DOI:
10.1039/C6CS00517A
(Review Article)
Chem. Soc. Rev., 2017,
46, 158-196
Carbon nanotubes: a novel material for multifaceted applications in human healthcare†
Received
9th July 2016
First published on 14th November 2016
Abstract
Remarkable advances have been achieved in modern material technology, especially in device fabrication, and these have facilitated the use of diverse materials in various applications. Carbon nanotubes (CNTs) are being successfully implemented in drug delivery, sensing, water purification, composite materials, and bone scaffolds. Thus, CNTs must meet a wide range of criteria such as surface modification, high aspect ratio, desired conductivity, high porosity and loading, non-toxicity, specificity, and selectivity, and compatibility for device fabrication. The main focus of this review is to explore the maximum applications of CNTs for human health, and we particularly focus on nanocarrier and biomedical applications. The scope of this review initially covers the basic aspects of CNTs and is also extended further to describe their synthesis strategies as well as various challenges encountered in their functionalization, dispersion, and toxicity. Our discussion also emphasizes future directions for these emerging fields of research.
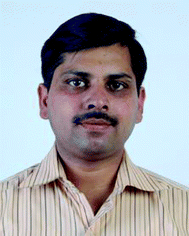
Sandeep Kumar
| Dr Sandeep Kumar is working as Assistant Professor at the Department of Bio and Nano Technology, Guru Jambheshwar University of Science and Technology, Hisar, Haryana, India. Dr Kumar did his PhD at Panjab University, Chandigarh. His research interests include synthesis of nanomaterials, nanocarriers for healthcare applications, nanomaterial based sensors, biomaterials, and nanotoxicology. He has one patent and published more than 50 research papers in many reputed international journals. Dr Kumar runs both international and national sponsored research projects from different funding agencies. Dr Kumar has visited Hanyang University, Seoul, South Korea, as a visiting Professor and also Australia, UK, Scotland, and Bangkok under different schemes of Govt of India. |
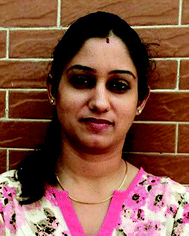
Ruma Rani
| Ruma Rani is a Doctoral Research Fellow at the Department of Bio & Nano Technology, Guru Jambheshwar University of Science and Technology, Hisar, Haryana, India. She did her bachelor's in Pharmacy and master's in Medical Biotechnology with first rank in the University (Gold Medalist-Master Degree). She has been awarded the prestigious fellowship ‘INSPIRE’ by the Department of Science and Technology, Ministry of Science and Technology, Govt of India, for pursuing a full-time PhD study. Her research interests include synthesis and evaluation of nanoformulations of herbal bioactive compounds, drug delivery, and nanotoxicology. |
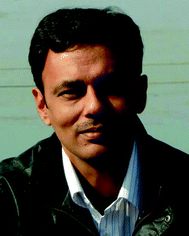
Neeraj Dilbaghi
| Professor Neeraj Dilbaghi completed his doctorate degree in Microbiology from CCSHAU, Hisar, India, and is presently working at the Department of Bio and Nano Technology, Guru Jambheshwar University of Science and Technology, Hisar, Haryana, India. Dr Dilbaghi holds the position of Director, Internal Quality Assurance Cell (IQAC) and Incharge Radioecology Centre of GJUS&T, Hisar. His current research focuses on biosensors, diagnostics, drug delivery, and toxicological evaluation of nanomaterials. He has published more than 100 research papers in many reputed international journals. Dr Dilbaghi has received several grants from national and international funding agencies like DST, UGC, BARC, etc. to manage his research activities. |
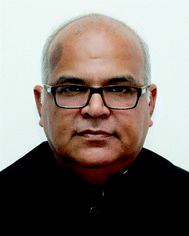
Tankeshwar Kumar
| Professor Tankeshwar Kumar is presently Vice-Chancellor of Guru Jambheshwar University of Science and Technology, Hisar. He completed post-doctorate work at Abdus Salam ICTP, Italy. Lastly, he worked in the capacity of UGC Professor at Panjab University, Chandigarh. The research outcomes achieved by Prof. Tankeshwar Kumar over the last two decades made distinct contributions to our understanding of atomic motions in liquids, micro-fluidics, and nano-fluidics. Relevance of the work to the study of flow of fluids like blood in arteries has been found. Dr Tankeshwar has received numerous research grants in his career. |
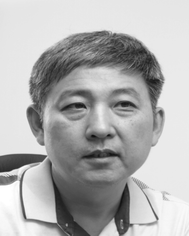
Ki Hyun Kim
| Prof. Ki-Hyun Kim was at Florida State University for an MS (1984–1986) and at the University of South Florida for a PhD (1988–1992). He was a Research Associate at ORNL, USA (1992–1994). Then, he moved to Korea and joined Sangji (1995–1998) and Sejong University (1999–2013). In 2014, he moved to Hanyang University. His research focuses on environmental analysis, air quality management, and materials engineering. He was awarded a National Star Faculty in 2006. He has published more than 410 articles in SCI journals and is serving as an editorial member of journals (e.g., Air Pollution Research, Sensors, and Scientific World). |
1. Introduction
Nanotechnology is a multi-disciplinary field that deals with a variety of materials produced at the nanometer scale through different physical, chemical, and biological routes. Nanomaterials possess novel properties that are typically not observed in their bulk counterparts. Hence, they may overcome the many limitations of existing products with respect to cost, functionality, fabrication strategies, and overall performance. This emerging technology has indeed opened up new opportunities for applications in the fields of biotechnology, molecular biology, and nearly all disciplines of veterinary and animal sciences.
Nanomaterials have been investigated intensively as carriers in modern healthcare applications due to their tunable surface properties. For example, a variety of materials including polymer-based carriers, emulsions, solid lipid nanoparticles, hydrogels, liposomes, niosomes, and carbon-based nanomaterials have been employed in drug delivery systems to ensure that a sufficient concentration of the drug reaches its destination after passing any physiological barriers. Spherical nanoparticles are currently the predominant form of nanomaterials used, as the spherical forms are easier to manufacture than non-spherical nanoparticles such as nanowires, microtubes, and nanotubes. The structures of micro- and nanotubes resemble those of tiny straws, affording them many advantages over other nanoparticles. In particular, carbon nanotubes (CNTs) in particular, have several interesting properties related to their structure, morphology, functionality, stability, ease of modification, and suitability in hybrid materials. As such, CNTs are often a material of choice for the fabrication of devices with unprecedented features.
As one of the most commonly used nanomaterials, CNTs have attracted numerous researchers as a material platform in the field of healthcare as well. CNTs are constructed as hollow cylindrical tubes consisting of carbon (graphite) with a high aspect ratio (∼1000) and sp2 hybridization. Depending on the number of graphite layers, CNTs can be classified as single-walled nanotubes (SWNTs), double-walled nanotubes (DWNTs), and multi-walled nanotubes (MWNTs).1–3 The nature of the carbon atoms in CNTs produces amazing properties that are suitable for a variety of applications in the electronics, photonics, renewable energy, drug delivery and the biomedical sector.4–10 Therefore, the production of CNTs has been increasing drastically over the last few years. The global market for CNT primary grades was $158.6 million in 2014. This market was projected to reach $167.9 million in 2015 and $670.6 million in 2019, with a CAGR of 33.4% from 2014 to 2019.11 Technological development and innovative processing methods have helped improve the skills involved in the synthesis and functionalization of CNTs.
Fig. 1(a) presents a pie chart containing basic statistics based on the number of publications with topic titles that include “CNT” and “application” (N = 1004, source: PubMed data). In Fig. 1(b), the results are provided for only the publications cited in this review paper (N = 676).
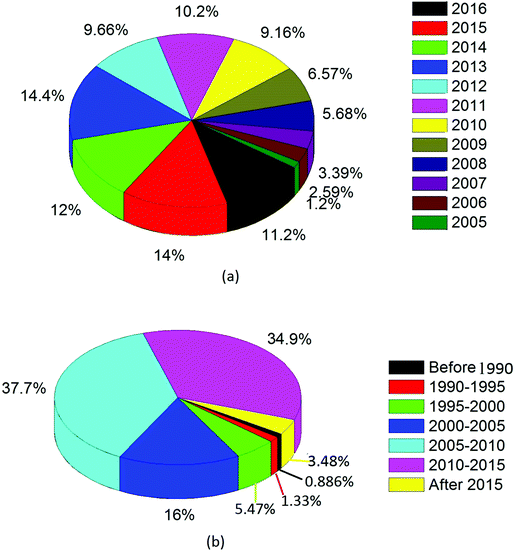 |
| Fig. 1 Pie charts drawn to describe the basic statistics of publications related to this research: (a) total number of publications (N = 1004) with the title topics including “CNT” and “application” based on PubMed data on September 6th, 2016 and (b) chart drawn using 676 publications cited in this review paper. | |
CNTs are used in a wide spectrum of applications due to their extraordinary physical and chemical properties, including an extremely large surface area to volume ratio, small diameter, hollow structure, exceptional optical and electrical properties, and exceptionally high tensile strength. In this article, we provide in-depth reviews on various application fields of CNTs with an emphasis on the healthcare sector. We specifically focus on drug delivery, nanoprobe sensing, antimicrobial treatment, cancer treatment, photothermal ablation, photoacoustic imaging, and bone scaffolds. Lastly, the issues associated with CNT toxicity and dispersion are also addressed as challenges to be resolved.
2. Synthesis strategies for CNTs and required characteristics of selected CNTs
2.1 Synthesis strategies for CNTs
Carbon nanotubes can be synthesized by various methods including arc discharge, laser ablation, and chemical vapor deposition (CVD). Arc discharge and laser ablation techniques developed earlier generally require high temperature (>1700 °C) during synthesis. However, these methods have now been replaced with CVD, which can be conducted at lower temperatures (<800 °C). Along with these established techniques, some non-standard methods like pyrolysis and hydrothermal treatment have also been employed.12 The major synthesis strategies for CNTs are briefly described below.
(i) Arc discharge technique.
This technique has been widely used for the fabrication of CNTs using direct current (DC) arc discharge. The CNTs are produced by arcing the graphite cathode and graphite anode electrode in a vacuum chamber in the presence of an inert gas (helium or argon); MWNTs are produced in this method.2,13 The electrical discharge on the anode results in high temperature (i.e., up to 6000 °C) that initiates sublimation of graphite (transformation of solid carbon directly to a gaseous state). These gaseous carbon atoms move toward the cathode and are deposited on its surface. Under certain circumstances, the anode is filled with metals (e.g., Fe, Co, and Ni) for the production of SWNTs.14,15 The production cost is higher in the case of SWNTs because the metal catalyst needs to be removed from the resulting product.16,17 There are a number of process parameters (e.g., temperature of the chamber, concentration of the carbon vapor in the inert gas, the addition of promoters, the exact composition and concentration of the catalyst, and the presence of hydrogen) that influence the size and purity of carbon nanotubes in the arc-discharge process.18 Recently, many researchers have employed stainless steel electrodes, silicon substrates, nickel mesh catalysts, and non-vacuum local arc discharge for the growth of CNTs using ethanol (as the carbon precursor); note that the use of stainless steel electrodes is helpful in preventing erosion of the electrodes, leading to the improvement in the quality of the CNTs.19,20 Other research groups have also used other chemicals (such as liquid N2, deionized water, and aqueous solutions of NiSO4, CoSO4, FeSO4, and NaCl) in the reaction environment for the synthesis of CNTs using arc discharge.21–38 Cai et al.39 accomplished the catalyst-free synthesis of vertically aligned CNTs using hydrogen for the generation of an arc discharge. Maria and Mieno40 carried out the synthesis of SWNTs using bipolar pulsed arc discharge, and they were able to obtain good quality SWNTs relative to the DC arc discharge method. Berkmans et al.41 reported the synthesis of single walled carbon structures of nanotube and nanohorn hybrids in a single step by arc discharge in open air, at relatively low current densities. Recently, Belgacem et al.42 reported the deposition of boron and nitrogen co-doped MWNTs through an arc-discharge method. Further, the synthesis of CNTs with a narrow diameter distribution (20–30 nm) and with a length up to 1 μm was achieved using this method.
(ii) Laser-ablation technique.
In this technique, an intense laser pulse is needed to ablate a graphite target at a very high temperature in the presence of an inert gas.43 The amount and quality of CNTs produced are strongly dependent on the combined effects of diverse variables such as (i) the wavelength and power of the laser, (ii) the structural and chemical composition of the target material, (iii) the chamber pressure and the chemical composition, (iv) flow and pressure of the buffer gas, (v) the substrate and ambient temperature, and (vi) the distance between the target and the substrates.12,44,45 Like the arc discharge technique, the laser ablation technique can yield both SWNTs and MWNTs. This method was reported for the first time by Guo et al.46 in 1995. They used a laser instead of arc discharge to generate the required heat energy. The laser ablation method can be used to produce purified forms of CNTs but at smaller quantities compared to the arc discharge method. However, the amount of synthesized CNTs can increase if one uses bimetallic graphite containing a Ni/Y catalyst (where the concentration of Ni is always higher than that of Y or with Ni/Co in the same ratio); as such, a high yield of SWCNT bundles can be achieved using the laser ablation method with these catalysts.47,48 It is noteworthy that the reaction temperature of the laser ablation method for the synthesis of CNTs is higher than 1200 °C, which it is still slightly lower than that required by the arc discharge method.49–51
Although arc discharge and laser ablation methods are useful for moderately high yield of good quality CNTs, they can still suffer from certain limitations listed below:52
• These methods require vacuum conditions and high-temperature reaction conditions, which makes them expensive to scale-up.
• CNTs produced by these methods also contain a mixture of unwanted materials in the form of impurities that need to be removed by adopting different procedures. The separation of unwanted materials from CNTs can cause problems and increase cost.
• CNT production using these methods cannot proceed on a continuous basis because the graphite electrodes and targets have to be replaced continuously during the synthesis process.
(iii) Chemical vapor deposition (CVD) technique.
