicity and effects resulting from a combination of these factors will certainly be dynamic and complex. Studying the effects of NPs under a range of relevant environmental conditions is essential for accurate projection of the potential aquatic toxicity of NPs, and isolating and testing each component of natural waters is the first step towards achieving these goals.
Acknowledgements
This work was supported by a grant from the National Research Council of Canada-Natural Sciences and Engineering Research Council of Canada (NSERC)-Business Development Bank of Canada Nanotechnology Initiative and Environment Canada (Grant # NNBPJ380151-08), an Alberta Innovates Technology Futures (AITF) nanoWorks collaborative research agreement (CRA Reference # 200900065), and an NSERC Strategic project grant (Grant # 463674-14) to GGG. KJO and JDE were supported by NSERC Vanier Canada Graduate Scholarship and AITF Scholarship. The NSERC Postgraduate Scholarship-Doctoral and Queen Elizabeth II Graduate Scholarship supported LCF. We would like to extend our gratitude to Dr. Darren Anderson of Vive Crop Protection Inc. for providing the ZnO NPs and NR-NCs, Dr. Yadienka Martinez-Rubi and Dr. Benoit Simard from the NRC for donating the SWCNTs, as well as Dr. Gregory Pyle and Sarah Bogart from the University of Lethbridge for AAS analysis. Biological Sciences Animal Services are thanked for zebrafish care. This research was conducted under animal care protocol AUP0000001 at the University of Alberta.
References
- F. Gottschalk and B. Nowack, J. Environ. Monit., 2011, 13, 1145–1155 RSC.
- L. Reijnders, Polym. Degrad. Stab., 2009, 94, 873–876 CrossRef CAS.
- T. M. Benn and P. Westerhoff, Environ. Sci. Technol., 2008, 42, 4133–4139 CrossRef CAS PubMed.
- T. M. Scown, R. van Aerle and C. R. Tyler, Crit. Rev. Toxicol., 2010, 40, 653–670 CrossRef CAS PubMed.
- S. Wagner, A. Gondikas, E. Neubauer, T. Hofmann and F. von der Kammer, Angew. Chem., Int. Ed., 2014, 53, 12398–12419 CAS.
- M. R. Wiesner, G. V. Lowry, K. L. Jones, M. F. Hochella Jr., R. T. Giulio, E. Casman and E. S. Bernhardt, Environ. Sci. Technol., 2009, 43, 6458–6462 CrossRef CAS PubMed.
- J. Gao, S. Youn, A. Hovsepyan, V. L. Llaneza, Y. Wang, G. Bitton and J.-C. J. Bonzongo, Environ. Sci. Technol., 2009, 43, 3322–3328 CrossRef CAS PubMed.
- L. P. Lukhele, B. B. Mamba, N. Musee and V. Wepener, J. Nanomater., 2015, 2015, 1–19 CrossRef.
- N. Musee, M. Thwala and N. Nota, J. Environ. Monit., 2011, 13, 1164–1183 RSC.
- S. K. Lee, D. Freitag, C. Steinberg, A. Kettrup and Y. H. Kim, Water Res., 1993, 27, 199–204 CrossRef CAS.
- N. R. Bury, F. Galvez and C. M. Wood, Environ. Toxicol. Chem., 1999, 18, 56–62 CrossRef CAS.
- T. Meinelt, R. C. Playle, M. Pietrock, B. K. Burnison, A. Wienke and C. E. W. Steinberg, Aquat. Toxicol., 2001, 54, 205–215 CrossRef CAS PubMed.
- A.-J. Miao, X.-Y. Zhang, Z. Luo, C.-H. Chen, W.-C. Chin, P. H. Santschi and A. Quigg, Environ. Toxicol. Chem., 2010, 29, 2814–2822 CrossRef CAS PubMed.
- P. MacCarthy, Soil Sci., 2001, 166, 738–751 CrossRef CAS.
- N. Meems, C. E. W. Steinberg and C. Wiegand, Sci. Total Environ., 2004, 319, 123–136 CrossRef CAS PubMed.
- S. Höss, M. Bergtold, M. Haitzer, W. Traunspurger and C. E. W. Steinberg, Freshwater Biol., 2001, 46, 1–10 CrossRef.
- S. Pflugmacher, C. Pietsch, W. Rieger and C. E. W. Steinberg, Sci. Total Environ., 2006, 357, 169–175 CrossRef CAS PubMed.
- C. Young and R. von Wandruszka, Geochem. Trans., 2001, 2, 16 CrossRef.
- S. Ghosh, H. Mashayekhi, P. Bhowmik and B. Xing, Langmuir, 2010, 26, 873–879 CrossRef CAS PubMed.
- S. P. Yang, O. Bar-Ilan, R. E. Peterson, W. Heideman, R. J. Hamers and J. A. Pedersen, Environ. Sci. Technol., 2013, 47, 4718–4725 CrossRef CAS PubMed.
