Sewage sludge treated with metal nanomaterials inhibits earthworm reproduction more strongly than sludge treated with metal metals in bulk/salt forms†
Received
25th July 2016
, Accepted 13th December 2016
First published on 14th December 2016
Abstract
Earthworms were exposed to soils amended with sewage sludges from a wastewater treatment plant (WWTP) treated with nanomaterials (ENMs) or metal/ionic salts. Sewage sludges were generated with either no metal added to the WWTP influent (control), ionic ZnO, AgNO3 and bulk (micron sized) TiO2 added (ionic metal-treated) or ZnO, Ag and TiO2 ENMs added (ENM-treated). A sandy-loam soil was amended with the treated sewage sludge and aged in outdoor lysimeters for six months. Earthworms were exposed to the aged mixtures and a dilution of the mixtures (using control soil–sludge mix). Separate earthworm exposures to as-synthesized ENM and ionic metals salts (Zn/Ag singly) were carried out in the same soil. Earthworm reproduction was depressed by 90% in the high-metal ENM treatment and by 22–27% in the ionic metal and low-metal ENM soil–sludge treatments. Based on total metal concentrations in the soil–sludges the as-synthesised metal salt and ENM exposures predicted Zn was driving observed toxicity in the soil–sludge more than Ag. Earthworms from the high-metal ENM treatment accumulated significantly more Ag than other treatments whereas total Zn concentrations in the earthworms were within the range for earthworm Zn regulation for all treatments. This study suggests that current Zn limits set to provide protection against ionic metal forms may not protect soil biota where metals are input to WWTP in the ENM form.
Environmental significance
Engineered nanomaterials (ENMs) are most likely to enter terrestrial ecosystems through the application of sludge from waste water treatment plants (WWTP) to agricultural soils. However very little is known about how transformed ENMs will behave in the environment and their subsequent bioavailability and toxicity to soil biota. Here we show that sewage sludge treated with ENMs applied to soil had a greater effect on earthworm survival and reproduction than the same sludge treated with bulk metal forms, even though the solid-phase speciation in the ENM- and bulk metal-treated sludges were essentially identical. This novel finding suggests that the current zinc limits set to provide protection against bulk metal forms may not protect soil biota where metals are in ENM forms.
|
Introduction
The growing use of engineered nanomaterials (ENMs) in numerous consumer products has led to an increase in their environmental inputs. ZnO, Ag and TiO2 are among the most commonly used ENMs in consumer products such as cosmetics, personal care products, paints and antimicrobial treatments. A major transfer of ENMs from the point of use will be through sewer systems into wastewater treatment plants (WWTP). Within WWTP, ENMs have been shown to largely partition to sludge1–3 the majority of which is subsequently applied to agricultural land as a fertiliser in many regions including the U.S. and the E.U.4 Such disposal of sludge may result in the release of ENMs, or their transformation products, to soil ecosystems where they may cause toxicity to soil biota and/or enter food webs. As the environmental risk is yet to be fully understood, studies that simulate relevant exposure pathways relating to sludge application to land are clearly necessary to assess any potential impacts to soil biota of the incorporation of ENMs or their transformation products into sludge subsequently applied to land.
As-synthesised/as-manufactured nanoparticulate forms of ZnO and Ag have been shown to affect survival and life history traits of soil invertebrates, such as reproduction and growth. Ag ENMs often show effects at lower concentrations than Zn ENMs.5–9 For ZnO and Ag ENMs, dissolution to their ionic forms in the soil porewater often has been related to observed toxicity,6–8 although this is not always the case.9 In contrast, TiO2 ENMs show extremely low solubility10,11 so dissolution products are unlikely to play a role in observed effects.11,12 Indeed, compared to ZnO and Ag ENMs, TiO2 ENMs have shown relatively low toxicity to soil organisms; higher concentrations of TiO2 ENMs are needed in the soil to cause mortality or reproduction effects compared to Zn or Ag.5 The bioavailability of ENMs to soil invertebrates has also been assessed by measuring the whole body metal concentrations of earthworms. In some cases tissue Ag and Zn tissue concentrations in ENM-exposed earthworms may reach concentrations which would normally result in mortality in equivalent ionic exposures but without the expected mortality effect occuring.7,8 This suggests that the form in which soil invertebrates are exposed to metals (either as ENMs, ionic metal or a mixture of both) exerts important controls on metal handling and toxicity.
Previous soil invertebrate toxicity studies have largely considered only exposure to as-synthesised ENMs and not environmentally realistic scenarios where ENMs may have been transformed (for example in WWTP) into physicochemically distinct end products. In the WWT process Ag ENMs are completely sulfidised,1,13–15 while ZnO ENMs have been shown to become sulfidised, phosphatised or associated with FeO(OH).1 In contrast, TiO2 is expected to be much less likely to transform chemically, although surface properties and agglomeration/aggregation state may be altered. The only studies we are aware of investigating ENM toxicity after transformation by the WWT process found that ENM-containing sludge applied to soil inhibited nodulation in the model legume Medicago truncatula which could be linked with the down-regulation of genes involved in general stress responses, metal homeostasis, nodulation and nitrogen metabolism.16,17 Some adverse ecosystem responses to Ag ENMs in biosolids were found when applied to mesocosms; there were significant changes to microorganism abundance, function and community composition.18 Other studies that investigated effects of ZnO ENMs in sewage sludge applied to soils found only slight effects on earthworm cocoon production and reduction in plant biomass (wheat, radish and vetch).19,20 These studies concluded that ZnO ENMs in sewage sludge pose a low environmental risk, although mostly in the latter studies as-synthesised ENMs were directly added to sludge rather than passed through the full WWT process.19,20 Thus there is still very little known about how transformed particles will behave in the environment and their subsequent bioavailability and toxicity to soil biota.
Earthworms are keystone species in terrestrial environments, integral to organic matter turnover in the soil, and so are a key group for which to assess the effects of amending soils with treated sludges with ENM inputs. In this study, earthworms were exposed to soils amended with sewage sludge generated by a pilot full WWTP.1 Sewage sludges were generated where either no metal was added to the WWTP influent (control); non-ENM metal salts ZnSO4, AgNO3 and bulk metal (micron-sized) TiO2 were added to the influent (ionic metal treatment), or ZnO, Ag and TiO2 ENMs were added to the influent (ENM treatment).1,16,17 The bioavailability and toxicity of the metals in the different soil–sludge treatments to earthworms were compared by considering the metal concentration in the soil and the total body metal concentrations in the earthworms, linking to effects on the key life history traits of growth and reproduction. Toxicity data from as-synthesised Ag/ZnO ENM and Ag/Zn ionic metal salt single exposures were used to predict effects of the total Zn and Ag concentrations in the soil and the total Zn and Ag concentrations in the earthworms from the soil–sludge exposures. Given that TiO2 is known to have little or no toxicity for earthworms at the concentrations in the soil–sludge treatment5,11,12 and are unlikely to transform, Ti was not considered as a toxicant in this study.