The CVD technique was introduced in 1993 for large-scale production of CNTs.53 The CVD method involves the pyrolysis of hydrocarbons (e.g., acetylene, ethylene, propylene, methane, benzene, and toluene)54–61 or other carbon feedstock (e.g., polymers and carbon monoxide)62–65 that are carried in a stream of an inert gas into a chamber with metal catalysts (e.g., Ni, Fe, and Co).66,67 Various parameters (such as hydrocarbon precursor composition, catalyst, temperature, pressure, gas-flow rate, deposition time, and reactor geometry) have been identified that can be used to control the growth mechanism of CNTs.68,69 Yan et al.70 reviewed the scalable CVD-based processes for the synthesis of CNTs including: (1) “Carbon Multiwall Nanotubes” made by Hyperion Company,71 (2) the Endo process developed at Shinshu University,72 (3) the “CoMoCAT Process at SWeNT” developed at the University of Oklahoma,73,74 (4) the “HiPCo Process” of Rice University,75,76 (5) the “Nano Agglomerate Fluidized” process developed by Tsinghua University,77,78 (6) the “Baytube” process from the Bayer Company,79 (7) processes for the supergrowth of SWCNT arrays,80–82 and (8) super-aligned CNTs for yarn preparation,83 for the synthesis of CNTs.
At present, CVD is the most intensively investigated method for the mass production of different types of CNTs due to its enhanced yield rate and simple instrumentation relative to others like arc discharge and laser ablation. The temperature of the CVD-based synthesis of CNTs generally ranges from 500 to 1200 °C at atmospheric pressure. The basic structure of CNT (e.g., diameter, length, and alignment) can be controlled effectively by controlling the temperature. MWNTs with diameters of 40–60 nm were prepared by the catalytic decomposition of methane at 680 °C for 120 min, using nickel oxide–silica binary aerogels as the catalyst.84,85 The growth of CNTs in situ on the pretreated graphite electrode was also explored using Ni(NO3)2 as the catalyst.86 The prepared CNTs were 200 to 1000 nm long with outer and inner diameter of 80 and 20 nm, respectively.
The diameter of synthesized CNTs was found to be dependent on the temperature; as the temperature increased, the diameter also increased.86 The synthesis of SWNTs was generally optimized under high temperature conditions around 900 °C, while that of MWNTs was optimized under low temperature conditions at around 650 °C. The pressure of the gaseous carbon precursor can be directly controlled by adjusting the flow rate of gas.87 When using a solid hydrocarbon precursor, the vapor pressure was optimized by controlling the hydrocarbon precursor mass, vaporizing temperature, and the flow rate of carrier gas.88 Likewise, for a liquid hydrocarbon precursor, the vapor pressure was optimized by heating the precursor at a certain temperature before it was pumped into the reactor.89 As shown in Fig. 2, many CVD alternatives have been established for the synthesis of CNTs in a controlled way to facilitate mass production: (1) fixed bed reactors,90–94 (2) fluidized bed reactors,95–99 (3) laser assisted,100,101 (4) plasma-assisted (plasma sources including microwave discharge, hot filament, dc-glow discharges, radio frequency (RF) capacitive coupled plasmas and RF inductively coupled plasmas),102–109 (5) aerosol-assisted,110–112 and (6) floating catalysts.113–115
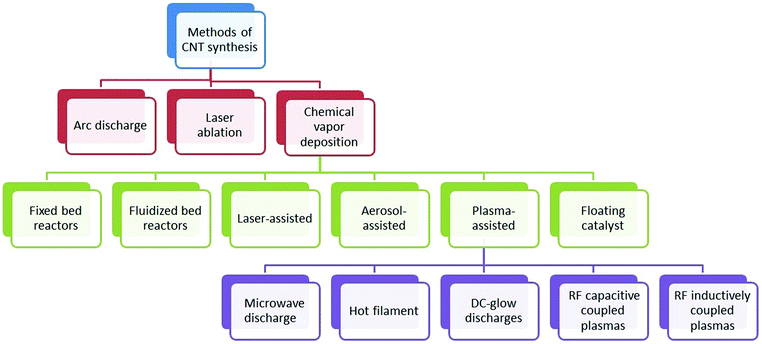 |
| Fig. 2 Classification of synthesis methods employed for carbon nanotubes. | |
Care should be taken for the removal of metal catalyst impurities for any method employed to synthesize CNTs because metals may affect the electro-catalytic properties of the CNTs. Although several methods have been adopted for purification, the amount of purified CNTs still varies from one technique to another. The large-scale production of purified CNTs requires cost effective techniques capable of isolating the purified product from metallic and amorphous impurities. Such approaches are expected to lower the market price of CNTs for utilization in diverse applications. Thus, it is a challenge for researchers to fabricate CNTs that are economically feasible for commercial and practical applications. The expected widespread use of CNTs in consumer products is likely to increase the chance of their exposure to manufacturing employees, consumers, and the environment.116–121 Because the release of CNTs may occur throughout the life of the product, it is important to identify the circumstances where humans and the environment might be affected by their exposure.122 It is thus desirable to accurately establish the true potential for their exposure through various routes as well as their long-term toxicity profile, especially for technical personnel involved in their production and transportation. The production of numerous CNT-based electronic devices (e.g., biomedical products) also raises concerns about their degradation in the form of e-waste. CNTs have been demonstrated to have cytotoxicity, which is closely associated with their surface coatings (functionalized groups) through in vivo pharmacokinetics and biodistribution of CNTs, as measured in biological samples.123 Therefore, a significant concern has been raised regarding their side effects on the environment and their effect on human health.124–127
2.2 Required characteristics of selected CNTs
CNTs have attracted the interest of many researchers in the field of nanomedicine and nanotechnology because of their distinctive photosensitive, mechanical, optical and electrical properties. The materials in this size range reveal novel, favorable properties when compared with the bulk. Indeed, CNT research has been one of the most productive subfields in nanobiotechnology. It has created a bridge between physical and biological sciences for the development of innovative tools with the potential to revolutionize the healthcare sector. The use of CNTs has favorably been expanded into various fields due to their many desirable properties listed below.
(i) The large surface area to volume ratio and hollow structural properties make CNTs suitable for drug loading and drug delivery.128–130
(ii) The hydrophobic nature of CNTs provides an attractive platform for the delivery of biomolecules like DNA,13–133 RNA,134,135 proteins,136–138 and immune modulators139,140 through the biological membrane without harming the adjacent cells.
(iii) The ease of functionalization allows CNTs to self-direct and target a site for the delivery of anticancer drugs.141–146
(iv) The optical properties of CNTs allow their use as a contrast agent or an optical tag for various biomedical applications such as photodynamic therapy, photothermal ablation, and photoacoustic imaging.147–151
(v) The excellent conductive profile of CNTs offers a unique platform for the development of biosensors including enzyme biosensors,152,153 gene biosensors,154,155 cancer biosensors,156,157 and sensors for air pollutants.158,159
(vi) The extraordinary mechanical strength of CNTs helps to provide a surface for cell adhesion, proliferation, and differentiation.160–162
(vii) The large surface area of CNTs make them in the category of excellent nanoadsorbents for the removal of metallic ions, organic compounds, and dyes from an aqueous solution163–166 or as a stationary phase for the separation of biomolecules including DNA and protein during electrophoresis.167,168
(viii) As CNTs exhibit good antimicrobial activity, they are useful as antimicrobial treatments in liquid media.169–171
3. Advancements in CNT technology for nanocarrier applications
3.1 Targeted and controlled release
Targeted drug delivery is a method for delivering an active therapeutic agent to a specific part of the body for a prolonged period. There are two basic mechanisms that are used for targeted nanoscale drug delivery: passive targeting and active targeting.172 Passive targeting is based on the size of the carrier and the growth behavior of tumors. In tumor cells, the endothelial cells may be spaced further from each other than normal cells. This spacing allows increased permeability of the drug carrier into the tumor cells. Moreover, the hydrophobic nature of the CNTs allows them to stay in the circulation for prolonged periods. This synergy leads to enhanced permeability and retention (EPR). The second mechanism involves using a CNT carrier to actively target and selectively deliver drug molecules to cancer cells or a diseased part of the body. For proper targeting, CNTs are functionalized with a tumor-targeting ligand so that they can easily distinguish tumor cells from surrounding healthy cells.173 Both mechanisms of drug targeting are shown in Fig. 3.
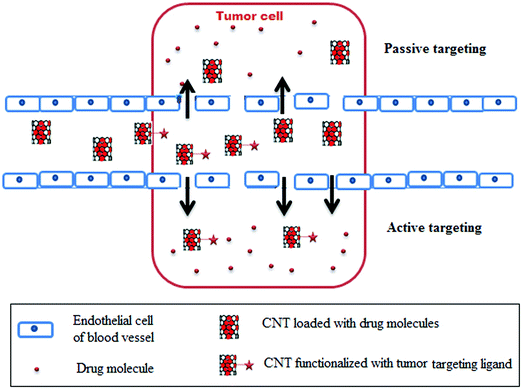 |
| Fig. 3 Targeted drug delivery by using CNTs. The tumor cell can be targeted by two mechanisms, passive and active targeting. The former mechanism of targeting proceeds via the EPR effect. The CNTs loaded with drug molecules crosses the endothelial cells of the blood vessels to target the tumor cell only because of disorganization of endothelial cells in the blood vessels of tumor cells. Therefore, it can lead to passive dilatation of vessels while increasing the extravasation of CNT loaded drug molecules in tumor tissue. The latter mechanism of targeting proceeds via functionalization of CNTs with a specific tumor-targeting ligand which is recognized by the receptors present on the tumor cells only. Thereafter, functionalized CNTs are internalized by the tumor cell to release the drug molecule. | |
The behavior of confined fluids on the nanoscale is quite different from the fluid confined at the micro or larger length scales. The dynamics of fluids confined in such geometries have been studied using experimental and theoretical techniques.174,175 Dynamics of fluids confined at the nanometer scale is attracting great attention both in fundamental and applied sciences due to the numerous applications which include biological and engineering devices. Strong interactions between fluid molecules and wall molecules influence the static and dynamic properties of confined fluids. The dynamical model for the study of the self-diffusion of a fluid confined in one direction176 and confined in two directions177 is documented. Devi et al.177 reported that diffusion parallel to the walls of the channel is different from the diffusion perpendicular to the walls. They also showed that denser fluids show more confinement effects than dilute fluids. A theoretical model based on microscopic considerations was developed, and it demonstrated that diffusion should fall significantly near the walls.178 CNT-based carriers have been designed or targeted in such a way so that they release the drug moiety after internalization into the cell. This mechanism is further discussed in the cancer diagnosis and treatment section.
For the delivery of drug molecules, controlled release occurs in response to external and/or internal stimuli or after a certain elapsed time. Changes in temperature, pH, enzyme activity, and redox potential are all internal stimuli. In contrast, changes in magnetic field, electric field, light irradiation, and temperature are classified as external stimuli.179–181 Controlled release of a drug in response to external or internal stimuli is illustrated in Fig. 4.
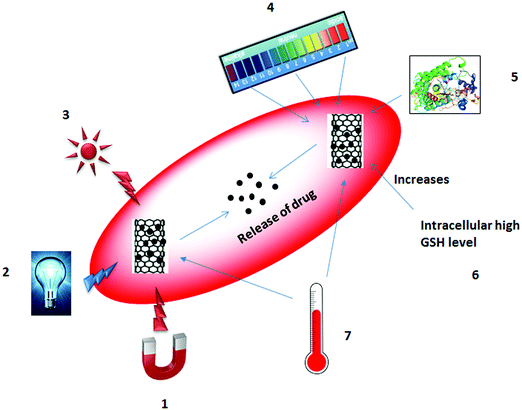 |
| Fig. 4 Controlled release of a drug via external and internal stimuli on CNTs: (1) magnetic stimuli, (2) electric stimuli, (3) light irradiation (NIR), (4) change in pH, (5) change in enzyme concentration, (6) redox potential (high GSH level), and (7) change in temperature. All of these variables are responsible for the release of drug molecules from CNTs. GSH stands for glutathione. | |
Changes in temperature can be considered as both an external and an internal stimulus. The effect of temperature has been investigated most intensively for the controlled release of drug based on the structure of the polymer (from a shrunken form to a swollen form or vice versa) or solubility.182–184 Magnetic fields are also useful in that they are harmless to the cell, unlike other external stimuli including electric field or light irradiation. In 1960, Freeman et al.185 proposed the controlled release of a drug using an applied magnetic field. An external magnetic field produces heat, which causes hyperthermia of the nanoparticles and results in the release of the drug molecules either by disruption of nanoparticles or by the pumping effect.186,187 Electric field influence the release of drugs either by ionization of the structure or through shrinking or swelling of the polymers. The good electrical properties of the CNTs can speed up the release of drugs through the heating effect. Im et al.188 invented a transdermal patch using a polymer–MWNT network for assessing electric properties. Light irradiation can trigger the release of drugs either by cleavage of a bond or by generation of reactive oxygen species (ROS) through the absorption of photon energy by the nanocarriers.189–191 It can also be used in photochemotherapy for the release of anticancer drugs and the destruction of tumor cells.192–194 Changes in pH are internal stimuli that can occur within the body. In tumor tissues, a reduced pH is observed compared to normal tissues; this difference can stimulate the release of the drug.195–197 Change in redox potential between the intracellular/extracellular compartments and/or between healthy cells/diseased cells can also provide the stimulus for drug release.198–200 Enzyme-sensitive nanocarriers have the potential for diagnosis and drug treatment by manipulating the expression of an enzyme in a particular region. For example, an increase in the concentration of an enzyme can lead to the transformation or degradation of the nanocarriers to release drug molecules.201–203
The controlled release profile of the delivered drugs can be directly observed in the living cells. Several researchers have used dye-labeling or radio-labeling approaches to track the release, location, and movement of drugs inside the cell.204–207 The pH-dependent release of doxorubicin (DOX) was reported, and the detachment of DOX from SWNTs was evaluated further by adopting a two-dye approach.204 For such applications, SWNTs were first labeled with fluorescein isothiocyanate (FITC), while DOX was also labeled separately with another red dye to track the location, movement, and release of the drug molecules from CNTs (within the cell by confocal microscopy). It was observed that the detachment of DOX from SWNTs took place in the lysosomes and was followed by the release of drug molecules into the cytoplasm and finally into the nucleus, while the SWNTs remained in the lysosomes.204 Peng et al.208 reported the sustained release of naproxen from a CNT hydrogel. To this end, CNT hydrogels were prepared by using chitosan and were characterized by scanning electron microscopy and Fourier transform infrared spectroscopy. Further, the performance of this CNTs hydrogel was compared against pure chitosan hydrogel in terms of controlled release efficiency. The results revealed a better-controlled release profile for CNT hydrogels than pure chitosan hydrogel; in addition, the releasing equilibrium time of the CNTs hydrogels was longer than that of a pure chitosan hydrogel.208
Bandyopdhyay and his group reported various research and review papers on the transdermal delivery of drugs such as anti-inflammatory drugs (diclofenac)209–211 and anti-hypertensive drugs (diltiazem)212 for diverse applications. Various polymers (e.g., carboxymethyl guar gum,209 2-hydroxyethyl methacrylate grafted carboxymethyl guar gum,210 and acrylic acid grafted guar gum211) have been utilized in combination with modified MWNTs for the sustained transdermal delivery of diclofenac. Further, the same research group recently used nanocomposites of poly(vinyl alcohol) modified MWNTs for the delivery of diltiazem.212 Likewise, diethylene glycol dimethacrylate-grafted carboxymethyl guargum and carboxy functionalized MWNTs have also been used for the sustained transdermal delivery of diclofenac. Sustained release of a drug from a nanocomposite was reported to be dependent on the concentration of MWNTs used in the formation of the nanocomposite. The controlled release patterns of the encapsulated drug were compared for two types of polymer/CNT composites prepared at two different concentration levels of MWNTs. Encapsulated drugs were more effectively released from the composite formulated with the higher quantities of MWNTs (42%) than that formulated with a lower concentration (16.4%).213
In addition to guar gum, bullfrog collagen was also used in combination with CNTs for the formation of hydrogels and was further employed for the sustained delivery of gentamicin.214 A polymethacrylic acid and CNT hybrid nanocomposite was synthesized as a potential models for the sustained release of quercetin; the cell viability assay (in vitro) was then evaluated to assess the toxicity of the conjugate.215 A pulsatile system was fabricated for programmable transdermal drug release of nicotine using CNTs and a hydroxyethylcellulose polymer.216 These authors concluded that controllable amounts of nicotine could be released upon the application of electric field over long durations. Such systems would not however be appropriate for the treatment of chronic diseases, wherein high concentrations of therapeutic agents are required within a short period. Servant et al.217 developed an electroresponsive polymer–CNT (poly(methylacrylic acid)–pristine MWNTs) hybrid hydrogel for pulsatile release of sucrose. Radio-labeled sucrose was used as a model hydrophilic drug for evaluating the controlled release profile under the application of an electric field. Mandal et al.218 also compared the performance of a biodegradable, biocompatible transdermal nanocomposite hydrogel (which was derived from carboxymethyl cellulose) and MWNTs for sustained release of diclofenac sodium. The polymer composite was a better alternative for the transdermal formulation, as it released diclofenac sodium in a sustained release profile.