- K.-T. Kim, M.-H. Jang, J.-Y. Kim, B. Xing, R. L. Tanguay, B.-G. Lee and S. D. Kim, Sci. Total Environ., 2012, 426, 423–429 CrossRef CAS PubMed.
- B. Jezierska, K. Ługowska and M. Witeska, Fish Physiol. Biochem., 2009, 35, 625–640 CrossRef CAS PubMed.
- A. J. Hill, H. Teraoka, W. Heideman and R. E. Peterson, Toxicol. Sci., 2005, 86, 6–19 CrossRef CAS PubMed.
- L. C. Felix, J. D. Ede, D. A. Snell, T. M. Oliveira, Y. Martinez-Rubi, B. Simard, J. H. T. Luong and G. G. Goss, Carbon, 2016, 104, 78–89 CrossRef CAS.
- K. J. Ong, X. Zhao, M. E. Thistle, T. J. MacCormack, R. J. Clark, G. Ma, Y. Martinez-Rubi, B. Simard, J. S. C. Loo, J. G. C. Veinot and G. G. Goss, Nanotoxicology, 2014, 8, 295–304 CrossRef CAS PubMed.
- A. G. Schultz, K. J. Ong, T. MacCormack, G. Ma, J. G. C. Veinot and G. G. Goss, Environ. Sci. Technol., 2012, 46, 10295–10301 CrossRef CAS PubMed.
- K. S. Kim, G. Cota-Sanchez, C. T. Kingston, M. Imris, B. Simard and G. Soucy, J. Phys. D: Appl. Phys., 2007, 40, 2375–2387 CrossRef CAS.
- K. S. Kim, M. Imris, A. Shahverdi, Y. Alinejad and G. Soucy, J. Phys. Chem. C, 2009, 113, 4340–4348 CAS.
- B. K. Price, J. R. Lomeda and J. M. Tour, Chem. Mater., 2009, 21, 3917–3923 CrossRef CAS.
- P. Zhong, Y. Yu, J. Wu, Y. Lai, B. Chen, Z. Long and C. Liang, Talanta, 2006, 70, 902–906 CrossRef CAS PubMed.
- B. C. Heng, X. Zhao, E. C. Tan, N. Khamis, A. Assodani, S. Xiong, C. Ruedl, K. W. Ng and J. S.-C. Loo, Arch. Toxicol., 2011, 85, 1517–1528 CrossRef CAS PubMed.
- L. C. Felix, V. A. Ortega, J. D. Ede and G. G. Goss, Environ. Sci. Technol., 2013, 47, 6589–6596 CAS.
- C. B. Kimmel, W. W. Ballard, S. R. Kimmel, B. Ullmann and T. F. Schilling, Dev. Dyn., 1995, 203, 253–310 CrossRef CAS PubMed.
- S. Ali, H. G. J. van Mil and M. K. Richardson, PLoS One, 2011, 6, e21076 CAS.
- J. Gao, K. Powers, Y. Wang, H. Zhou, S. M. Roberts, B. M. Moudgil, B. Koopman and D. S. Barber, Chemosphere, 2012, 89, 96–101 CrossRef CAS PubMed.
- J. Chen, Z. Xiu, G. V. Lowry and P. J. J. Alvarez, Water Res., 2011, 45, 1995–2001 CrossRef CAS PubMed.
- Z. Li, K. Greden, P. J. J. Alvarez, K. B. Gregory and G. V. Lowry, Environ. Sci. Technol., 2010, 44, 3462–3467 CrossRef CAS PubMed.
- B. D. Johnston, T. M. Scown, J. Moger, S. A. Cumberland, M. Baalousha, K. Linge, R. van Aerle, K. Jarvis, J. R. Lead and C. R. Tyler, Environ. Sci. Technol., 2010, 44, 1144–1151 CrossRef CAS PubMed.
- A. Lapresta-Fernández, A. Fernández and J. Blasco, TrAC, Trends Anal. Chem., 2012, 32, 40–59 CrossRef.
- A. J. Edgington, A. P. Roberts, L. M. Taylor, M. M. Alloy, J. Reppert, A. M. Rao, J. Mao and S. J. Klaine, Environ. Toxicol. Chem., 2010, 29, 2511–2518 CrossRef CAS PubMed.
- H. Hyung and J.-H. Kim, Environ. Sci. Technol., 2008, 42, 4416–4421 CrossRef CAS PubMed.
- M. Baalousha, A. Manciulea, S. Cumberland, K. Kendell and J. R. Lead, Environ. Toxicol. Chem., 2008, 27, 1875–1882 CrossRef CAS PubMed.
- J. Fabrega, S. R. Fawcett, J. C. Renshaw and J. R. Lead, Environ. Sci. Technol., 2009, 43, 7285–7290 CrossRef CAS PubMed.