Materials and methods
Soil–sludge mixtures for toxicity tests
The sludge generation and soil–sludge mixtures are described in detail in Ma et al. 2014 (ref. 1) and Judy et al. 2015 (ref. 16) respectively. In brief, a sandy loam soil (Woburn, U.K.) was amended with sewage sludge derived from spiking WWTP influent with either (1) ZnO (30 nm uncoated described previously1), Ag (50 nm stabilized with a 55 kDa average molecular weight polyvinylpyrrolidone (PVP) previously fully described21) and TiO2 (27 ± 7.5 nm, Sigma Aldrich, (Fig. S1, ESI†)) ENMs (ENM sewage sludge), (2) ZnSO4, AgNO3 and micron-sized TiO2 (ionic/bulk metal sewage sludge) or with (3) no metals (control). The intended concentration for Zn in the sludge was 2800 mg Zn kg−1 dry mass, based on the current U.S. cumulative pollutant loading limit for Zn in soils amended with biosolids (2800 kg Zn ha−1).22 Ag and Ti loadings were set to give intended sludge concentrations of 100 mg Ag kg−1 and 2400 mg Ti kg−1 (dry mass), respectively, based on percentiles (98th) of concentration from the U.S. targeted national sewage sludge survey.16 A total of 40 kg of dry sludge (160 kg of wet sludge at 25 weight% solids) were produced from each plant1 (ENM, ionic metal and control) to be used in plant studies16,17,23 and in this current earthworm study. Sludges were air-dried at Rothamsted Research (UK) and mixed with the sandy loam soil in a ratio of 0.58
:
0.42 soil
:
sludge, to give a target Zn concentration of 1400 mg Zn kg−1 dry soil in the ionic metal treatment.16 The ratio of sludge to soil was based on the current U. S. EPA cumulative pollutant loading limit for Zn and was selected following the guidelines within Guide to the Biosolids Risk Assessments for the EPA, CFR 40 Part 503, which results in a 1
:
1 soil
:
sewage sludge ratio in the top 15 cm of soil following 10 years of application at the maximum allowable concentration of Zn in sludge.16 The soil–sludge mixtures were aged in freely-draining outdoor lysimeters for six months23 to create a set of ‘aged’ soil–sludge mixtures. Earthworms were exposed to five aged soil–sludge treatments; three of the treatments were the 0.58
:
0.42 soil
:
sludge mixture treatments: (1) control soil–sludge (no metal addition) (2) high-metal ENM soil–sludge, (3) high-metal ionic metal soil–sludge and the two other treatments were the high-metal ENM or ionic metal soil–sludge treatments mixed with control soil–sludge in a 1
:
1 ratio giving a (4) low-metal ENM soil–sludge and (5) a low-metal ionic metal soil–sludge (Fig. S2, ESI†). To confirm that the earthworm reproduction was above the minimum number stipulated by OECD guidelines (>30 juveniles), a soil control (Woburn sandy loam soil) without any sludge amendment was also included as a fully replicated test treatment.
Experimental design and toxicity test procedure
The soil–sludge mixtures were distributed in four replicate containers each containing 300 g dry weight of the soil–sludge mix. There were also eight soil controls, each containing 550 g dry weight giving a comparable volume of soil to the soil–sludge mixtures due to differences in the bulk densities of the test media. All soils were wet to 50% of their respective water holding capacities (Table 1) using de-ionised water and left for ten days before the organisms were introduced. Eisenia fetida were initially obtained from a commercial source (Blades Biological, Kent, UK) and maintained in culture soil in a controlled temperature room at 20 ± 1 °C in a 12:12 hour light:dark cycle.8 The toxicity test procedure followed the OECD guideline 222 (earthworm reproduction test (Eisenia fetida/andrei)). Groups of ten fully-clitellated earthworms (average weight 10 worms = 3.41 ± 0.2 g, mean ± SD, n = 28) were rinsed, excess moisture removed with paper towel and weighed as a batch before being added to each replicate container. Horse manure (10 g dry weight), wetted to 80% of its water holding capacity, was added to the soil-only control treatments as food.24 No food was added to the soil–sludge treatments as the sludge provided a food source for the earthworms that also allowed for oral exposure.25 The earthworm exposure containers were kept in a controlled temperature room at 20 ± 1 °C under a 12:12 hour light:dark cycle. After 14 and 28 days of incubation, earthworm survival and batch weight were measured. Surviving adult earthworms were removed from the test containers after 28 days and three earthworms from each replicate were rinsed to remove adhered soil and then kept individually on clean filter paper for 24 hours to allow them to purge their gut contents.7,8 This ensured that minimal soil was left in the earthworm prior to tissue Ag and Zn analysis. The soil–sludge mixtures were the incubated for a further 28 days to allow juveniles to hatch from laid cocoons. The number of juveniles was counted as previously described.8
Table 1 Total soil Ag, Zn and Ti concentrations, pH values and dissolved organic carbon concentration in porewaters and the water holding capacity for each of the soil–sludge mixtures and the sandy loam control soil (Woburn)a
Treatment |
Total Ag (mg Ag kg−1 dry mass) |
Total Zn (mg Zn kg−1 dry mass) |
Total Tib (mg Ti kg−1 dry mass) |
Porewater pH |
Porewater dissolved organic carbon (μg ml−1) |
Water holding capacity (ml per 100 g) |
Each value represents mean ± one standard deviation.
Data from Judy et al. 2015.16 n.d. means that the measurements were not determined.
ENM = engineered nanomaterials.
|
Control |
2.84 ± 0.35 |
321.5 ± 7.19 |
1180 ± 32.7 |
7.10 ± 0.03 |
283 ± 24.7 |
94 |
High metal ionic metal |
111 ± 7.75 |
1600 ± 52.3 |
2365 ± 61.8 |
7.02 ± 0.04 |
304 ± 11.5 |
92 |
High metal ENMc |
94.3 ± 4.77 |
1360 ± 64.8 |
2467 ± 181 |
7.06 ± 0.06 |
299 ± 6.83 |
92 |
Low metal ionic metal |
71.2 ± 2.21 |
985 ± 34 |
n.d. |
7.30 ± 0.06 |
272 ± 1.5 |
93 |
Low metal ENM |
51.6 ± 2.42 |
853 ± 35.7 |
n.d. |
7.09 ± 0.08 |
314 ± 15 |
93 |
Soil control |
0.09 ± 0.02 |
39.2 ± 0.71 |
n.d. |
7.31 ± 0.08 |
119 ± 11.1 |
32 |
In order to compare the toxicity observed in the ENM and ionic metal soil–sludge mixtures, single compound earthworm exposures (i.e. separate exposure were set up for each compound so they were not added as mixture) to as-synthesised ZnO ENM (30 nm uncoated)7 and PVP-coated Ag EMM as well as Zn (Zn(NO3)2) and Ag (AgNO3) salts (Sigma Aldrich, UK) were set up and run using the same procedures as for the soil–sludge exposures (i.e. 28 days survival test and 56 day reproduction test). The same sandy loam (Woburn) soil was spiked with the ENMs or salt, either Zn (100, 225, 500, 1100, 2200 mg Zn kg−1) or Ag (9, 22.5, 56.3, 141, 352, 880, 2200 mg Ag kg−1), in triplicate according to the protocol previously described.8 Spiked soils were wet to 50% of the water holding capacity (Table 1) and after one week ten adult earthworms were added to each test replicate. The toxicity test set up and duration followed the same as for the soil–sludge experiments above and previously described test protocols.7,8 Three surviving adult earthworms were prepared and stored for tissue Zn or Ag analysis in the same manner as for the soil–sludge treatments. It was not possible to carry out these as-synthesised exposures in the control soil–sludge due to the limited amount that could be produced by the pilot WWTP.