3.2 Cancer diagnosis and treatment
Diagnosis and excision of a tumor at its proliferative phase are very tedious; in most cases, these tumors are asymptomatic. X-ray, X-ray-computed tomography scan (CT-scan), and magnetic resonance imaging (MRI) represent conventional techniques of cancer detection. They have been used to detect early morphological changes in neoplastic disorders, although none of them individually has the appropriate spatial resolution to detect such changes. Therefore, the aforementioned detection techniques are usually applied in combination with other techniques such as biomolecular markers, autofluorescence bronchoscopy, endobronchial ultrasonography, optical coherence tomography, confocal microendoscopy, single-photon emission computed tomography (SPECT), and positron emission tomography (PET). Among these options, PET and video-assisted surgery are good technologies for detecting various fluctuations in tissue biochemistry and metabolism at the molecular level, and they can, therefore, be used for imaging and treating disease.219 However, signals of increased metabolism do not necessarily indicate the presence of a tumor. Thus, it is imperative to develop novel tools and techniques for early cancer diagnosis.
Over the past few years, cancer diagnosis and treatment strategies using CNTs have expanded in terms of identifying, targeting and selectively irradiating the tumor area by adopting various methodologies. McDevitt et al.220 have reported tumor targeting CNT platforms that were fabricated from sidewall functionalized hydrophilic CNTs by covalently binding multiple copies of tumor specific antibodies, radiometal-ion chelates and fluorescent probes. In vivo biodistribution and pharmacokinetic studies have been carried out by different research groups using different CNT materials, different surface functionalizations, and different tracking methodologies.7,220–222
The degree of functionalization of CNTs is a crucial factor that affects their biological behavior. Wang et al.223 demonstrated that narrow MWNTs (average diameter of 9.2 nm) had enhanced tissue affinity, particularly for non-reticular endothelial tissues, as compared to wider MWNTs (average diameter: 39.5 nm). They concluded that the higher aspect ratio of narrow MWNTs might be advantageous in biological applications owing to higher tissue accumulation. Many researchers have actively been involved in diagnosing and delivering anticancer agents using CNTs because of their ability to independently cross the biological membrane through endocytosis. Numerous studies have been conducted toward in vitro and in vivo behavior of CNTs for drug release.224–226 CNTs have the potential to be easily functionalized by different groups for different applications, and they are capable of escaping from the reticuloendothelial system (RES). Appropriate functionalization can thus help in targeting, imaging, and real-time monitoring of drugs delivered to tumor cells.227–229
In a targeted drug delivery system, a CNT with a chemotherapeutic drug is functionalized with a tumor-targeted ligand that was first recognized by the specific receptors present in the tumor cell. Thereafter, the CNT–tumor targeted ligand complex attached to the receptor experienced internalization by the process of receptor-mediated endocytosis. Finally, the whole complex crosses the cell membrane. Inside the cell, the CNTs begins to release the chemotherapeutic drug to efficiently suppress the propagation of the tumor relative to the untargeted tumor cells.230,231 The whole mechanism of targeting cancer cells using CNTs as a carrier is illustrated in Fig. 5.
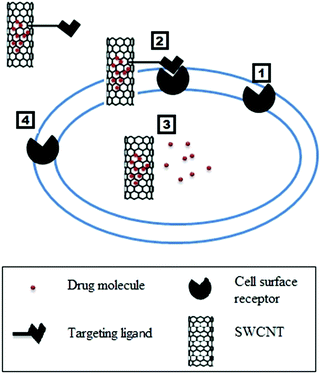 |
| Fig. 5 Cancer cell targeting using CNTs. Here, CNTs are functionalized with a targeting ligand for specific targeting followed by loading of anti-cancer drug molecules: (1) specific cancer cell receptor present on the cancer cell, (2) recognition and binding of the ligand-functionalized CNTs by the receptor, (3) internalization of CNT through a cell membrane and controlled release of anticancer drug, and (4) free receptor for new ligand recognition again. | |
There has been an attempt to internalize a platinum(IV) prodrug compound on PL-PEG-functionalized SWNTs (f-SWNTs).232 The whole complex was taken in by the cell through endocytosis and engulfed by endosomes. The release of platinum(II) proceeded from the complex due to the change in the pH inside the endosomes and the subsequent death of the cancer cells. It was also noted that pH-selective release of platinum(II) increased the cytotoxicity of the whole complex (platinum(IV)-PL-PEG-SWNTs) by over 100-fold compared to the single platinum(IV). Cancer cells have folate receptors (FR) on their surfaces. Hence, many studies have designed CNTs functionalized with a folic acid (FA) derivative so as to be recognized by the overexpressed FR receptors overexpressed on cancer cells and target only cancerous cells. Some investigators modified drug delivery strategies by entrapping the anticancer drug molecule in MWNTs functionalized with magnetic NPs.
In 2008, Yang et al.141 reported the delivery of chemotherapeutic agents into the pores of the MWNTs functionalized with magnetic NPs and with FA for targeted drug delivery to the lymphatic node. They observed that the MWNTs were retained in targeted drainage lymph nodes for several days in the presence of an external magnetic field and that the chemotherapeutic agent was released constantly to selectively kill tumor cells with FR on the surface. Note that folate was also applied as a homing device for the targeted delivery of an anticancer drug with the aid of SWNTs functionalized with FA; this approach was applied to directly to target human cancer cells through overexpression of the folate receptor (FR).233 The use of f-SWNTs with a conjugate between chitosan, a biodegradable polymer, and FA was also reported for the delivery of a chemotherapeutic agent (doxorubicin) based on a sustained release approach.234 The introduction of chitosan then led to a slow release of doxorubicin from an SWNT–doxorubicin–chitosan–FA conjugate compared to an SWNT–doxorubicin conjugate.
Along with chemotherapeutic agents, researchers also explored the delivery of nucleic acid (antisense oligonucleic acid and small interfering RNA (siRNA)) using CNTs for the treatment of cancer by silencing the expression of an oncogene or by blocking the cellular pathways.230 Zhang et al.135 reported the first silencing of telomerase reverse transcriptase (TERT) both in vitro and in vivo by conjugation of TERT-siRNA to SWNTs. Accordingly, they presented CNTs as a new class of material for the transportation of siRNA. Thereafter, numerous in vitro and in vivo studies have been performed to evaluate the potential of CNTs for the delivery of nucleic acid to silence the oncogene expression.235–238 Along with targeted drug delivery, CNTs exhibited excellent absorbance of near infrared (NIR) light; hence, SWNTs offer various types of useful functions for living cells through optical stimulation of nanotubes.239,240 Likewise, NIR light has been shown to heat the CNTs at the targeted site, which ruptures the cell nucleus and enhances tumor cell death without harming receptor-free normal cells.192,193 The details of this approach are provided below in the photothermal ablation therapy section. Table 1 summarizes various reports on anticancer drug delivery strategies based on CNTs.
Table 1 Summary of functionalized CNTs applied for the delivery of an anticancer drug
S. no. |
CNT material |
Release mechanism |
Biological studies |
Ref. |
(A) Doxorubicin (DOX) |
1 |
Carboxylated SWNTs (non-covalent approach) |
At low pH |
In vitro in HeLa cells with much more efficiency than free DOX |
241
|
2 |
Oxidized SWNTs functionalized with MAb, a fluorescent marker, and DOX (non-covalent approach) |
Acidic pH |
In vitro in colon cancer cells |
142
|
3 |
SWNTs (non-covalent approach) |
Enzymatic cleavage |
In vitro in murine melanoma cells and in vivo in mice |
143
|
4 |
SWNTs (non-covalent approach) |
NIR radiation |
In vitro in leukemia cells |
144
|
5 |
SWNTs (non-covalent approach) |
Acidic pH |
In vitro in glioblastoma cancer cells |
242
|
6 |
SWNTs conjugated with folic acid (non-covalent approach) |
At lower pH |
In vitro in breast cancer cells |
243
|
7 |
Dendrimer-modified MWNTs (covalent approach) |
At lower pH |
— |
244
|
|
(B) Cisplatin |
8 |
SWNTs (non-covalent approach) |
Reduction from Pt(IV) to Pt(II) due to endosomal acidic pH |
In vitro in testicular cancer cells |
232
|
9 |
SWNTs functionalized with FA (non-covalent approach) |
Reduction from Pt(IV) to Pt(II) due to endosomal acidic pH |
In vitro in choriocarcinoma, nasopharyngeal, and testicular cancer cells |
135
|
|
(C) Gemcitabine |
10 |
SWNTs (non-covalent approach) |
Magnetite NPs guided with an external magnetic field |
In vivo in Sprague-Dawley rats |
245
|
11 |
SWNTs (non-covalent approach) |
— |
— |
246
|
|
(D) Methotrexate |
12 |
MWNTs (covalent approach) |
Enzymatic cleavage |
In vitro in breast cancer cells |
145
|
13 |
MWNTs (covalent approach) |
— |
In vitro in T-lymphocytes |
247
|
|
(E) Paclitaxel |
14 |
SWNTs (non-covalent approach) |
Enzymatic cleavage |
In vitro and in vivo in breast cancer |
248
|
15 |
MWNTs (covalent approach) |
Acidic pH or enzymatic hydrolysis |
In vitro in lung and ovary cancer cells |
249
|
16 |
PEG-grafted SWNTs |
At pH 7 or 5 |
In vitro in HeLa and MCF-7 cancer cells |
250
|
|
(F) Carboplatin |
17 |
MWNTs (non-covalent approach) |
— |
In vitro in bladder cancer cells |
251
|
|
(G) Camptothecin |
18 |
MWNTs (covalent approach) |
Enzymatic cleavage |
In vitro in gastric carcinoma cells and in vivo in hepatoma-bearing mice |
252
|
|
(H) Curcumin (Cur) |
19 |
SWNTs (non-covalent approach) |
— |
In vitro in PC-3 cells; improved inhibition efficacy of SWCNT-Cur compared to that of native curcumin |
253
|
|
(I) Tamoxifen (TAM) |
20 |
Asparagine–glycine–arginine (NGR) peptide-modified SWNTs (non-covalent approach) |
— |
In vitro in 4T1 cancer cells; higher targeted and treatment efficiency than TAM alone |
254
|
3.3 CNTs as carriers for peptides, proteins, and genes
Peptides, proteins, and genes are macromolecules that can be easily degraded by enzymes present on the surface of a cell or inside the cell. Therefore, these biological macromolecules can be enclosed in such a carrier that can cross the biological barriers present on the cell surface without any loss of functionality. This can be done with the help of gene therapy for the transferal of genetic material used to replace the damaged cell and/or to repair the diseased portion. To this end, viral and non-viral vectors are needed to transfer the genetic material across the biological membrane. Endocytosis includes various pathways: phagocytosis (cell eating), pinocytosis (cell drinking), clathrin-mediated endocytosis (receptor mediated), and caveolae or the lipid-rafts pathway (clathrin independent).255 Clathrin-mediated endocytosis occurs when extracellular species are recognized by receptors to produce a clathrin coat on the membrane. This leads to invagination of the cell membrane so that extracellular species are finally trapped within the clathrin-coated vesicles for internalization.255,256
In case of caveolae (i.e., the lipid-rafts pathway), invagination of the cell membrane takes place through the enrichment of cholesterol and glycolipids. CNTs are unique in that they can traverse the cell membrane without perturbing the membrane, and they can thus facilitate the internalization of macromolecules into living cells. Therefore, nanotubes have been studied as probable non-viral nanocarriers for various kinds of drug moieties,129 genes,131,132 proteins,136 and peptides.137 Note that needle-like CNTs easily accommodate a variety of therapeutic materials on their surfaces for delivery into the target cell. Conjugated CNTs (SWNTs and MWNTs) having different therapeutic agents can be exposed to the endothelial cell, and the complexes can potentially treat the endothelial cells in a dose-dependent manner. For example, Walker et al.257 reported the dose-dependent effects of SWNTs and MWNTs on human aortic endothelial cells. They observed no sign of cytotoxicity for lower concentrations of SWNTs and MWNTs (0.04–0.4 microgram ml−1), whereas they observed considerable cytotoxicity for higher concentrations of SWNTs and MWNTs (1.5–4.5 microgram ml−1).257 If the concentration of CNTs exceeds the permissible limits, it may lead to cell death258 and apoptosis259 and may prevent cell proliferation.160
To explore the possible use of CNTs as a novel carriers for genes, the physicochemical interactions between DNA and cationic functionalized CNTs (f-CNTs) were investigated thoroughly.260 To this end, the researchers utilized three types of functionalized CNTs, i.e., ammonium-functionalized SWNTs, ammonium-functionalized MWNTs, and lysine-functionalized SWNTs with plasmid DNA. The results of their experiments revealed that all three different functionalized CNTs led to higher gene expression than naked DNA in human and murine cell lines. They also demonstrated that, of those three types of CNTs, ammonium-functionalized SWNTs had a greater capability to penetrate human and murine cells and provided enhanced gene expression. Shi Kam et al.133 investigated the utility of SWNTs for the transportation of various types of proteins (≤80 kD) that can adhere covalently but non-specifically to the sidewalls of the nanotubes. These authors revealed that transportation of the conjugate was achieved via receptor-mediated endocytosis. Along with delivery, SWNTs can help protect the DNA probe from intracellular enzymes that cleave the biological molecule, as reported by Gao et al.261 These authors demonstrated the delivery of the green fluorescent protein (GFP) gene into cultured human cells with the help of MWNTs functionalized with an amino group, and they demonstrated that the DNA probe was protected. Recently Spinato et al.238 reported the direct conversion of the carboxylic groups present on oxidized MWNTs into amines, and they used modified, aminated, oxidized MWNTs for the delivery of siRNA. Further, cellular uptake studies have also been performed to monitor the delivery capability of nanotubes. Table 2 documents the various reports on the CNT-mediated delivery of biomolecules.