- S. Diegoli, A. L. Manciulea, S. Begum, I. P. Jones, J. R. Lead and J. A. Preece, Sci. Total Environ., 2008, 402, 51–61 CrossRef CAS PubMed.
- P. Christian, F. von der Kammer, M. Baalousha and T. Hofmann, Ecotoxicology, 2008, 17, 326–343 CrossRef CAS PubMed.
- J. Liu, S. Legros, F. von der Kammer and T. Hofmann, Environ. Sci. Technol., 2013, 47, 4113–4120 CrossRef CAS PubMed.
- I. L. Gunsolus, M. P. S. Mousavi, K. Hussein, P. Bühlmann and C. L. Haynes, Environ. Sci. Technol., 2015, 49(13), 8078–8086 CrossRef CAS PubMed.
- P. Boguta and Z. Sokołowska, PLoS One, 2016, 11(4), e0153626 Search PubMed.
- C. Jiang, G. R. Aiken and H. Hsu-Kim, Environ. Sci. Technol., 2015, 49, 11476–11484 CrossRef CAS PubMed.
- S.-W. Bian, I. A. Mudunkotuwa, T. Rupasinghe and V. H. Grassian, Langmuir, 2011, 27, 6059–6068 CrossRef CAS PubMed.
- K. M. Kuhn, E. Neubauer, T. Hofmann, F. von der Kammer, G. R. Aiken and P. A. Maurice, Environ. Eng. Sci., 2015, 32, 54–65 CrossRef CAS.
- C. E. W. Steinberg, S. Kamara, V. Y. Prokhotskaya, L. Manusadžianas, T. A. Karasyova, M. A. Timofeyev, Z. Jie, A. Paul, T. Meinelt, V. F. Farjalla, A. Y. O. Matsuo, B. K. Burnison and R. Menzel, Freshwater Biol., 2006, 51, 1189–1210 CrossRef CAS.
- S. Lee, K. Kim, H. K. Shon, S. D. Kim and J. Cho, J. Nanopart. Res., 2011, 13, 3051–3061 CrossRef CAS.
- W. Bai, Z. Zhang, W. Tian, X. He, Y. Ma, Y. Zhao and Z. Chai, J. Nanopart. Res., 2010, 12, 1645–1654 CrossRef CAS.
- J. Hua, M. G. Vijver, M. K. Richardson, F. Ahmad and W. J. G. M. Peijnenburg, Environ. Toxicol. Chem., 2014, 33(12), 2859–2868 CrossRef CAS PubMed.
- T. C. King-Heiden, P. N. Wiecinski, A. N. Mangham, K. M. Metz, D. Nesbit, J. A. Pedersen, R. J. Hamers, W. Heideman and R. E. Peterson, Environ. Sci. Technol., 2009, 43, 1605–1611 CrossRef CAS PubMed.
- K. Sano, K. Inohaya, M. Kawaguchi, N. Yoshizaki, I. Iuchi and S. Yasumasu, FEBS J., 2008, 275, 5934–5946 CrossRef CAS PubMed.
- J. Cheng, E. Flahaut and S. H. Cheng, Environ. Toxicol. Chem., 2007, 26, 708–716 CrossRef CAS PubMed.
- S. Cermelli, Y. Guo, S. P. Gross and M. A. Welte, Curr. Biol., 2006, 16, 1783–1795 CrossRef CAS PubMed.
- R. L. Johnson, G. O. Johnson, J. T. Nurmi and P. G. Tratnyek, Environ. Sci. Technol., 2009, 43, 5455–5460 CrossRef CAS PubMed.
- L. Truong, T. Zaikova, E. K. Richman, J. E. Hutchison and R. L. Tanguay, Nanotoxicology, 2012, 6, 691–699 CrossRef CAS PubMed.
- K. L. Chen and M. Elimelech, J. Colloid Interface Sci., 2007, 309, 126–134 CrossRef CAS PubMed.
- O. Bar-Ilan, K. M. Louis, S. P. Yang, J. A. Pedersen, R. J. Hamers, R. E. Peterson and W. Heideman, Nanotoxicology, 2012, 6, 670–679 CrossRef CAS PubMed.
- O. Bar-Ilan, C. C. Chuang, D. J. Schwahn, S. Yang, S. Joshi, J. A. Pedersen, R. J. Hamers, R. E. Peterson and W. Heideman, Environ. Sci. Technol., 2013, 47, 4726–4733 CrossRef CAS PubMed.
- H. Ma, A. Brennan and S. A. Diamond, Environ. Toxicol. Chem., 2012, 31, 1621–1629 CrossRef CAS PubMed.
Footnote |
† These authors contributed equally to the work. |
|
This journal is © The Royal Society of Chemistry 2017 |