Soil porewater extraction
Soil porewater has been identified as an uptake route for ionic metal in soils.26–28 To get a better measure of metal reactivity in the soil, the soil porewater was extracted by centrifugation from each replicate of the soil–sludge mixtures at the end of the exposure period (56 days), before the juveniles were counted. Two 20 g (25 g from the soil control) (dry weight equivalent) soil samples, for separate Zn and Ag analysis, were collected from each of the treatment replicates, saturated to 140% of the water holding capacity of the soil–sludge mixture and equilibrated overnight before porewater was extracted following the extraction protocol described in Whitley et al. 2013 but with two amendments to the protocol.29 The soil sample extracted for Ag was filtered through glass wool and ultra-filters that were pre-soaked in a 0.1 M CuSO4 solution to minimise Ag ion adsorption and losses.8,30 The samples were centrifuging at 4000g for 1. 5 hours (J2-HC, Beckman Coulter, California, USA) to achieve maximum porewater extraction from the soil. A total of 5 ml of the extracted porewater was placed in a 10 kD ultra-filtration device (Amicon Ultra-15 Filters, Millipore, Ireland) and centrifuged for 1.5 hours at 4000g.8 The extracted porewater and ultra-filtered porewater from each replicate were analysed for Ag or Zn using ICP-OES and pH measured (Sartorius Professional Meter PP-25, Sartorius AG, Goettingen, Germany; combination pH probe, filled with 3M KCl).
Chemical analysis
Approximately 0.75 g of air-dried soil and soil–sludge mixtures or 0.5 g of freeze-dried whole earthworm were refluxed with a 3
:
1 mixture of hydrochloric and nitric acids (Merck, ‘Aristar’ grade) at 140 °C for 2.5 h. After digestion the solutions were allowed to cool and then filtered using Whatman number 540 (12.5 cm diameter) filter papers that were pre-soaked with a 0.1 M CuSO4 solution (Sigma-Aldrich, ‘purum’ grade). Digests were made up to a final volume of 50 ml with 0.5% v/v nitric acid and stored at 4 °C prior to analysis for either Ag or Al and Zn. A 1 ml aliquot of porewater was digested with a 3
:
1 mixture of hydrochloric and nitric acids (Merck, ‘Aristar’) using closed Teflon vessels in a microwave digestion system (CEM Corporation, MARSXpress). The digests were heated to 180 °C over a period of 30 minutes and then held at this temperature for a further 30 minutes. Digests were allowed to cool and then made up to a final volume of 50 ml with 1% v/v hydrochloric acid. The soil, porewater and earthworm digests were analysed by inductively coupled plasma mass spectrometry (ICPMS) using a Perkin Elmer Nexion 300D ICPMS instrument. The details of the procedures for checking the efficiency of the digestions, digest dilutions and instrument calibration are in the ESI.† For the primary element of interest, Ag, the ICPMS instrument detection limit was 0.14 μg l−1 (mean blank + 3σ reagent blank, n = 10) and the instrument method had a precision of 1.4% (CoV, at 5 μg l−1, n = 10).
Total metal concentrations in the earthworms were corrected, if necessary, for metal due to soil residues remaining in the gut following depuration. This was done using the total Al concentrations in the soils and earthworms. Aluminium was used to correct as it is naturally present at readily detectable concentrations in the soil and largely present in non-bioavailable forms, thus the concentrations in the worms could be attributed to residual soil present in the gut rather than to uptake into the tissues.
The expression used for correction was
| {M}worm,corr = {M}worm − m × {AL}worm | (1) |
where {M}
worm and {Al}
worm are the measured metal and Al concentrations in the worm and {M}
worm,corr is the corrected tissue concentration. The term
m is the slope of the linear regression of the measured worm metal against the measured worm Al. Separate regressions were done for body burden concentrations of worms exposed to each soil–sludge mixture. Significant relationships (regression
p < 0.05) were found for Al and Ag or Zn concentrations in worms exposed to either the ionic metal or ENM-treated sludges, so corrections were applied to the total Ag and Zn concentration in the earthworms from these exposures.
Data analysis
Survival, weight change and reproduction were first checked for normal variance structure using the Anderson–Darling normality test and log transformed if required. Comparisons of survival, reproduction, and weight change, total Ag and Zn concentrations in the earthworms, total and ultra-filtered metal concentrations in the porewaters across all the treatments were carried out in Minitab 16 using analysis of variance (ANOVA). Where significant differences were found, the Tukey test was used to identify the pattern of significant differences among treatments. Total and ultra-filtered concentrations for each treatment were also compared using an unstacked ANOVA.
To estimate response parameters for the as-synthesised ENMs and metal salts earthworm exposures, data for reproduction (juvenile production rate) was used to fit a three-parameter log-logistic model (eqn (2)) to obtain estimates for the EC50 values based on total metal in the soil and total metal concentrations in the earthworms. Models were fitted in the form:
| y = ymax/(1 + (cc/EC50)exp(b)) | (2) |
where
ymax is the upper asymptote, EC
50 is the concentration (soil/body) resulting in a 50% effect on the measured endpoint (EC
50) and
b the slope parameter. Model fits to derive parameters with associated standard errors were completed using SigmaPlot. EC
25 and EC
90 values were also estimated from the dose response curves.
Results
Test validation
The earthworms in the sandy loam soil control produced more than the minimum 30 juveniles/individuals (39 ± 10 juveniles; mean ± SD; n = 6) thus validating the test procedure.24 The earthworms in the control soil–sludge treatment both gained more weight, 29 ± 11% weigh increase compared to a 4.5 ± 4.9% weight loss in the soil control and produced 2.5 times more juveniles (97 ± 14.6 juveniles, n = 4) than the earthworms in the soil control over the test (Table 2). This improved performance of the sludge-exposed earthworms is likely to be related to the superior quality of food in the organic-rich sewage sludge compared to the soil control. Hence to identify adverse effects of the sludge treated with ENM or ioinic metal all comparisons were made to the control sludge treatment throughout the study and not the soil control.
Table 2 The survival, percentage weight change, reproduction and total Zn and Ag concentrations in earthworms for the soil–sludge treatments and the sandy loam control soil (Woburn)a
Treatment |
% survival |
% weight change |
Reproduction (juveniles per worm per week) |
Total Zn concentration in earthworms (μg Zn g−1) |
Total Ag concentration in earthworms (μg Ag g−1) |
Each value represents mean ± one standard deviation; survival, weight change and reproduction had n = 4, Zn, Ag concentration: n = 12.