Table 2 Various reports on CNT-mediated delivery of proteins, peptides, and genes
S. no. |
CNT-mediated delivery |
Ref. |
(A) Proteins |
1. |
• Uptake by clathrin-dependent endocytosis
• Intracellular transportation by protein–SWNT conjugates
|
133
|
2. |
• Streptavidin (SA), protein A (SpA), bovine serum albumin (BSA) and cytochrome c (cyt-c)
• Uptake by caveolae or the lipid-rafts pathway
|
262
|
|
(B) Peptides |
|
• α-Subunit (αs) of the Gs protein conjugated with amino-modified SWNTs
• Tagged with FITC
|
136
|
|
• Wrapping of SWNTs with polycationic and amphiphilic peptides [H-(-Lys-Trp-Lys-Gly-)7-OH]
• SWNT composite further modified with PEG
• Seven-fold higher uptake as compared with the SWCNT–peptide composite without PEGylation
|
263
|
|
(C) Plasmid DNA |
5 |
• Nickel-embedded SWNTs linked with plasmid DNA |
264
|
6 |
• Delivery of plasmid DNA by functionalized CNTs |
260 and 262
|
7 |
• SWNT-modified DNA probe to target a specific mRNA in the cell
• Increased self-delivery capability
• Increased bio-stability intracellularly
|
265
|
8 |
• Chemical conjugation of the carboxylated MWNT to the cationic polymers polyethylenimine (PEI) and polyallylamine (PAA) for the transfer of plasmid DNA (pCMV-βGal) |
266
|
9 |
• Carboxylated MWNT used for the delivery of plasmid DNA |
267
|
|
(D) Genes |
10 |
• Hybrids of SWNT–RNA polymer poly(rU)
• Negligible cytotoxicity to breast cancer cells (MCF7)
|
268
|
11 |
• Intracellular transfer of the GFP gene |
269
|
12 |
• Polyamido amine dendrimer-coated MWNTs used for the delivery of c-myc oligonucleotides
• Labelled with FITC for tracking the conjugates
|
270
|
13 |
• Magnetic CNTs bound to 1-ethyl-3-(3-dimethylaminopropyl) carbodiimide hydrochloride (EDC) for GFP plasmid delivery |
271
|
14 |
• PEG- and PEI-covalently modified SWNTs attached with 5TR1 aptamer
• 8.5–10 fold increase in transfection activity
|
272
|
|
(E) siRNA |
15 |
• Conjugation of telomerase reverse transcriptase-siRNA to SWNTs for the delivery of siRNA |
135
|
16 |
• Functionalization of SWNTs with cleavable disulfide bonds for efficient delivery of siRNA
• Controlled delivery of siRNA
• Achieved potent RNAi activity
|
273
|
17 |
• Delivery of siRNA using SWNTs (+) into antigen-presenting cells
• Possibility of in vivo immunotherapeutics
|
274
|
18 |
• PEI-functionalized CNTs for the delivery of siRNA |
275
|
19 |
• Amino-functionalised MWNTs for the delivery of siRNA
• Confirmation by cellular uptake studies
|
276
|
20 |
• SWNTs/siRNA nanoplex for the delivery of siRNA for gene therapy of pancreatic cancer
• Pure siRNAs incapable of transfecting cells as they are negatively charged and degraded in biological fluids
|
277
|
21 |
• Cationic MWNT-NH3+ used to deliver the apoptotic siRNA against polo-like kinase (siPLK1) in Calu6 tumor xenografts by direct intratumoral injections
• Improved therapeutic efficacy observed in the f-CNT-based siRNA delivery system as compared to single siRNA was established for the first time
|
278
|
3.4 CNTs as delivery devices for antigens and adjuvants
New and innovative delivery systems are being developed for the effective administration of vaccines and immuno-therapeutics. CNTs have emerged as a promising choice among the existing delivery systems because they can be easily functionalized and easily taken up by several cells in the immune system (monocytes, macrophages, T-cells, B-cells, and natural killer (NK) cells) with fewer cytotoxic effects.226,279–281 The nano-particulate nature of CNTs allows them to offer advantages in terms of controlled and targeted drug delivery, e.g., delivery of peptide antigens and adjuvant vehicles.282–284 Note that CNTs are also effective at delivering vaccines as they can activate the cells of the innate immune system.279–280,282 Both functionalized and non-functionalized CNTs can be used to activate genes (interleukin-1β (IL-1β), IL-6, and tumor necrosis factor-α (TNF-α)) directly or indirectly present on immune cells, and they effectively increase the expression of genes related to oxidative stress and apoptosis.285,286 The mechanism of vaccine delivery by CNTs involves the coupling of the antigen molecules onto the surfaces of CNTs to produce antibodies or immunocompetent cells.283,284 Therefore, CNTs can deliver antigens to produce adequate responses.282
3.4.1 Interaction of peptide antigens.
A protein antigen includes a region with a specific amino acid sequence and a B-cell epitope on the surface. These peptide antigens can be used as immunogens to induce specific B- and T-cell responses (CD4+ or cytotoxic CD8+). Then, CD4+ T-cells can indirectly activate B-cells to produce antibodies that neutralize viruses and bacterial toxins.287 The main role of CD8+ T-cells is to act as effector cells for the removal of infecting viruses.288 In this manner, lymphocytes regulate the immune response as depicted in Fig. 6. These peptide antigens are attached to f-CNTs to improve the cellular uptake and response time for the immune reaction.
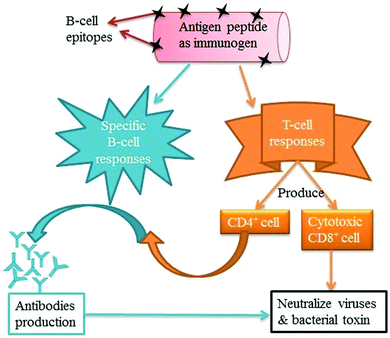 |
| Fig. 6 Regulation of immune response by lymphocytes. Immunogens activate the B- and T-cell response to directly produce antibodies and CD4+/D8+ cells, respectively, for neutralization of viruses and bacterial toxins. | |
Pantorotto et al.289 evaluated the immunological properties of the CNTs functionalized with peptide for vaccination. For this purpose, the antigen peptide from the foot-and-mouth disease virus (FMDV) was conjugated with SWNTs. The enzyme-linked immunosorbent assay (ELISA) was performed to check the efficiency of the peptide–CNT conjugation. Accordingly, the peptide–CNT conjugate was recognized by polyclonal and monoclonal antibodies generated against the peptide. The results of surface plasmon resonance (SPR) analysis indicated that the epitope structure of the peptide retained its antigenic form after conjugation to the CNTs, implying that the CNTs did not decrease the antigenicity of the peptide. As recognized using both ELISA and SPR analysis, the peptide–CNT conjugate should adopt the correct secondary conformation for antibody recognition. After that, the same research group developed a CNT–peptide conjugate for immunogenic efficacy. A bifunctional linker was used for the covalent attachment of the amine-functionalized CNTs with a B-cell epitope of the FMDV. The CNT–peptide conjugate then responded like an antigen, which can be recognized by specific antibodies. This immunogenic feature was further verified in mice immunized with an FMDV peptide–nanotube conjugate, which provoked a high immunogenic effect relative to the naked peptide.284 The covalent conjugation of Wilm's tumor protein (WT1) peptide to the SWCNTs was carried out and used for delivery to antigen presenting cells (dendritic cells and macrophages).282 The immunogenic efficiency of the peptide–SWNT conjugate was more efficient for inducing humoral immune responses than the plain antigens when evaluated both in vitro and in vivo.282
3.4.2 The CpG motif adjuvant.
Adjuvants are immune-stimulating molecules that provoke an immune response when combined with vaccine antigens, although they cannot provide immunity for themselves.290 For example, immune-stimulatory oligodeoxynucleotides (ODN) containing the CpG motif (deoxycytidyl-deoxyguanosine) (CpG–ODN) induce the production of various chemicals in the cell either directly or indirectly. Chemicals such as cytokines and cells such as B cells, monocytes, and helper T-cells are indirectly induced, while NK cells and dendritic cells are directly activated through toll-like receptor 9 (TLR-9), leading to enhanced T-cell responses.285,290 Speiser et al.291 reported the synthetic ODN, i.e., CpG 7909, as an effective vaccine adjuvant that induces the human innate immune response by generating antigen-specific CD8+ T cells. Fig. 7 depicts the schematic pathway through which the immune response is generated in the body by CpG–ODN. CpG–ODN directly triggers the toll-like receptors (TLR) and cytokine secretion. As CpG–ODN indirectly stimulates B-cell proliferation for antibody secretion, it can also activate antigen-presenting cells (APCs), which in turn can stimulate co-stimulatory molecules. The secreted cytokines (interleukin (IL)-12 and tumor necrosis factor (TNF)-α) promote the production of interferon (IFN)-γ by NK and T cells to further boost the proliferation of antigen-specific T cells and the differentiation of naive T cells.
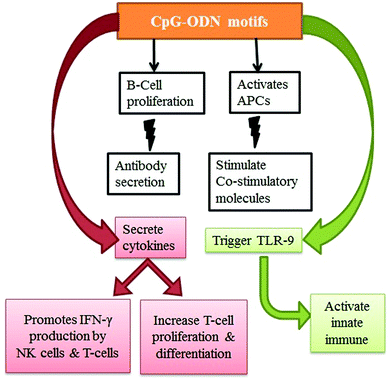 |
| Fig. 7 Generation of the immune response via the CpG–ODN motif. CpG–ODN motifs directly trigger TLR-9 and cytokines which in turn promote the production of interferon with the proliferation and differentiation of T-cells. CpG–ODN indirectly stimulates B-cells and APCs for the release of antibodies and costimulatory molecules for the regulation of immune response in the body. | |
Along with its potential advantages, CpG–ODN has a major disadvantage in that it has a short window of activity. Specifically, CpG–ODN alone showed a low ability to cross the cell membrane and low physiological stability. The application of CNTs toward the delivery of CpG–ODN offers exciting opportunities in the medical field. The existing literature had demonstrated an enhancement in the immuno-stimulatory properties140,292 when immunostimulatory CpG–ODN conjugated with CNTs is utilized. The conjugate (CpG–ODN–CNT) was more effective than free CpG–ODN in the cell uptake of CpG,276 and it also induced antitumor immunity.293
4. Advancements in CNT technology for biomedical applications
The many advantages of CNTs (e.g., large surface area, electronic transportability, catalytic activity, and ease of surface modification) have prompted the development of various sensing systems to detect analytes and/or biological species (proteins, peptides, DNA, RNA, etc.), as discussed below. The unique optical properties of CNTs also allow them to be used in a variety of biomedical applications. CNTs have excellent optical properties because they have strong optical absorption in the NIR-I window (750–1000 nm) which leads to photoacoustic imaging and photothermal ablation in situ.294 Along with optical absorption, these tubular structures also exhibit fluorescence emission in the NIR-II window (1000–1700 nm), which allows their use as fluorescent contrast agents for deep tissue fluorescence biological imaging applications.294 Further, multiplexable microarray technology has been used in combination with isotopically labeled CNTs to provide simultaneous multi-color detection of multiple analytes.295 It was also demonstrated that CNTs are capable of providing a versatile, multifaceted platform for a broad range of biomedical applications including cancer diagnosis and therapies.296–299 The use of CNTs has also been expanded to photoacoustic imaging, photothermal ablation therapy, sensing, the point of care devices, microbial treatment, bone scaffolds, and protein separation, as discussed below.