ENM = engineered nanomaterials.
c–f Means with the same superscript letters are not significantly different (p > 0.05). |
Control |
97.5 ± 5c |
27.9 ± 11.5c |
2.45 ± 0.318c |
116 ± 14.9c |
0.881 ± 0.129d |
High metal ionic metal |
100c |
58.1 ± 5.02c |
1.90 ± 0.617c |
86.9 ± 26.4d |
3.28 ± 1.86e |
High metal ENMb |
75 ± 2.65c |
41.1 ± 13.1c |
0.236 ± 0.277d |
113 ± 18.3c |
8.99 ± 2.75f |
Low metal ionic metal |
97.5 ± 5c |
48.1 ± 29.4c |
1.71 ± 0.367c |
122 ± 11.8c |
5.16 ± 0.925e,f |
Low metal ENM |
100c |
57.4 ± 18.1c |
1.688 ± 0.483c |
118 ± 17.5c |
4.62 ± 1.55d,e |
Soil control |
100c |
−4.46 ± 4.92d |
0.969 ± 0.267c |
90.3 ± 7.42d |
0.036 ± 0.011c |
For the as-synthesised Ag and Zn ENM and ionic metal exposures in the sandy loam soil, concentration–response relationships were obtained for all exposures. It was possible to calculate EC25, EC50 and EC90 values based on the total Ag or Zn concentration in the soil and the total Ag and Zn concentration in the earthworms in all cases (Table S1, ESI†).
Soil metal concentrations and earthworm responses
The metal concentrations in the ENM and ionic metal soil–sludge mixes are shown in Table 1. The Zn concentrations were close to the target value of 1400 mg kg−1, being on average 114% and 97% of the target in the high-metal ionic and ENM treatments respectively. Recovery of Ag was also close to the intended Ag concentrations, being 111% and 94% of the target in the ionic and ENM mixtures respectively (Table 1).
Earthworm survival and reproduction were clearly decreased more in the high-metal ENM soil–sludge treatment compared to all other treatments (Fig. 1, Table 2). Earthworm survival was reduced by 25% and reproduction was significantly reduced by 90% compared to the control soil–sludge treatment (ANOVA: F = 110.25, p < 0.001) (Table 2). In comparison the ionic metal soil–sludge treatments and the low-metal ENM treatment reduced reproduction, although not significantly, by 25–30% compared to the control soil–sludge (ANOVA: F = 2.55, p = 0.12) (Fig. 1) and there was 100% survival (Table 2). Earthworm weight change did not vary significantly across any of the soil–sludge treatments (ANOVA: F = 2.07, p = 0.135) (Table 2). The Zn concentrations in each of the soil–sludge treatments were above the EC25 and EC90 effect concentrations for the ionic metal and low metal ENM as-synthesised exposures, respectively (Fig. 1a, Table S1†). Only the EC90 value for the as-synthesised Zn ENM (1926 mg Zn kg−1) was above the Zn concentration in the high metal soil–sludge treatment (1690 mg Zn kg−1). In the case of Ag, all the soil–sludge treatments with the exception of the high metal ENM treatment had higher Ag soil concentrations than the EC25 or EC90 effect concentrations in the ionnic metal as-synthesised concentration–response curves (Fig. 1b). The Ag soil concentration high metal ENM treatment (94 mg Ag kg−1) was most similar to the ionic metal as-synthesised EC90 value (74 mg Ag kg−1) and indeed fell along as-synthesised ionic metal DRC.
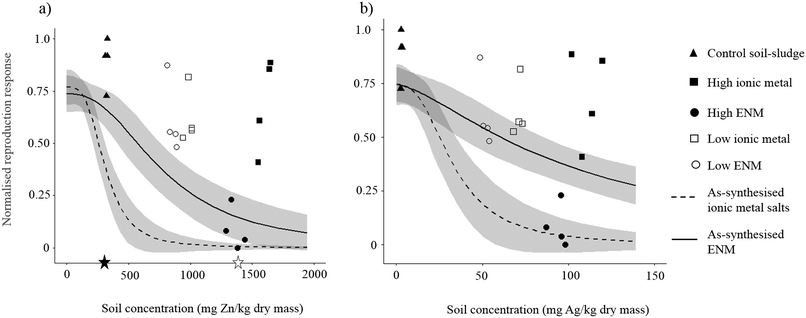 |
| Fig. 1 The normalised reproduction response (normalised to reproduction in the control soil–sludge) with increasing soil (a) Zn or (b) Ag concentrations. The data points are response data from the five soil–sludge treatments. Solid line = ENM concentration–response curve, dashed line = ionic metal concentration–response curve for Zn or Ag in sandy loam control soil. The grey shaded areas around the response curves represent the 95% confidence intervals around the curves. The black star represents the EU limit (86/278/EEC) (max. 300 mg kg−1) and the white star the US limit22 (1400 mg kg−1) for Zn in soil from sludge application to land. | |
Total metal concentrations in the earthworms
Earthworms exposed in the control soil–sludge had significantly higher total Ag concentrations (0.881 ± 0.129 μg Ag g−1), than those from the soil control (0.036 ± 0.011 μg Ag g−1), although both had significantly lower total Ag concentrations than in all other treatments (Fig. 2b, Table 2). Total Ag concentrations in earthworms from the ENM and ionic metal soil–sludge treatments were only compared to those from the control soil–sludge. The total Zn concentrations in the earthworms across all the soil–sludge treatments ranged from 86.9 ± 26.4 μg Zn g−1 to 122 ± 11.8 μg Zn g−1 (Fig. 2a, Table 2). Exposure of earthworms to the ionic metal and ENM soil–sludge treatments did not result in significantly higher total Zn concentrations in earthworms compared to the control soil–sludge (Fig. 2a). Total Zn concentrations in earthworms from the soil–sludge treatments were all below effect concentrations (EC25/EC90) shown in the concentration–response curves from the as-synthesised ionic metal and ENM Zn exposures (Fig. 2a). There was a poor correlation between total Zn concentrations in earthworms and the observed effect on reproduction across the soil–sludge treatments (r2 = 0.007). This suggests that Zn exposure in all the mixtures was within the physiological tolerance range of the earthworms for Zn (100–200 μg Zn g−1)31 although soil concentrations were above what would usually be tolerated in as-synthesised Zn exposures.
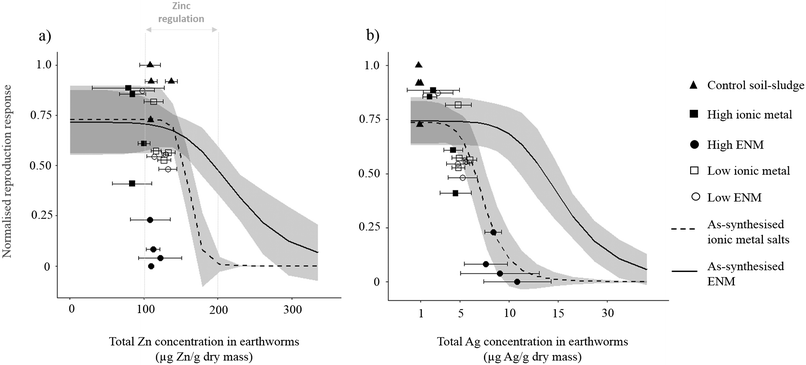 |
| Fig. 2 The normalised reproduction response (normalised to reproduction in the control soil–sludge) with increasing total (a) Zn or (b) Ag concentrations in earthworms. The data points are response data from the five soil–sludge mixtures. Error bars are the standard deviations of total metal concentrations in earthworms from three replicate earthworms in each treatment replicate. The model fits are from the as-synthesised ENM and bulk metal Zn and Ag exposure data. Solid line = ENM concentration–response curve, dashed line = ionic metal concentration–response curve for Zn or Ag sandy loam control soil. The grey shaded areas around the response curves represent the 95% confidence intervals around the curves. Vertical grey lines = limits for Zn regulation by earthworms.31 | |
Earthworms exposed in the high-metal ENM soil–sludge treatment had significantly higher total Ag concentrations than earthworms from all of the other treatments, with the exception of the low-metal ionic metal soil–sludge treatment (Fig. 2b, Table 2). There was a strong relationship between the total Ag concentrations in earthworms from the soil–sludge treatments and the observed effects on reproduction (r2 = 0.864). Earthworm from the soil–sludge treatments had total Ag concentrations that were also less than the effect concentrations (EC25/EC90) seen in the concentration–response curves from the as-synthesised ionic metal and ENM Ag exposures (Fig. 2b, Table S1†). However the earthworms from the high-metal ENM soil–sludge treatment accumulated significantly more Ag than those in other treatments (9 mg Ag kg−1) which was most similar to the EC90 for total Ag concentrations in earthworms (10.6 mg Ag kg−1) from the ionic metal as-synthesised exposure (Fig. 2b).
Porewater metal concentrations
Zn concentrations in the soil porewater were dependent on the total soil Zn concentrations; porewater in the high-metal treatments had greater Zn concentrations than in the low-metal treatments (Fig. 3a). The porewater Zn concentrations were significantly higher in the ionic metal soil–sludge treatments compared to the ENM soil–sludge treatments (ANOVA: F = 144.58, p < 0.01). Ultra-filtered porewater Zn concentrations did not differ significantly from the total porewater concentrations in any of the treatments (ANOVA: F = 0.22, p > 0.05) (Fig. 3a). Soil porewater Ag concentrations were significantly higher in the high-metal ENM and the two ionic metal soil–sludge treatments than in the control soil–sludge and low-metal ENM treatments (ANOVA: F = 17.09, p < 0.001) (Fig. 3b). Ultrafiltration significantly reduced the porewater Ag concentrations, for both ENM and ionic metal sludge treatments and Ag concentrations in the ultra-filtered porewaters did not differ across the soil–sludge treatments (F = 1.35; p > 0.05) (Fig. 3b).
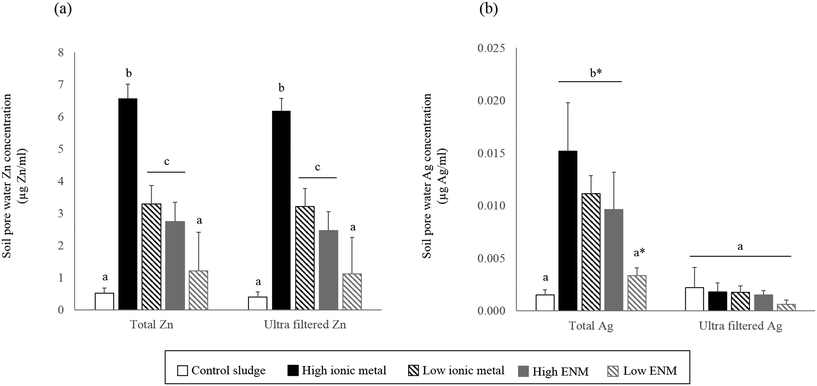 |
| Fig. 3 The total and ultra-filtered porewater concentrations of (a) Zn and (b) Ag in the soil–sludge mixtures extracted from the soils at the end of the toxicity exposure. Different letters denote significant differences between the total and ultra-filtered metal concentrations in the soil–sludge treatments. Asterisks next to the letters indicate where the total metal concentration in the porewater was significantly different from the ultra-filtered porewater concentration for the same soil–sludge treatment. Error bars are standard deviations. | |
Discussion
The application of sewage sludge to soils represents a realistic pathway for nanomaterials, or their transformation products, to enter terrestrial ecosystems. In order to understand, and ultimately regulate, the use and input of nanomaterials into the environment it is necessary to assess the risks resulting from land application of sludge produced from WWTP receiving inputs of nanomaterials, in scenarios that are realistic and representative of the final exposure for soil organisms. Ag and Zn nanomaterials were transformed by the wastewater treatment process into forms that were more thermodynamically stable under WWTP conditions, becoming largely or almost completely sulphidised or phosphatised.1,2 Crucially, ionic forms of Zn and Ag were also transformed, to a similar extent, producing essentially identical solid-phase speciation (coordination environment and oxidation state) in both the ENM- and ionic metal-treated sludges.1,16 There is evidence that Ag nanomaterial sulphidation reduces toxicity in controlled laboratory studies32,33 with similar passivation of Zn toxicity expected.34 Durenkamp et al. 2016 found that very little metal was leached during the six month aging process (in total over six month – 5 μg Zn g−1 and 2 μg Ag g−1) and that there was no difference in speciation between the ENM and ionic metal forms of Ag and Zn.1,23 However they did find that the inorganic N form did change; at the beginning of the aging process (i.e. fresh sludge) the majority was present in the form of NH4+ (a toxic form for earthworms) whereas NO3 dominated (up to 90%) at the end, but was the same in the ionic metal and ENM treatments.23 Earthworms in all soil–sludge treatments (control, ionic metal and ENM) gained similar weight over the duration of the exposure. However in this study, clear and significant differences were observed between the effects on earthworm reproduction when exposed in soils mixed with sludges derived from WWTP lines treated with either ENM or ionic metal forms. Although all earthworms gained weight in the three sludge treatments (control, ionic metal and ENM) over the duration of the exposure the ENM treated sludge depressed earthworm reproduction four times more than the same sludge treated with ionic metals. These results suggest the hypothesis that sludges showing similar solid-phase speciation of Zn and Ag should result in similar toxicity, regardless of the form of the spike, is incorrect. A similar conclusion was reached by Judy et al. 2015 for effects on the legume Medicago truncatula.16 Judy et al. 2015 used the same aged soil–sludge mixture as in this study and showed that the solid-phase speciation did not differ between ENM and ionic/bulk metal treatments. The solid-phase speciation was determined using X-ray absorption spectroscopy (XAS), which measures the oxidation state and local coordination environment of metals. It is possible that although the metals had similar coordination environments, the mineral particles may have had different sizes, morphologies, crystal structure or other nano-scale attributes that differed between treatments that are not measured by XAS which is an angstrom-scale characterisation.16 However given the greater toxicity of the ENM treatment, the U.S. regulations for ionic metals in sludge may not protect soil biota in the case of sludge derived from WWTP primarily receiving inputs of Zn and/or Ag in the form of ENMs.