4.1 Photoacoustic imaging
Photoacoustic imaging is a new imaging technique to provide enhanced spatial resolution to visualize deeper tissues through the production of acoustic signals from light-absorbing molecules in biological systems. Zerda et al.150 first took photoacoustic images in a tumor mouse model, using SWNTs as the contrast agent. This work introduced a new platform for advanced research in the field of diagnosis. Indeed, SWNTs may allow biological imaging of deeper tissues with higher spatial resolution. These developed images were reported to have eight times better resolution than other images taken with traditional optical imaging techniques.300–308 Some diseases may not exhibit natural photoacoustic contrast early on. Therefore, a photoacoustic contrast agent must be introduced for imaging purposes. A number of contrast agents for photoacoustic imaging have been suggested and applied by various researchers.149,309,310
The major problem associated with contrast agents is targeting the diseased tissue. However, this problem can be overcome by conjugating a specific contrast agent with SWNTs. For example, Xiang et al.311 designed antibody-functionalized SWNTs for photoacoustic molecular imaging to specifically target an early stage tumor. Kim et al.312 reported the growth of gold nanoparticles (Au NPs) on the surface of CNTs and utilized this conjugate as a photoacoustic contrast agent for in vivo imaging and for killing cancer cells via a photothermal effect. To achieve the targeted effects, gold nanoparticles were grown on the surface of SWNTs, which were then functionalized with PEG-modified FA. This SWNT–Au–FA conjugate served as a strong imaging probe for the detection of cells with FR on their surface to allow photothermal ablation of cancer cells upon exposure to NIR.313 Other researchers also reported the coating of inorganic nanoparticles on the surface of nanomaterials in which the conjugate was used for drug delivery, fluorescence bio-imaging, and photothermal therapy.314,315 The efficacy of using a nanotube complex for imaging and photothermal/photodynamic therapy was tested in vitro and in vivo using a conjugate of indocyanine green (ICG) tagged to hyaluronic acid nanoparticles (HANPs) encapsulated with SWCNTs.316 The nanotube complex had no apparent systemic and local toxic effects on mice and maintained its thermal stability upon exposure to NIR during photoablation therapy. Recently, Xie et al.317 also reported a nanotube-based delivery system capable of providing fluorescence and photoacoustic imaging of tumors for effective tumor ablation therapy.
4.2 Photothermal ablation therapy
Photothermal ablation therapy was achieved by heating nanotube structures due to the absorption of light by the CNTs.318 CNTs have a strong optical absorbance in the NIR region.183,205 This optical property of CNTs has been investigated for the destruction of cancer cells using a photothermal effect.318–330 For example, Kam et al.331 reported the utilization of a DNA-coated SWNT–FA conjugate on HeLa cells for the destruction of cancer cells only by NIR-triggered photothermal ablation without harming receptor-free normal cells. They concluded that this destruction of cancer cells occurred due to the intrinsic optical properties of CNTs. Optical coupling of light with CNTs is expected to reach a maximum when nanotube lengths are at least half the wavelength of the incident light beam based on the antenna theory.332 Surface defects (dopants) in the structure of the MWNTs can be used to improve the antenna properties of the nanotubes. These defects can cause scattering of currents which, in turn, decreases the heating time but increase the rate of heating of the nanotube. The density of such defects can be controlled by the amount of dopants (e.g., nitrogen) added into the carbon lattice. This N-doped photothermal ablation approach was explored by Torti et al.320 They attempted to destroy kidney cancer cells using NIR irradiation of N-doped MWNTs for photothermal ablation therapy. The length of the CNTs was found to be a critical variable in determining the heat transfer ability of the nanotube, and nanotubes between 700 and 1100 nm long were optimal for heat absorption and destruction of tumor cells.320 Unfortunately, however the photothermal destruction may not be specific for cancer cells due to limited ability to penetrate into deep tumor areas.
Functionalization of CNTs with targeting moieties (e.g., FA or inorganic nanoparticles) is a potential solution to the problems mentioned above. Examples of this are found in Section 3.2 Cancer diagnosis and treatment and Section 4.1 Photoacoustic imaging. Different researchers studied CNT-based photothermal ablation of cancer cells using various targeting peptides or antibodies conjugated with CNTs.333–335 For example, Chakravarty et al.333 developed an SWNT-FA modified by a PEG conjugate for the targeted photothermal destruction of cancer cells with FR on the surface. In 2009, four different research groups almost simultaneously demonstrated the effectiveness of in vivo photothermal ablation therapy based on CNTs. For instance, a folate-bearing nanotube (0.81 nm in size and a maximum NIR absorbance at 980 nm) was successful in the photothermal destruction of cancer cells under both in vitro and in vivo conditions when applied for ablation therapy.321 Burke et al.324 reported that (like SWNTs) MWNTs exhibited a strong NIR absorbance. They suggested that MWNTs functionalized with pluronic F-127 had ablative properties when injected into tumor cells after exposure to 1064 nm laser irradiation for 30 s. In another work, Ghosh et al.325 observed that a DNA-coated MWNT conjugate injected into PC3 xenograft tumors completely ablated the tumors when irradiated with a 1064 nm laser for 70 s.
A photothermal effect for the destruction of cancer cells was demonstrated by using PEGylated SWNTs through irradiation with an 808 nm laser.336 The photothermal destruction of tumor cells can be further enhanced by the use of MWNTs functionalized with antibodies (f-MWNTs-abs) by inducing a photothermal effect. In this context, Chou et al.329 suggested that conjugation of antibodies for BT-474 cancer cells with f-MWNTs (f-MWNTs-abs) have higher cell specificity compared to using antibodies alone. Moreover, such applications improved the ability to kill cancer cells compared to normal f-MWNTs upon exposure to NIR light. However, it is difficult to destroy tumor cells using photothermal ablation if the heat is distributed unevenly to the affected area. Therefore, some researchers proposed the combination of photothermal ablation and chemotherapy to enhance antitumor activity by both photothermal cell destruction and drug release.
The use of multifunctional CNTs might reduce the toxic effects of chemotherapy.192,193,243 The photothermal effect of SWNTs was pronounced when used in combination with the anticancer drug doxorubicin (DOX) for targeting and accelerated the destruction of breast cancer cells.243 This group synthesized DOX-loaded SWNTs conjugated with FA for targeted delivery, and the release of DOX was monitored for 3 days. After that, the breast cancer cells were exposed to laser radiation at 800 nm, and accelerated death rates of breast cancer cells were observed. The dual effect of gene therapy and photothermal therapy was also demonstrated by using a PEI-functionalized SWNTs as a carrier for siRNA, which was further conjugated with a peptide (NGR) for targeted delivery.330 The whole SWNT-PEI/siRNA/NGR complex significantly enhanced the therapeutic efficacy by combining RNAi therapy and photothermal therapy.
As stated above, multifunctional nanotube-based conjugates have been effectively employed for imaging as well as photothermal and photodynamic therapy.316,317 The surface modification of SWNTs was tested further with Evans blue (EB) and albumin coated paclitaxel for chemo/thermo therapy.337 The SWNTs/EB/albumin/paclitaxel nanocomplex exhibited long term stability (for weeks under certain physiological conditions) with strong NIR absorbance when administered into the MDA-MB-435 tumor; effective ablation of the tumor was observed by chemo and photothermal therapy.337 Recently, a technique involving the attachment of Cu2−xSe to the CNT surface was also evaluated for photothermal ablation efficiency against tumor cells.338
4.3 Nanoprobe sensing applications
One of the most important applications for CNTs is nanoprobe sensing. CNT-based probes have been used in a variety of applications including nanoelectrodes, nanosensors, high-resolution structural imaging, nano-lithography, field emitters, and drug delivery. In order to achieve high resolution imaging, a single MWNT was attached to the tip of a scanning probe microscope (SPM). The highly conducting nature of CNTs makes them useful in scanning tunneling microscopy (STM), atomic force microscopy (AFM), and other scanning probe instruments.339,340
Conventionally used silicon, silicon nitride, and other metal tips are brittle. They tend to have a large radius of curvature, and their lateral resolution is therefore generally limited. CNT-based tips on a pyramidal AFM probe341,342 were thus developed to overcome such limitations. These CNT-based tips have high elasticity, small end radii of 5–12 nm,343 a high aspect ratio,343,344 and selective functionalization with any moiety.345–351 In addition, the probes do not crack when making contact with the substrate molecules due to the high elasticity of the nanotubes. CNT probe tips are capable of imaging biological molecules such as DNA with much higher resolution than conventional STM probe tips.352 Wong et al.352 reported the first use of SWNTs and MWNTs for imaging amyloid-b-protofibrils with high resolution. The smaller size and agglomeration behavior of the nanotubes pose challenges to mounting individual CNTs on the tips of SPMs. Bundles of nanotubes are first mounted onto the AFM tip, and the tip ends are thereafter sharpened by cleavage to expose individual CNTs. The attachment and alignment are not yet controllable, and nanotube agglomeration ultimately introduces a secondary oscillation that leads to a loss in imaging viability. To resolve this problem, many attempts have been made to grow individual nanotubes directly on the tips using a CVD method; this approach indeed produced thin individual MWNT tips, which were used to acquire images of biological structures with high resolution.353,354 Therefore, CVD has the potential to be used for the commercial production of tips.
CNTs can also be used as a molecular probe through the chemical modification of a functional group. As such, they have the potential to be applied in the fields of chemical force microscopy, diagnosis, and treatment. Specific functionalized CNTs have been used as AFM probes to measure the interactions or binding forces between two molecules such as protein–ligand or antigen–antibody. Thus, depending upon the chemical functionalization of the CNT probe, they can be used in various applications including drug delivery, imaging of interactive chemical patterns, and molecular recognition.355–359
4.4 Point-of-care devices
Point-of-care (POC) testing assumes the availability of better care and rapid treatment options at or nearby the site of the event. Global healthcare practices have been constantly improving through the development of POC technologies. Some examples of POC sensors used for healthcare purposes include (1) Stat strip Gluc for glucose, (2) Stat strip Lac for lactate, (3) Stat sensor Creat for creatinine developed by Nova Biomedical, (4) i-STAT, a bedside blood testing biosensor developed by Abbott Point Of Care, (5) Guardian real-time system for glucose sensing using screen printed electrodes developed by Medtronic (SPE), (6) AC1.AChE for acetylcholinesterase, (7) AC1.GOx for glucose, and (8) AC1.W1.R1 for dopamine developed by Palm Sens.360
CNT-based biosensors have extraordinary sensitivity with fast electron-transfer properties to allow their use in the development of versatile tools for diagnosis. Such development is expected to have a significant impact on the healthcare sector, environmental monitoring, and food product analysis. The fast electron transfer reactions that occur within CNTs also help enhance transducer sensitivity for viral disease therapy361 and viral diagnosis;362 they are thus promising for the development of a rapid detection method of human and animal pathogens. Depending on the type of analytes, biosensors can be classified into enzyme biosensors, gene biosensors, and immune biosensors. Biosensors can be classified based on the transducer such as optical, piezoelectric, electrochemical, or thermometric. Of these options, electrochemical biosensors have been more successfully applied than the other techniques with regard to successful commercialization. For the fabrication of sensors, CNTs functionalized with biological elements are directly attached to the electrode to enhance the electrochemical response and to amplify the signal.
4.4.1 Enzyme biosensors.
The most common biosensor used is the glucose biosensor. A CNT-based glucose sensor was first developed by incorporating CNTs with glucose oxidase (GO) via a Teflon binder.363 Thereafter, several modifications were employed to further improve the sensitivity of such a biosensor.364–376 There was also an effort to replace its electrode with a screen-printed CNT.377 The performance of these biosensors was assessed by coating CNTs with one layer of enzyme250 or multiple layers of enzyme378 by a layer-by-layer attachment process. A schematic view of a CNT-based glucose biosensor is provided in Fig. 8 in which negatively charged CNTs interact with a cationic poly(diallyl dimethyl ammonium)chloride (PDDA) layer and glucose oxidase (GO) immobilized on CNTs, followed by one more layers of PDDA with a sandwich-like structure (PDDA/GO/PDDA/CNT/PDDA/GO/PDDA). This structure helped preserve the bioactivity of the enzyme, while preventing leakage.365 Recently, Manikandan et al.379 have used zinc oxide, MWNTs and poly(vinyl chloride) for the fabrication of a ternary composite which was deposited on a glassy carbon surface for the direct sensing of glucose. These biosensors have been used to monitor the blood glucose in people with diabetes. In addition to the glucose biosensors, CNTs have also been used for the detection of other biological analytes including fructose,380 galactose,381 neurotransmitters (i.e., dopamine,382–385 acetylcholine,386–388 epinephrine,389,390 and norepinephrine391), amino acids (i.e., glutamic acid,392,393D-amino acids,394 and L-amino acids395), immunoglobulins (i.e., IgG396,397 and IgE398), albumin,399 insulin,400 human chorionic gonadotropin (HCG),401,402 C-reactive protein (CRP),403 cancer biomarkers,404,405 microorganisms,406,407 DNA,408,409 and other biomolecules (e.g., uric acid,410,411 ascorbic acid,412,413 cholesterol,413,414 folic acid,415,416 and NADH417).
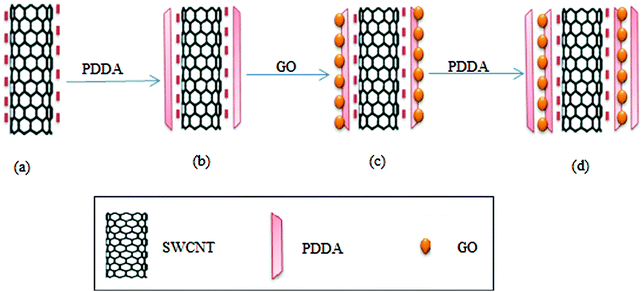 |
| Fig. 8 CNT-based glucose biosensor. PDDA and GO immobilized on the negatively charged CNT by the layer-by-layer synthesis technique for glucose biosensing: (a) negatively charged CNT surface, (b) PDDA immobilized on CNT, (c) GO immobilized on PDDA attached CNT, and (d) sandwich-like layer structure (PDDA/GO/PDDA/CNT/PDDA/GO/PDDA). | |
Along with screen-printed electrochemical biosensors, CNT-modified screen-printed amperometric biosensors have also been used as a rapid assay of organophosphorus (OP) compounds.418 Such modified screen-printed carbon electrodes were subsequently co-immobilized with acetylcholinesterase (ACHE) and choline oxidase (ChO) enzymes. This biosensor possesses a significant catalytic effect on the generation of hydrogen peroxide (H2O2), a product of the redox reaction. Hydrogen peroxide produced after catalysis of choline by choline oxidase was amperometrically detected by the CNT/ACHE/ChO biosensor; this amperometric response was inversely proportional to the amount of the OP compound introduced into the system. The basic principle for the detection of OP compounds through amperometry is depicted in Fig. 9. Other researchers have also used this approach for the detection of paraoxon.419,420
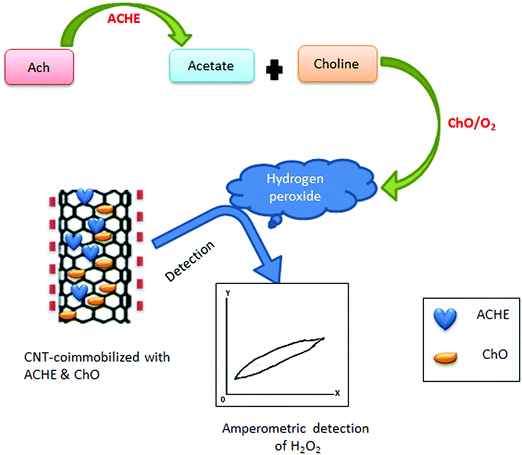 |
| Fig. 9 Schematic representation of the amperometric detection of OP compounds. For such applications, ACHE and ChO are immobilized on the CNT surface for the fabrication of CNT-modified screen printed amperometric biosensors. These biosensors can be used to detect the concentration of H2O2, which is in negative proportion to the concentration of the OP compounds. If the detected concentration of H2O2 is high, it implies that there is a low amount of the OP compounds. On the other hand, if H2O2 is low, then there is a high amount of the OP compounds [ref. 418]. | |
4.4.2 DNA biosensors.
DNA biosensors have been developed to test both genetic and infectious types of diseases because their use is very simple, rapid, and cost effective. In CNT-based electrochemical DNA biosensors, a single-stranded (ss-) DNA probe sequence is immobilized on the surface of a CNT-modified electrode (transducer surface) that can produce transduction signals via the formation of a duplex (Fig. 10).421,422 In some cases, the hybridized form requires a label for proper detection, viz., an enzyme, fluorophore, magnetic beads, or radioactive compounds. The labeling step is not mandatory, although it can enhance the sensor sensitivity.423,424 A CNT-based nanoelectrode array has also been employed for ultrasensitive detection of DNA.398 A vertically aligned MWNT electrode insulated with SiO2 was constructed and functionalized further by carbodiimide; this probe DNA was then attached for the detection of hybridization. To improve the sensitivity of CNT-based electrochemical DNA biosensors, various types of metal NPs (e.g., gold,425–427 silver,428 platinum,429 zinc,427 and nickel429) have been devised in combination with CNTs.
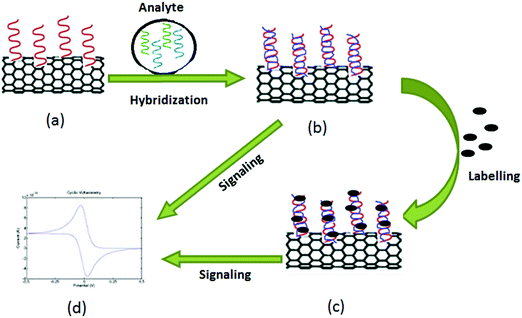 |
| Fig. 10 Schematic layout for DNA detection with the help of the CNT electrode: (a) immobilization of the probe on the CNT electrode, (b) hybridization of the analyte on the probe, (c) labeling of the hybridized probe with the enzyme or fluorophore, and (d) detection of signals by cyclic voltammetry. | |
4.4.3 Cancer biosensors.
Cancer is caused by the proliferation of abnormal and uncontrolled cells that leads to death caused by damage to various organs. Cancer-specific biomarkers such as DNA, RNA, or proteins exist in a bodily fluid, and these can be valuable in the early diagnosis and treatment of cancer. In fact, many types of cancer biomarkers have been evaluated. However, diagnosis of particular cancer types is not yet possible, as a single protein biomarker can be indicative of multiple diseases.430,431 A biosensing system can be used to improve the diagnosis of cancer screening at an early stage by employing integrated and automated procedures for rapid analysis. Every cancer cell generates specific cancer biomarker antigens (CBAs) for a specific type of cancer, such as prostate-specific antigen (PSA),432–434 prostate-specific membrane antigen (PSMA),435,436 carcinoembryonic antigen (CEA),437 interleukin 6 (IL-6),438,439 interleukin 8 (IL-8),439 platelet factor 4 (PF-4),440 cancer antigens (e.g., CA-125, CA-19-9),441,442 C-reactive protein (CRP),444 alpha fetoprotein (AFP),445 and miRNA (miR-15b*, miR-23a, miR-133a, miR-150*, miR-197, miR-497, miR-548b-5p and miR-181).446–448 These CBAs produce an interactive reaction when they come in contact with their associated antibody.
Devices for POC-based detection of CBAs must be robust, ultra-sensitive, easy to operate, and low in cost. POC devices for cancer detection must be accurate regarding the elevated concentration level of the target protein only with the potential to distinguish normal and diseased cells. Justino et al.448 described two possible options for a POC biosensing device for CBA identification. The principle behind both strategies was the same, i.e., the interaction of antigens with specific antibodies mounted on a CNT electrode layered by nafion produced a detectable signal.448 As a normal cell does not produce CBAs, it is not likely to interact with antibodies mounted on a CNT electrode layered by nafion; hence it will not produce a detectable signal.448 With regard to the interaction between antigens and antibodies, the current signal is generated and measured electrochemically and should be proportional to the concentration of antibodies bound to CBAs. Thus, one can easily distinguish between a normal cell and a cancer cell. Fig. 11 is a schematic representation of this principle for the detection of cancer.
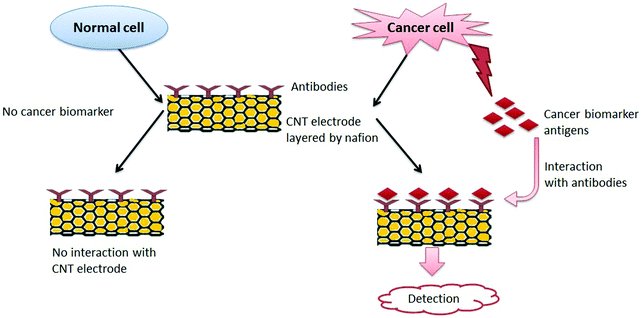 |
| Fig. 11 The mechanism for the detection of cancer by using cancer biosensors. A normal cell can be distinguished from a cancerous cell; as the former does not have a surface biomarker antigens, and it does not interact with the CNT electrode. On the other hand, the biomarker antigen present on the surface of the cancer cell interacts with the antibodies coated on the CNT electrode layered by nafion. Thus, their interaction has been illustrated in the form of a reaction. | |
A CNT-based electrochemical immunosensor was first applied for the detection of PSA in real biomedical serum samples and tissue lysates for the diagnosis of prostate cancer.449 The performance of the PSA detection (e.g., limit of detection (LOD)) was compared to a CNT-based immunosensor and the ELISA technique. The CNT-based immunosensor had a detection limit of 4 pg mL−1 (100 amol mL−1) for PSA in 10 μL of undiluted calf serum, which was highly sensitive with ±5% accuracy for human serum samples compared with the ELISA method.449 Similarly, numerous other strategies have been explored to develop a CNT-based cancer biosensor. For example, specific biomarkers have been immobilized on a chip for the detection of specific cancer cells, as listed in Table 3.
Table 3 Cancer biomarker detection biosensors based on CNTs
S. No. |
Electrode modification |
Detection technique |
Detection range |
Ref. |
(A) PSA |
1 |
SWCNT-Ab1-PSA-Ab2-HRP-biconjugate |
Amperometric immunosensor |
0.4–40 ng mL−1 (LOD 0.4 ng mL−1) |
449
|
2 |
SWCNT-Ab1-PSA-Ab2-RuBPY-silica |
Electrochemiluminescent immunosensor |
0.04–5 ng mL−1 (LOD 40 pg mL−1) |
450
|
3 |
MWCNTs-AuNPs-Ab1-PSA-Ab2-HRP |
Cyclic voltammetric immunosensor |
1.0 pg mL−1–10 ng mL−1 (LOD 0.4 ± 0.03 pg mL−1) |
451
|
|
(B) PSA-ACT |
4 |
CNTs-FET modified with solutions containing various linker-to-spacer ratios |
Field effect transistor biosensor |
1–100 ng mL−1 (LOD 1 ng mL−1) |
452
|
|
(C) IL-6 |
5 |
SWCNTs-HRP-Ab2 |
Amperometric immunosensor |
40–150 pg mL−1 (LOD 30 pg mL−1) |
453
|
6 |
SWCNTs-HRP-Ab2 |
Amperometric immunosensor |
0.5–30 pg mL−1 (LOD 0.5 pg mL−1) |
454
|
|
(D) PSA and IL-8 |
7 |
CNT-HRP-Ab2 (screen-printed carbon electrode) |
Amperometric immunosensor |
5 pg mL−1 of PSA and 8 pg mL−1 of IL-8 |
455
|
|
(E) Carcinoembryonic antigen (CEA) |
8 |
Monoclonal anti-CEA on PEI-wrapped MWCNT SPE |
Square wave voltammetry immunosensor |
5 × 10−12–5 × 10−7 g mL−1 (LOD 1 × 10−12 g mL−1) |
456
|
9 |
Gold nanoparticles (Au NPs) used to link the substrate materials and Ab1 and palladium NPs–vanadium pentoxide–MWCNTs used as the label Ab2 |
Electrochemical immunosensor |
0.5 pg mL−1 to 25 ng mL−1 (LOD 0.17 pg mL−1) |
457
|
|
(F) Alpha-fetoprotein |
10 |
Au NP/CNT-doped chitosan-modified glassy carbon electrode (GCE) |
Amperometric immunosensor |
1–55 ng mL−1 (LOD 0.6 ng mL−1) |
458
|
11 |
Prussian blue (PB) and AuNPs on MWCNT-modified GCE |
Amperometric immunosensor |
0.01–300 ng mL−1 (LOD 3 pg mL−1) |
459
|
|
(G) miR-141 |
12 |
Poly(JUG-co-JUGA)/o-MWCNT-modified electrode |
Square wave voltammetry immunosensor |
LOD 8 fM |
460
|
4.5 Antimicrobial treatment
CNTs were shown to have strong antimicrobial activity, as reported first by Kang et al.461 Functionalization of CNTs might increase the antimicrobial activity while helping to decrease the toxicity toward mammalian cells.462,463 A thin film of CNTs was functionalized with two polyelectrolytes, (poly(L-lysine) and poly(L-glutamic acid)).462 These authors reported significant increases in antimicrobial activity (up to 90%) toward Escherichia coli and Staphylococcus epidermidis. Similarly, a phospholipid functionalized with PEG (PL-PEG) was also observed to efficiently disperse SWNTs in an aqueous solution.463 An analysis of antimicrobial activity (toward Escherichia coli) indicated 90% inactivation of the microbes.463 However, the antimicrobial activity of CNTs was dependent on many factors. SWNTs were reported to be more toxic to bacteria than MWNTs, suggesting that the diameter of the CNTs affected antimicrobial activity.461 Moreover, the length of the CNTs also played a role in the strength of antimicrobial activity. According to Yang et al.,464 longer SWNTs exhibited a more pronounced concentration-dependent effect on microbial growth. In addition, MWNTs were more toxic when they were uncapped, debundled, and dispersed in the solution.461
The toxicity of CNTs was also affected by the extent of dispersion, as individually dissolved SWNTs were found to be more toxic to bacteria in comparison to aggregated CNTs.465 Functionalization of CNTs can effectively alter the surface properties to make them more dispersive or more easily dissolved in various solvents. In the case of fine dispersion, SWNTs were seen to act as ‘nano-darts’ so that the attacked bacterial cells were degraded until death.465Fig. 12 presents a schematic diagram of the antimicrobial mechanism of CNTs.