The sludges contained Zn, Ag and Ti added to the WWTP inflow in either the ENM or ionic metal form and previous work had shown that similar solid-phase speciation of Zn and Ag was found in the ENM and the ionic metal sludges.1,16 Consequently the ionic metal effect data (EC50) for Zn and Ag were used to model the responses in both the ionic metal and ENM soil–sludge treatments. Thus, the toxic effects observed in the ENM soil–sludge treatments were compared to predicted effects (EC25 or EC90) calculated from both the ionic metal and the ENM as-synthesised effect data. The Ti concentrations in the soil–sludge treatments were between 1180 and 2467 mg Ti kg−1 (ref. 16) about 10 times lower than exposure concentration (10
000 mg Ti kg−1) where only slight effects of TiO2 ENMs were found on reproduction reported in toxicity studies.11 Hence in this study we assume that effects due to Ti were negligible and so were not included in the assessment of toxicity. The total Zn and Ag metal concentrations in the ENM and ionic metal sludge treatments were effectively the same. The total Zn and Ag soil concentrations were above the observed effect concentrations (EC25 or EC90) in the as-synthesised ionic metals soil exposures, particularly for Zn, but the predicted toxicity was not realised in the low-metal ENM or the ionic metal soil–sludge treatments. This could be expected when the exposure medium is considered; the sludge treatments had much higher organic matter content than the sandy loamy soil alone in which the as-synthesised metal exposures were conducted. It is widely established that ionic metals can show lower toxicity in soil with high organic matter.35,36 Another consideration is the aging of metals in soil which is known to greatly influence their toxicity to organisms in soils. In the case of ionic metals the aging in soils has been well described typically showing metals to become less toxic to organisms as they become more associated and bound to the solid phase in soils.37–39 Hence, a leaching-aging factor of 3 has been recommended to be applied to laboratory data in order to account for aging in the field and leaching of salts both of which will lower Zn toxicity.37 In this study the Zn concentration in the ENM soil–sludge treatment which caused a 90% effect (1690 mg Zn kg−1) was about three times greater than the ionic metal Zn EC90 (605 mg Zn kg−1). This means the safety factor applied to ionic metal response data may not be fully protective for Zn and certainly not Ag in cases where the metal is in the form of an aged or transformed ENM. Indeed Diez et al. 2015 showed that Ag ENM toxicity to earthworms increased over a one year time period (EC50 reduced from 1420 to 34 mg Ag kg−1) compared to a decrease in Ag ionic metal toxicity (EC50 increased from 49 to 104 mg Ag kg−1)8 which emphasizes the limitations of short-term exposures to as-synthesised ENMs in predicting ultimate toxicity. Overall in this study the ENM as-synthesised exposures showed low ENM toxicity compared to ionic metals and did not predict the level of effect observed in the high-metal ENM treatment better than ionic metal exposures.
Porewater measurements of metal in toxicity exposures may be used to explain variability in the solubility and thus the chemical reactivity of the metals across treatments. For ionic metals, increased solubility suggests greater bioavailability, though caution needs to be applied when considering porewater metal concentrations across soil types, due to the additional influence of variables such as the porewater pH and organic matter on metal availability.40,41 However, the low variability of pH and dissolved organic carbon across the different sludge treatments suggests that the variability in the porewater metal concentrations could be usefully used as a surrogate for metal reactivity and hence the bioavailability of ionic forms. Accordingly, if the observed uptake and toxicity were due to uptake of ionic Ag and/or Zn, it would be expected that the porewater concentrations of at least one metal would be higher in the ENM treatments compared to the ionic treatments. A small number of studies that have investigated the aging processes of ENMs in soils have shown the progression of metal toxicity to be different from that of ionic metals and that over time ENMs will undergo dissolution into the porewater which has been linked with greater toxicity.6,8,42 However, porewater concentrations of both Zn and Ag were consistently higher in the ionic treatments. Therefore, conventional patterns of ionic metal bioavailability cannot explain the observed effects and accumulation.
Organism body concentrations, in principle, provide the closest direct link to exposure since they integrate bioavailability and effects. Ag concentrations in the earthworm tissues varied significantly across the treatments; earthworms exposed to the ENM sludge accumulated more Ag than the ioinic metal treatments. In the high-metal ENM treatment there was also significantly greater accumulation of Zn in the ENM treatment than the ionic treatment. A similar pattern was observed by Judy et al. 2015, in M. truncatula where shoot concentrations of all three metals were higher in the ENM treatment than ionic/bulk, although only statistically significant for Zn.16 Total Zn concentrations in the earthworms were within the physiological limits for earthworm Zn regulation,31 and showed no relationship to total soil concentrations or to effects. However, the possibility of effects due to Zn cannot be precluded, as the earthworms may become stressed as a result of the energy requirements to maintain a physiologically stable body concentrations in the face of a Zn stress.43 Total Ag concentrations in earthworms did show a strong relationship with effect, across both the ENM and ionic metal treatments. The effects of the soil–sludge treatments were more similar to the ionic metal as-synthesised response curve compared to the ENM as-synthesised response. However as the effects in the high metal ENM treatment were observed at slightly lower total Ag concentrations in earthworms than those expected from the as-synthesised ionic metal or ENM exposures it would suggest that either both Ag and Zn contribute to the effects or that the transformations of the metals in the WWTP system increase their toxic potency relative to the as-synthesised forms. For example, it is possible Ag2S particles are being taken up and entering different locations in cells and then undergoing dissolution locally. There is evidence for the apparent changing toxicity of Ag ENMs in soils to earthworms; Diez et al. 2015 found that the EC50 (as total Ag concentration in the earthworms) for Ag initially spiked into a soil in the ENM form decreased from 64 μg Ag g−1 total Ag concentration in earthworms on initial toxicity testing to 7 μg Ag g−1 after incubation of Ag in the soil for a year.8 This trend was interpreted as being due to differential uptake of Ag ENMs and ionic Ag, coupled with gradual dissolution of Ag ENMs to ionic Ag over the incubation period. Thus, it is not possible to draw definite conclusions regarding the relative role of Zn and Ag in exerting toxic effects in the sludge treatments, since their toxic potencies may be dependent upon their chemical speciation. Furthermore, the differences in toxic effect observed across the ionic and ENM treatments suggest that the toxic potencies of the forms in the final sludges have been influenced by the nature of the starting metal form (i.e. ionic metal or ENM), despite the observation that the bulk phase speciation was similar in both sludge treatments. Indeed given that toxicity was unexpectedly highest in the high-metal ENM treatments, more research is required into the physicochemical form and distribution of the metals in the sludges to draw more definitive links with the observed toxicity.
This study was designed to represent the worst case scenario for ENM contamination associated with sludge application to soils. At present the maximum allowable concentrations are only set for Zn and these are to provide protection against the toxicity of metal salt forms. Although the metal salt exposures over-predicted toxicity for most of the sludge treatments it more closely predicted the ENM toxicity following transformation and aging. This study clearly shows as-synthesised ENM exposure studies do not accurately predict the toxicity of ENMs in environmentally realistic scenarios (aged and transformed after WWTP). Studies which show ENMs to be more toxic than the ionic metal are rare but there is a growing body of evidence that aging ENMs8 and/or exposure in more environmentally realistic forms such as sludge treated with ENMs16–18 can result in greater toxicity than when treated with the ionic/bulk metal forms. Although previous studies have demonstrated that sulifdation and phosphatation of Ag and ZnO nanomaterials greatly reduces their toxicity,14,34,44 ENMs can be more toxic than ionic metals after undergoing similar transformations. When both materials were aged the ENM metal forms were more toxic than the metal salt form suggesting that current Zn limits may not protect soil biota if the majority of metals enter the WWTPs from which these sludges are produced in the ENM form.