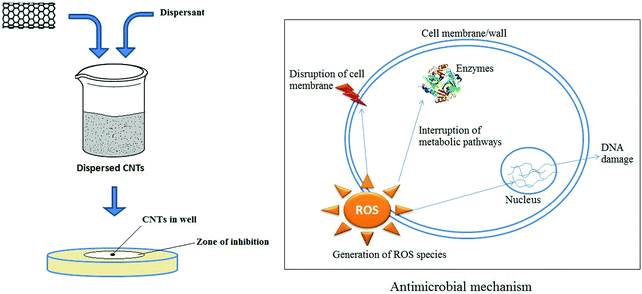 |
| Fig. 12 Schematic representation of antimicrobial activity by modified CNTs. | |
Covalent modification of CNTs with polylysine is also effective for dispersing CNTs. Improved dispersion has a pronounced effect on certain antimicrobes such as Escherichia coli, Pseudomonas aeruginosa, and Staphylococcus aureus.466 Similarly, functionalization with polylysine helped improve dispersion of CNTs to stimulate antimicrobial activity against three Gram-negative bacteria and three Gram-positive bacteria.467 As such, functionalized CNTs exhibited enhanced antimicrobial activity relative to pristine CNT.443 Functionalized CNTs can also be used as innovative and efficient carriers in transport and cellular translocation of amphotericin B.468 In that study, f-CNT–amphotericin B conjugates, amphotericin B, and dispersed amphotericin B were tested against fungal strains (Candida sp). The f-CNT–amphotericin B conjugates exhibited better antifungal activity than amphotericin B in its pristine form whether they were dispersed or not. Along with antifungal activity, this group also reported that the f-CNT–amphotericin B conjugate had no significant in vitro toxic effects.468 Another group confirmed that f-CNTs–amphotericin B conjugates were superior in performance to amphotericin B alone for anti-leishmanial efficacy.469 Surface-engineered CNTs may be able to capture pathogenic microbes in a liquid medium because microorganisms can be adsorbed onto the engineered surfaces of CNTs.470–473 Specifically, the utility of CNTs was thus demonstrated in the capture,472 removal,470,471 and inactivation of bacteria.471 Vecitis et al.471 described an MWNT filter to treat drinking water through the removal and inactivation of virus and bacteria.471Table 4 shows a summary of the applications of modified CNTs in antimicrobial treatment. A number of researchers have investigated the antibacterial activity of CNTs in paints474,475 and biofilms.476,477
Table 4 Antimicrobial activities of modified CNTs
S. no. |
Modification on CNTs |
Achievements |
Consequences |
Ref. |
1 |
Purified SWNTs and MWNTs |
SWNTs exhibit much stronger antibacterial activity against Escherichia coli than MWNTs |
Inhibition due to cell membrane damage and oxidative stress |
461
|
2 |
High purity SWNTs with an average diameter of 0.83 nm |
SWNTs have antimicrobial activities against Escherichia coli, Pseudomonas aeruginosa, Staphylococcus aureus and Bacillus subtilis |
Individually dispersed SWNTs were found to be more toxic than aggregated SWNTs; Gram(+) bacteria were more vulnerable to SWNTs than Gram(−) bacteria |
465
|
3 |
CNTs covalently functionalized with polylysine |
Antimicrobial activities against Escherichia coli, Pseudomonas aeruginosa and Staphylococcus aureus |
Dispersion enhanced the antimicrobial activity |
466
|
4 |
SWNTs |
Three different lengths of SWNTs were tested for antimicrobial activity |
Longer SWNTs had more pronounced antimicrobial activity |
464
|
5 |
f-CNTs conjugated with amphotericin B |
Antifungal activity against Candida spp. |
The f-CNT-amphotericin conjugate had a better antifungal effect than amphotericin alone |
468
|
6 |
CNTs covalently functionalized with polylysine |
Antimicrobial activities against three Gram(+) and three Gram(−) bacteria |
Properly dispersed, f-CNTs had greater antimicrobial activity than pristine, aggregated CNTs |
467
|
7 |
CNTs modified with poly(L-lysine) and poly(L-glutamic acid) |
Antimicrobial activity (up to 90%) toward Escherichia coli and Staphylococcus epidermidis |
Functionalized CNTs exhibited greater antimicrobial effects than pristine CNTs |
462
|
8 |
Phospholipid functionalized with PEG for CNT dispersion |
Antimicrobial activity (up to 90%) toward Escherichia coli |
Dispersed CNTs had better antimicrobial activity than aggregated CNTs |
463
|
9 |
MWNTs functionalized with mono-, di-, and tri-ethanolamine |
Antibacterial activity against four Gram(+) and four Gram(−) strains |
Functionalized MWNTs showed better antibacterial activity than pristine MWNTs |
478
|
10 |
MWNTs covalently functionalized with epsilon-polylysine |
Antibacterial activity against Escherichia coli, Pseudomonas aeruginosa and Staphylococcus aureus |
Deposited film of functionalized MWNTs increased antibacterial activity compared to bare MWNTs |
479
|
11 |
MWNTs functionalized with cationic amino acids arginine and lysine |
Antibacterial activity against Escherichia coli and Salmonella typhimurium |
The sequence of antibacterial activity was MWNTs-arginine > MWNTs-lysine > pristine MWNTs |
480
|
12 |
MWNTs modified with Ag and Cu NPs |
Antimicrobial activity against Escherichia coli |
Ag-MWNTs, Cu-MWNTs, and MWNT–COOH inhibited bacterial growth by 97%, 75%, and 20%, respectively |
481
|
4.6 CNTs in bone scaffolding
Bones are a strong and hard part of the body that exist in various shapes. Bones have two layers – a hard outer layer called compact bone and a soft inner part called cancellous or spongy bone. Bone is a composite material consisting of a fibrous protein, collagen, and hydroxyapatite along with some lipids, chondroitin sulfate, and keratin sulfate. Cells inside of bone are attached to the extracellular matrix (ECM) and are subject to proliferation, migration, and differentiation into a specific tissue. The potential use of CNTs has been explored in the field of tissue engineering as they can provide 3D scaffolds with close resemblance to the ECM. In this respect, the matrix plays an important role in tissue engineering, as it can provide space for engineered tissue to facilitate the development of biological tissue.
PLGA and PLA are the most popular synthetic polymers that have been used as materials for the formation of a matrix, although their mechanical strengths are not sufficiently high. The recent progress in tissue engineering has stimulated the development of bio-implants that are based on biocompatible nanomaterials. CNTs may be able to provide structural reinforcement for tissue scaffolding as they afford significant improvements in mechanical strength when a small fraction of dispersed CNTs is mixed with polymers.482 In order to increase the mechanical strength of the scaffold composites, CNTs have been incorporated into polymers,483–489 either natural or synthetic, which further increases the proliferation,487,490 differentiation,490,491 and electrical conductivity.492Fig. 13 depicts the formation of nanocomposite scaffolds using functionalized CNTs with polymers and their roles in various cell responses.
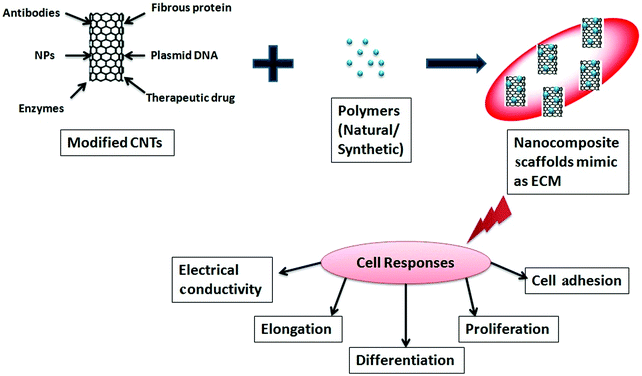 |
| Fig. 13 Schematic diagram describing the role of CNTs in the formation of scaffolds and in cell responses. | |
The growth of various cell types on CNT nanocomposites in vitro has been demonstrated successfully (such as smooth muscle cell growth supported by SWNTs with collagen,493 fibroblast growth supported by CNT scaffolds,493 and neuronal growth supported by the f-MWNT nanocomposite494,495). Likewise, SWNT and MWNT suspensions exhibited good biocompatibility with mesenchymal stem cells (MSCs).486 Further, CNT suspensions were found to have no adverse effects on MSCs. Instead, the proliferation and differentiation of MSCs were observed.
According to Sharma et al.,495 the fabrication of composite nanofibers [polyaniline-CNT/poly(N-isopropyl acrylamide-co-methacrylic acid)] and polymer nanofibers [poly(N-isopropyl acrylamide-co-methacrylic acid)] using the electrospinning method produced a suitable 3D tissue scaffolds. The cellular response of these nanofibers was then assessed with mouse L929 fibroblast cell lines; the composite nanofiber exhibited improvements in cell viability and cell growth rate relative to its pure form.496 CNT–PLGA composite scaffolds were also reported to have enhanced mechanical strength relative to simple PLGA scaffolds, while maintaining a higher rate of proliferation and differentiation than MC3T3-E1 osteoblast cells.490 As such, this nanocomposite also exhibited improvements in cell adhesion, growth, and viability relative to the use of nanofibers alone.466 In another study, an MWNT–PLGA nanocomposite scaffold made with the addition of 3% MWNTs exhibited significantly increased compressive strength and modulus (35 MPa, 510.99 MPa) relative to pure PLGA scaffolds (19 MPa and 166.38 MPa).497 The fabrication of a scaffold using CNTs with two types of polymers (e.g., hydroxyapatite (HA)/polycaprolactone (PCL)) was conducted by several groups. In particular, such modification provided better bioactivity, cell adhesion, and proper spreading of cells on the scaffold surface.498,499 The conductivity of CNTs also played a role in promoting osteoblast (bone-forming cells) activity. In addition, the functions of bone cells were also stimulated under an electrical current; hence, CNTs were suggested as the best nanofibers, and they have been used as a substrate owing to their electrical conductivity.491 The effect of fabrication conditions on the nanocomposites was also explored when using biodegradable PLGA in combination with carboxylated MWNTs in a solvent casting technique.500 These authors then examined the biocompatibility of these nanocomposites on rat bone marrow-derived mesenchymal stem cells (MSCs).500 The nanocomposite showed better cell adhesion and viability to promote the cell growth and differentiation of MSCs into osteoblasts.500
Third-generation scaffolds are being developed to achieve parameters such as proper cellular attachment, spreading of cells, proliferation, differentiation, biocompatibility, mechanical strength, controlled bio-resorbability, polarization, angiogenic cues, and vascularization.501 Therefore, CNT composite nanofibers developed in combination with natural or synthetic polymers should be capable of offering an improved 3D scaffold with their advanced features. These CNT composite scaffolds can thus provide an anchorage for cells on the scaffold surface, and stability for proliferation to initiate the desired process of differentiation, elongation, and conductivity.
4.7 CNTs in protein separation
Because of selective adsorptive power toward biomolecules, CNTs can be employed for the separation of biomolecules such as DNA and proteins.502–504 Wang et al.502 used CNTs for the separation of protein, where CNTs were the stationary phase in capillary electrophoresis. Exact and efficient separation of biomolecules is a tedious task in electrophoresis. The efficiency of electrophoretic separation of various proteins, peptides, and DNA can be improved by combining CNTs with specific and selected polymers employed as matrices in an appropriate ratio.
Polyacrylamide gel electrophoresis (PAGE) is the most popular technique for protein separation. PAGE involves separation in the presence of an external electric field through a porous gel matrix. Here, the separation is controlled by differences in charge, density, size, and shape of the protein molecules. Sodium dodecyl sulfate (SDS)-PAGE is another method through which a denatured protein can be separated based on molecular weight using a surfactant (i.e., SDS).505,506 Pore size is a crucial factor determining the efficiency of separation in all electrophoresis methods. The gel formed by polyacrylamide (PAM) is not uniform in terms of size and shape.505,507 Hence, the separation efficiency of molecules can be improved by effectively combining high-aspect ratio nanomaterials (e.g., CNTs) with specific and selected polymers for the formation of matrices to provide unique properties, e.g., self-organization, adsorption, good percolation behavior, and high conductivity.506,508,509 Using this approach, Huang et al.510 improved the separation efficiency of apolipoprotein A-1 and complement C3 by using CNTs with PAM as a matrix in polyacrylamide gel electrophoresis. A schematic diagram describing the separation of biomolecules through the matrix is presented in Fig. 14.
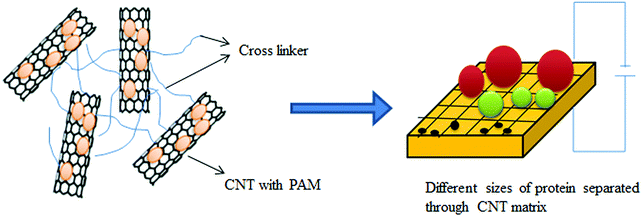 |
| Fig. 14 Schematic layout for the separation of protein through a CNT based matrix. | |
Over the past several years, various biological applications of CNTs have been explored, and many have focused on the interactions between CNTs and proteins.511–514 Such efforts led to the progress in the separation and identification of proteins in disease diagnosis using PAGE when CNTs were employed in the gel. Guo et al.515 reported the separation and detection of human serum proteins by adding SWNTs and MWNTs to the native PAGE gel. They used SWNTs functionalized with Triton X-100 and carboxylate functionalized MWNTs to properly ensure dispersion in the gel for more specific separation of serum proteins (IgG) than that achieved by normal PAGE.515 Similarly, Jiang et al.516 reported the separation of complement C3 protein and haptoglobin (Hp) from human serum to distinguish the samples between a healthy person and a patient with liver disease. For this, they used CNTs modified with Triton X-100 and carboxylate to produce water-soluble proteins; then, serum samples were run through the matrix for separation and identification of proteins.516
CNTs were incorporated into an SDS-PAGE gel matrix to determine the molecular weight of proteins and to assess the interaction between serum proteins and nanotubes.517,518 Variations in the molecular weight calibration plots were observed in terms of the standard deviation by 55% for MWNTs and by 34% for SWNTs used in gel matrix formation, when compared to pristine polyacrylamide gels.517 The interactions between the serum proteins and CNTs were highly dependent on the physicochemical properties of the CNTs, including the type, size, arrangement model, and surface modification.518 These authors also clarified that various serum proteins (such as albumin, prealbumin, transferrin, and immunoglobulin) were quickly adsorbed on the CNT surface, whereas the adsorption of proteins proceeded rather slowly on CNTs functionalized with PEG.518 Apart from the pore size distribution and morphology, the formation of the gel matrix was also affected by many other factors such as polymerization kinetics, purity of monomers, cross-linkers and reagents used for polymerization, temperature, and oxygen content in the atmosphere.505 Gunavadhi et al.519 used MWNTs in a polyacrylamide gel in two different ways. First, MWNTs were used only as fillers inside the gel matrix. Second, MWNTs were used as a catalyst for the polymerization of acrylamide.519
5. Challenges and optimization strategies for using CNTs in diverse applications
Carbon nanotubes have been investigated intensively over the past two decades. One of the critical factors that hamper their widespread use in diverse fields of the applications lies in their controlled synthesis. To date, numerous reports have been made which focus on the synthesis of CNTs based on physical/chemical methods. Nonetheless, there are yet no reports regarding the biogenic synthesis of CNTs using an ecofriendly approach, although various forms of nanoparticles have already been synthesized by using biogenic sources (plants, bacteria, fungi, yeast, etc.). If CNTs can ever be synthesized by biogenic processes, then eco-friendly products may be possible due to the potential for reduced toxicity. In addition, the use of such products will be more feasible for clinical applications.
Another important aspect to consider is the durability of the CNTs when transferred inside the body when administered with a conjugate. If CNTs are conjugated with the inorganic non-biodegradable metals such as gold or silver, carbon nanomaterials would be retained safely in various parts of the body. As such, there are many reports on the low toxicity of CNTs in vitro and in vivo, when they are administered with an appropriate surface coating and size. Therefore, the development of a biocompatible and biodegradable nanoagents is expected to further expand the list of clinical and/or biomedical applications of CNTs.