Acknowledgements
The authors wish to thank S. Marinakos at Duke University, Department of Civil and Environmental Engineering for her work in preparing particles. We also wish to acknowledge A. Romero and A. A. Horton for their assistance in the laboratory toxicity test work and A. Robinson for his assistance in creating the figures for the text. This work was funded by the Natural Environment Research Council Transatlantic Initiative for Nanotechnology and the Environment (TINE) grant NE/H013679/1, and NanoFASE EU Horizon 2020 research and innovation programme under grant agreement no.646002. M. D. was also supported by the UK Biotechnology and Biological Sciences Research Council (BBS/E/C/00005094). M. M. was supported by the Marie-Curie FP7-PEOPLE-2011-IEF, Micronanotox (PIEF-GA-2011-303140).
References
- R. Ma, C. Levard, J. D. Judy, J. M. Unrine, M. Durenkamp, B. Martin, B. Jefferson and G. V. Lowry, Fate of zinc oxide and silver nanoparticles in a pilot wastewater treatment plant and in processed biosolids, Environ. Sci. Technol., 2014, 48(1), 104–112 CrossRef CAS PubMed.
- B. Kim, C.-S. Park, M. Murayama and M. F. Hochella, Discovery and characterization of silver sulfide nanoparticles in final sewage sludge products, Environ. Sci. Technol., 2010, 44(19), 7509–7514 CrossRef CAS PubMed.
- R. Kaegi, A. Voegelin, B. Sinnet, S. Zuleeg, H. Hagendorfer, M. Burkhardt and H. Siegrist, Behavior of metallic silver nanoparticles in a pilot wastewater treatment plant, Environ. Sci. Technol., 2011, 45(9), 3902–3908 CrossRef CAS PubMed.
- A. Kelessidis and A. S. Stasinakis, Comparative study of the methods used for treatment and final disposal of sewage sludge in European countries, Waste Manage., 2012, 32(6), 1186–1195 CrossRef CAS PubMed.
- P. S. Tourinho, C. A. M. van Gestel, S. Lofts, C. Svendsen, A. M. V. M. Soares and S. Loureiro, Metal-based nanoparticles in soil: Fate, behavior, and effects on soil invertebrates, Environ. Toxicol. Chem., 2012, 31(8), 1679–1692 CrossRef CAS PubMed.
- P. L. Kool, M. D. Ortiz and C. A. M. van Gestel, Chronic toxicity of ZnO nanoparticles, non-nano ZnO and ZnCl2 to Folsomia candida (Collembola) in relation to bioavailability in soil, Environ. Pollut., 2011, 159(10), 2713–2719 CrossRef CAS PubMed.
- L. R. Heggelund, M. Diez-Ortiz, S. Lofts, E. Lahive, K. Jurkschat, J. Wojnarowicz, N. Cedergreen, D. Spurgeon and C. Svendsen, Soil pH effects on the comparative toxicity of dissolved zinc, non-nano and nano ZnO to the earthworm Eisenia fetida, Nanotoxicology, 2013, 8(5), 559–572 CrossRef PubMed.
- M. Diez-Ortiz, E. Lahive, S. George, A. Ter Schure, C. A. M. Van Gestel, K. Jurkschat, C. Svendsen and D. J. Spurgeon, Short-term soil bioassays may not reveal the full toxicity potential for nanomaterials; bioavailability and toxicity of silver ions (AgNO3) and silver nanoparticles to earthworm Eisenia fetida in long-term aged soils, Environ. Pollut., 2015, 203, 191–198 CrossRef CAS PubMed.
- S. Manzo, A. Rocco, R. Carotenuto, F. De Luca Picione, M. Miglietta, G. Rametta and G. Di Francia, Investigation of ZnO nanoparticles' ecotoxicological effects towards different soil organisms, Environ. Sci. Pollut. Res., 2011, 18(5), 756–763 CrossRef CAS PubMed.
- H. Wang, R. L. Wick and B. Xing, Toxicity of nanoparticulate and bulk ZnO, Al2O3 and TiO2 to the nematode Caenorhabditis elegans, Environ. Pollut., 2009, 157(4), 1171–1177 CrossRef CAS PubMed.
- H. McShane, M. Sarrazin, J. K. Whalen, W. H. Hendershot and G. I. Sunahara, Reproductive and behavioral responses of earthworms exposed to nano-sized titanium dioxide in soil, Environ. Toxicol. Chem., 2012, 31(1), 184–193 CrossRef CAS PubMed.
- M. Simonin, J. P. Guyonnet, J. M. F. Martins, M. Ginot and A. Richaume, Influence of soil properties on the toxicity of TiO2 nanoparticles on carbon mineralization and bacterial abundance, J. Hazard. Mater., 2015, 283, 529–535 CrossRef CAS PubMed.
- E. Lombi, E. Donner, S. Taheri, E. Tavakkoli, Å. K. Jämting, S. McClure, R. Naidu, B. W. Miller, K. G. Scheckel and K. Vasilev, Transformation of four silver/silver chloride nanoparticles during anaerobic treatment of wastewater and post-processing of sewage sludge, Environ. Pollut., 2013, 176, 193–197 CrossRef CAS PubMed.
- C. Levard, E. M. Hotze, G. V. Lowry and G. E. Brown, Environmental transformations of silver nanoparticles: Impact on stability and toxicity, Environ. Sci. Technol., 2012, 46(13), 6900–6914 CrossRef CAS PubMed.
- A. E. Pradas del Real, H. Castillo-Michel, R. Kaegi, B. Sinnet, V. Magnin, N. Findling, J. Villanova, M. Carrière, C. Santaella, A. Fernández-Martínez, C. Levard and G. Sarret, Fate of Ag-NPs in sewage sludge after application on agricultural soils, Environ. Sci. Technol., 2016, 50(4), 1759–1768 CrossRef CAS PubMed.
- J. D. Judy, D. H. McNear, C. Chen, R. W. Lewis, O. V. Tsyusko, P. M. Bertsch, W. Rao, J. Stegemeier, G. V. Lowry, S. P. McGrath, M. Durenkamp and J. M. Unrine, Nanomaterials in biosolids inhibit nodulation, shift microbial community composition, and result in increased metal uptake relative to bulk/dissolved metals, Environ. Sci. Technol., 2015, 49(14), 8751–8758 CrossRef CAS PubMed.
- C. Chen, J. M. Unrine, J. D. Judy, R. W. Lewis, J. Guo, D. H. McNear and O. V. Tsyusko, Toxicogenomic responses of the model legume Medicago truncatula to aged biosolids containing a mixture of nanomaterials (TiO2, Ag, and ZnO) from a pilot wastewater treatment plant, Environ. Sci. Technol., 2015, 49(14), 8759–8768 CrossRef CAS PubMed.
- B. P. Colman, C. L. Arnaout, S. Anciaux, C. K. Gunsch, M. F. Hochella, B. Kim, G. V. Lowry, B. M. McGill, B. C. Reinsch, C. J. Richardson, J. M. Unrine, J. P. Wright, L. Yin and E. S. Bernhardt, Low concentrations of silver nanoparticles in biosolids cause adverse ecosystem responses under realistic field scenario, PLoS One, 2013, 8(2), e57189 CAS.
- M. D. Fernández, M. N. Alonso-Blázquez, C. García-Gómez and M. Babin, Evaluation of zinc oxide nanoparticle toxicity in sludge products applied to agricultural soil using multispecies soil systems, Sci. Total Environ., 2014, 497–498, 688–696 CrossRef PubMed.
- C. García-Gómez, M. Fernández and M. Babin, Ecotoxicological evaluation of sewage sludge contaminated with zinc oxide nanoparticles, Arch. Environ. Contam. Toxicol., 2014, 67(4), 494–506 CrossRef PubMed.
- Y. Cheng, L. Yin, S. Lin, M. Wiesner, E. Bernhardt and J. Liu, Toxicity reduction of polymer-stabilized silver nanoparticles by sunlight, J. Phys. Chem. C, 2011, 115(11), 4425–4432 CAS.
-
EPA, A Guide to the Biosolids Risk Assessments for the EPA Part 503 Rule, In Agency, E. P., Ed., Washington DC, 1995 Search PubMed.
- M. Durenkamp, M. Pawlett, K. Ritz, J. A. Harris, A. L. Neal and S. P. McGrath, Nanoparticles within WWTP sludges have minimal impact on leachate quality and soil microbial community structure and function, Environ. Pollut., 2016, 211, 399–405 CrossRef CAS PubMed.
-
OECD, Test No. 222: Earthworm Reproduction Test (Eisenia fetida/Eisenia andrei), OECD Publishing, 2004 Search PubMed.
- R. K. Sinha, S. Herat, G. Bharambe and A. Brahambhatt, Vermistabilization of sewage sludge (biosolids) by earthworms: converting a potential biohazard destined for landfill disposal into a pathogen-free, nutritive and safe biofertilizer for farms, Waste Manage. Res., 2010, 28(10), 872–881 CrossRef CAS PubMed.
- M. G. Vijver, J. P. M. Vink, C. J. H. Miermans and C. A. M. van Gestel, Oral sealing using glue: a new method to distinguish between intestinal and dermal uptake of metals in earthworms, Soil Biol. Biochem., 2003, 35(1), 125–132 CrossRef CAS.
- S. Sauvé, W. Hendershot and H. E. Allen, Solid-solution partitioning of metals in contaminated soils: Dependence on pH, total metal burden, and organic matter, Environ. Sci. Technol., 2000, 34(7), 1125–1131 CrossRef.
- S. Thakali, H. E. Allen, D. M. Di Toro, A. A. Ponizovsky, C. P. Rooney, F.-J. Zhao, S. P. McGrath, P. Criel, H. Van Eeckhout, C. R. Janssen, K. Oorts and E. Smolders, Terrestrial biotic ligand model. 2. Application to Ni and Cu toxicities to plants, invertebrates, and microbes in soil, Environ. Sci. Technol., 2006, 40(22), 7094–7100 CrossRef CAS PubMed.
- A. R. Whitley, C. Levard, E. Oostveen, P. M. Bertsch, C. J. Matocha, F. V. D. Kammer and J. M. Unrine, Behavior of Ag nanoparticles in soil: Effects of particle surface coating, aging and sewage sludge amendment, Environ. Pollut., 2013, 182, 141–149 CrossRef CAS PubMed.
- G. Cornelis, J. K. Kirby, D. Beak, D. Chittleborough and M. J. McLaughlin, A method for determination of retention of silver and cerium oxide manufactured nanoparticles in soils, Environ. Chem., 2010, 7(3), 298–308 CrossRef CAS.
- K. Lock and C. R. Janssen, Zinc and cadmium body burdens in terrestrial oligochaetes: Use and significance in environmental risk assessment, Environ. Toxicol. Chem., 2001, 20(9), 2067–2072 CrossRef CAS PubMed.
- B. C. Reinsch, C. Levard, Z. Li, R. Ma, A. Wise, K. B. Gregory, G. E. Brown and G. V. Lowry, Sulfidation of silver nanoparticles decreases Escherichia coli growth inhibition, Environ. Sci. Technol., 2012, 46(13), 6992–7000 CrossRef CAS PubMed.
- C. L. Doolette, M. J. McLaughlin, J. K. Kirby and D. A. Navarro, Bioavailability of silver and silver sulfide nanoparticles to lettuce (Lactuca sativa): Effect of agricultural amendments on plant uptake, J. Hazard. Mater., 2015, 300, 788–795 CrossRef CAS PubMed.
-
S. Rathnayake, Transformations, bioavailability and toxicity of manufactured ZnO nanomaterials in wastewater, University of Kentucky, 2013 Search PubMed.
- D. J. Spurgeon and S. P. Hopkin, Effects of variations of the organic matter content and pH of soils on the availability and toxicity of zinc to the earthworm Eisenia fetida, Pedobiologia, 1996, 40, 80–96 CAS.
- T. Crommentuijn, A. Doornekamp and C. A. M. Van Gestel, Bioavailability and ecological effects of cadmium on Folsomia candida (Willem) in an artificial soil substrate as influenced by pH and organic matter, Appl. Soil Ecol., 1997, 5(3), 261–271 CrossRef.
- E. Smolders, K. Oorts, P. Van Sprang, I. Schoeters, C. R. Janssen, S. P. McGrath and M. J. McLaughlin, Toxicity of trace metals in soil as affected by soil type and aging after contamination: Using calibrated bioavailability models to set ecological soil standards, Environ. Toxicol. Chem., 2009, 28(8), 1633–1642 CrossRef CAS PubMed.
- C. E. Smit and C. A. M. Van Gestel, Effects of soil type, prepercolation, and ageing on bioaccumulation and toxicity of zinc for the springtail Folsomia candida, Environ. Toxicol. Chem., 1998, 17(6), 1132–1141 CrossRef CAS.
- K. Lock and C. R. Janssen, Influence of ageing on zinc bioavailability in soils, Environ. Pollut., 2003, 126(3), 371–374 CrossRef CAS PubMed.
- S. Lofts, D. J. Spurgeon, C. Svendsen and E. Tipping, Deriving soil critical limits for Cu, Zn, Cd, and Pb: A method based on free ion concentrations, Environ. Sci. Technol., 2004, 38(13), 3623–3631 CrossRef CAS PubMed.
- D. J. Spurgeon, S. Lofts, P. K. Hankard, M. Toal, D. McLellan, S. Fishwick and C. Svendsen, Effect of pH on metal speciation and resulting metal uptake and toxicity for earthworms, Environ. Toxicol. Chem., 2006, 25(3), 788–796 CrossRef CAS PubMed.
- C. Coutris, E. J. Joner and D. H. Oughton, Aging and soil organic matter content affect the fate of silver nanoparticles in soil, Sci. Total Environ., 2012, 420, 327–333 CrossRef CAS PubMed.
- C. A. M. van Gestel, E. M. Dirven-van Breemen and R. Baerselman, Proceedings of the 2nd European Conference on Ecotoxicology Accumulation and elimination of cadmium, chromium and zinc and effects on growth and reproduction in Eisenia andrei (Oligochaeta, Annelida), Sci. Total Environ., 1993, 134, 585–597 CrossRef.
- D. L. Starnes, J. M. Unrine, C. P. Starnes, B. E. Collin, E. K. Oostveen, R. Ma, G. V. Lowry, P. M. Bertsch and O. V. Tsyusko, Impact of sulfidation on the bioavailability and toxicity of silver nanoparticles to Caenorhabditis elegans, Environ. Pollut., 2015, 196, 239–246 CrossRef CAS PubMed.
Footnote |
† Electronic supplementary information (ESI) available. See DOI: 10.1039/c6en00280c |
|
This journal is © The Royal Society of Chemistry 2017 |