The use of CNTs in phototherapy is based on the NIR light. As the effective penetration depth of NIR light is still shallow, its application is limited to only some types of cancers such as skin cancer, oral cancer, esophageal cancer, and stomach cancer. Scaffolds may cause toxicological effects when using CNTs in tissue engineering. Hence, actual toxicological assessments of CNT fibers and polymers needs to be conducted in vivo on a long-term basis. Thus, more studies need to be carried out to propose the realistic scenarios for the exposure to nanocomposites containing CNTs along with their life-cycle. Exposure to CNTs during manufacturing, during the product life-cycle, and after use is also a significant concern for manufacturers, consumers, and the environment. Advances in CNT surface modifications techniques are expected to expand their use in health care applications. Indeed, many reports are available regarding healthcare applications of CNTs. However, many challenges still remain toward clinical application of modified (functionalized) CNTs to take the full advantages of their usefulness and effectiveness. The important aspects regarding the functionalization, dispersion, and toxicity of CNTs are discussed below.
5.1 Functionalization
Different strategies are required for the surface functionalization of each type of nanomaterial to make them soluble or dispersed in aqueous media. Carbon nanotubes can be functionalized using two approaches – a covalent or a non-covalent scheme depending on the specific requirement for a particular application (Fig. 15). In the former scheme, various chemical approaches have been developed toward the formation of chemical bonds with carbon nanotubes such as either at the end or on the sidewalls.520 The chemical reaction can involve oxidation,521–523 halogenation (fluorination,524–526 chlorination,527–529 and bromination530–532), amidation,533,534 thiolation,535,536 hydrogenation,537,538 addition of radicals,539,540 addition of carbenes,541,542 or addition of nitrene.543,544 The use of covalent functionalization is limited because it generates sp3 hybridization on the carbon sites of CNTs, which blocks the transitions of π-electrons. Consequently, novel CNT properties may be lost. Further, covalent functionalization of CNTs provides a broad selection of chemical approaches for conjugation as well as solubilization. Covalent conjugation of CNTs with other molecules can also cause damage to the π-network, which is a big concern, especially for fluorescence imaging applications possible in the NIR-II window (1000–1700 nm) as it leads to a decrease in fluorescence quantum efficiency. Still, several researchers used this approach for the fluorescence imaging applications in a second near-infrared window.545–548
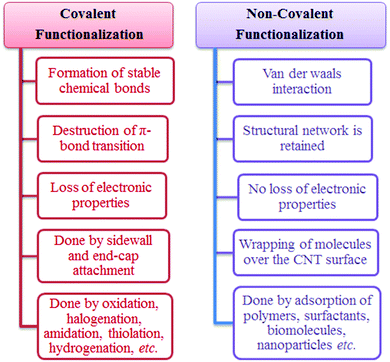 |
| Fig. 15 Comparison of covalent and non-covalent functionalization of CNTs. | |
Non-covalent functionalization of CNTs has the potential to be used for supra-molecular adsorption or wrapping of various functional groups like amphiphilic surfactants,549–551 polymers,552–554 or biomolecules.127,129,130,555 Non-covalent functionalization can be used to enhance the solubility and dispersibility of CNTs. However, it has limited capability for drug delivery because it is based on weak van der Waal's forces. Hence, such factors can modify the tube surface, the π–π network of carbon atoms in CNTs, and the hydrophobic nature of CNTs. The generation of sp3 hybridization on carbon sites of CNTs is favorable in pharmaceutical drug delivery because blocking the transitions of π-electrons allows for the slow release of the therapeutic agent. Therefore, covalently functionalized CNTs are more prominent and useful for diagnosis and therapeutic delivery.131,138,239
5.2 Dispersion
Dispersion is one of the most important factors to consider in the fabrication of CNT/polymer nanocomposites. The agglomeration behavior of CNTs is known to affect the mechanical, rheological, thermal, and electrical properties required for their diverse applications. The hydrophobic nature of CNT sidewalls and the strong interactions between individual tubes can lead to the congregation of CNTs in a bundle-like shapes. To achieve an optimal dispersion of CNTs, the medium should be compatible enough to wet the hydrophobic surfaces without inducing CNT aggregation. Dispersion of CNTs can be achieved either by chemical or physical approaches. As explained below, the chemical approaches include (1) surfactant-assisted dispersion,556–560 (2) solvent dispersion,561–563 (3) polymer-assisted dispersion,564–566 and (4) biomolecular dispersion.567–571
(1) Surfactant-assisted dispersion.
This is one of the main approaches to disperse and exfoliate carbon nanotubes (and graphene) by using surfactants. A variety of surfactants including anionic, cationic, and nonionic surfactants have been used in different studies. However, the exact mechanism of interaction between CNTs and surfactants is still uncertain. It was suggested that van der Waals interactions, π–π stacking, and hydrophobic interactions should be responsible for the dispersion of CNTs.572 Sodium dodecyl sulfate (SDS) was used to disperse CNTs in a nematic solvent.573,574 The proposed mechanism behind the stability of dispersion was the small density gradients existing between the nanotubes and the nematic fluid. A layer formed on the CNT (i.e., adsorbed onto the nanotube surface) by the surfactant or nematic micelles helped decrease their tendency to aggregate in water. Monodisperse assemblies of CNTs can also be achieved by using the surfactant dodecyl itaconate.575 There are numerous types of surfactants that have been used by researchers for creating stable dispersions such as sodium deoxycholate, Triton X-405, Brij S-100, Pluronic F-127, and polyvinyl pyrrolidone.574–579
(2) Solvent dispersion.
The solvents used most often for making CNT dispersions are polar–polar solvents such as water, ethanol, dimethylformamide, tetrahydrofuran, dimethylacetamide, 1,2-dichlorobenzene, and dimethyl pyrrolidone. Pristine CNTs were shown to form larger aggregates than oxidized CNTs.580–582 Previous studies have shown that the oxidation of CNT side walls improves their stability in polar solvents. The application of a solvent to the oxidized nanocarbons induced much stronger repulsion between them (i.e. nanocarbons or nanotubes) as compared to pristine nanocarbons which helped maintain a stable dispersion.583 Many solvents have been tested to disperse oxidized CNTs and more efficient routes of their dispersion using those solvents have been characterized for different methods.584–588
(3) Polymer-assisted dispersion.
Polymer-assisted dispersion has also been used to enhance the dispersion of CNTs in both aqueous589,590 and organic solvents591 because it facilitates separation of nanotubes from carbonaceous and metal impurities.592 Several types of polymers including poly(phenylene vinylene), polyvinyl pyrrolidone, polystyrene sulfonate, and polylactic acid have been used for the efficient dispersion of CNTs. The CNT–polymer composites are formed by the wrapping of polymers around the CNTs to form supramolecular complexes of CNTs.579,593,594
Several methods including solution mixing, melt mixing, electrospinning, in situ polymerization, liquid crystalline phase-induced methods, and chemical functionalization have been adopted to achieve a stable dispersion of nanotubes in the polymer matrix.552,595 For the synthesis of CNT/polymer composites, a thin film composite amorphous-CNT was initially treated with polyvinyl alcohol to improve CNT dispersion in water.596 This composite was seen to have higher crystallinity with the increase in amorphous-CNT concentration relative to pure polyvinyl alcohol. Functionalized CNTs (PEGylated-CNTs), made of these composites, exhibited enhanced colloidal stability in water (or a better dispersibility).586,597 As with the synthetic polymers, natural polymers such as gum arabic,598–600 gellan gum,601 cellulose,602 and lignin603 can also be used to enhance the dispersion of CNTs.
(4) Biomolecular dispersion.
Numerous biomolecules (e.g., peptides, proteins, DNA, and RNA) are also recognized as useful to maintain the stable dispersion of CNTs through the interaction on their surface.604–606 Functionalization of CNTs with lysine was reported for the first time using a microwave irradiation method.466 A diazonium reaction between lysine molecules and CNTs induced a covalent attachment of lysine to the nanotube surfaces, and the aqueous solubility of this complex then increased considerably.466 The covalent linkage of ssDNA containing two stretches of cytosine-rich domains to carbon nanotubes was also proposed for the enhanced dispersion of CNTs in a water–glycerol binary solvent.605 The use of double stranded calf-thymus DNA (dsDNA) was in fact demonstrated to successfully disperse the bamboo-like MWCNTs.607 The interaction between DNA and CNTs caused the formation of a supramolecular complex with superior biostability based on π-stacking between anionic groups of DNA (aromatic bases) and cationic groups on the CNT surface. Recently, a supramolecular complex was synthesized by polymerizing the polypeptide group to the CNT surface.608 The authors suggested that the dispersion of the complex was facilitated in both polar and non-polar media by the interaction between the polypeptide and the CNT surface.
Physical approaches for dispersion include some mechanical processes such as ultrasonication, calendaring, ball milling, and stir/extrusion.552 Ultrasonication is an effective tool for the dispersion of CNTs in liquids; it can be achieved with the help of a sonicator by applying ultrasonic energy to aggregated particles in solution form. This ultrasonic energy produces shock waves that can enhance the “peeling off” effect of aggregated particles to separate individual particles. If sonication is continued for a longer duration, the layered carbon nanotubes may be mechanically damaged.609 Calendering can also be applied by using three roll mills in which a shear force is generated for mixing, dispersion, or homogenization of viscous materials.610 Ball milling is a grinding method used to reduce the size of the material to a fine powder based on the cascading effect. Ball milling of CNTs has been applied to transform CNTs into nanoparticles,611 to introduce a chemical moiety,612 to modify the morphology of CNTs,613 and to generate carbon nanoparticles for hydrogen storage applications.614 Stir/extrusion is also a popular technique for the dispersion of CNTs in either a liquid system or in a polymer matrix. To achieve fine dispersion, a high shear stress should be applied to the agglomerated CNTs.615 Most of the aforementioned physical techniques are also useful to attain a better dispersion of CNTs; however, if dispersion is maintained on a long-term basis by the above methods, it may cause damage to the surface and/or structure as well as properties of carbon nanotubes.616–619 Thus, to avoid the problems associated with the mechanical approaches, a chemical approach (explained above) has been preferred for the dispersion of CNTs.
A number of quantitative methods have been proposed to assess the dispersion of CNTs. The most common techniques for such evaluations are UV-visible spectroscopy,620–624 Fourier-transform infrared spectroscopy,625,626 nuclear magnetic resonance spectroscopy,627,628 Raman scattering,629–631 small-angle neutron scattering,632,633 and small-angle X-ray scattering.634,635 Some imaging techniques such as scanning electron microscopy636,637 and transmission electron microscopy638,639 have also been utilized for such purposes.
5.3 Toxicity
Human safety is a basic requirement for any kind of material used in the field of medical research. Several researchers documented the health impact of particle size in the nanometer range (approximately 1–100 nm). It did not take long for concerns to be raised over possible health issues associated with these nanoscale materials. The pharmacological parameters such as adsorption, distribution, metabolism, excretion, and toxicity should be evaluated for nanoscale materials utilized in medicine. As the toxicity profile of CNTs is a main concern, it has been studied extensively in vitro and in vivo. Numerous reports are available on this issue, wherein various kinds of cell lines and indicator dyes have been utilized for the determination of cytotoxicity. Many studies showed that the size and surface area of the CNTs are important variables affecting toxicological parameters.640–643
A small size structure with increased surface area can lead to enhanced interactions with living cells and translocation into the cells. As such, interactions might result in adverse biological effects that would not be seen with larger CNTs.640,642 Various researchers have also reported that SWNTs should exhibit a greater cytotoxic effect, whereas MWNTs had less cytotoxic effects.460 In addition to these factors, there are a number of other important factors that can determine the cytotoxicity of CNTs: (i) the size of the CNTs used, (ii) the surface area presented to the target organ, (iii) the type of CNTs, (iv) the type of functionalization, (v) the purified product, (vi) dispersion, (vii) the type and concentration of the surfactant, (viii) the different cell culture media, (ix) the cell type, and (x) the duration of the interaction.
Some reports also suggest that the route of administration of CNTs might be responsible for the degree of toxicity. It was found that a dose delivered through the subcutaneous route644 is safer than via the abdominal cavity645 or into the bloodstream.646 Although many researchers are studying the mechanism of CNT toxicity, its significance has not yet been fully elucidated. The identified mechanisms of CNT toxicity are diverse enough to include oxidative stress,647,648 rupture of the cell membrane,460 disruption of intracellular metabolic pathways,460 generation of reactive oxygen species,649,650 and induction of transcription factors.651,652 MWNTs have been reported to have remarkable radical scavenging capacity, which can effectively reduce oxidative stress effectively relative to SWNTs.653,654Table 5 summarizes a list of studies that have focused on the toxicity of CNTs.
Table 5 Various reports on the toxicity profile of CNTs
S. no. |
Affected body part |
Consequences |
Ref. |
(A) In vitro study of SWNTs |
1 |
Lung cells and pulmonary system |
Acute toxic effects and apoptosis in human lung epithelial cells |
655
|
2 |
Skin |
Increased epidermal thickness and activation of dermal fibroblasts, which resulted in increased collagen and release of pro-inflammatory cytokines and inflammation |
656
|
3 |
Human blood cells |
A decrease in the number of human peripheral lymphocyte cells |
657
|
4 |
Human brain cells |
SWNT–surfactant conjugate showed cytotoxicity on human astrocytoma 1321N1 cells |
658
|
5 |
Human fibroblast cells |
Interactions between SWNTs and fibroblast cells caused cellular apoptosis or necrosis |
659
|
6 |
Human embryonic kidney cells |
SWNTs induced apoptosis and decreased the cellular adhesion ability of human embryonic kidney (HEK293) cells |
660
|
7 |
Neural cells |
SWNTs and graphene induced apoptosis in neural phaeochromocytoma-derived PC12 cells |
661
|
8 |
Neural and glial cells |
Acute toxic effects in primary cultures of the central and peripheral nervous system of chicken embryos |
662
|
9 |
Endothelial cells |
Caused endothelial dysfunction and alteration in the expression of genes and proteins involved in fibrinolysis; displayed pro-thrombotic effects |
663
|
|
(B) In vivo study of SWNTs |
10 |
Pulmonary system |
SWNTs can induce chronic pulmonary inflammation and granuloma formation and also produce oxidative stress |
664
|
11 |
Skin |
Formation of free-radical species; accumulation of mast cells resulting in skin thickening; caused oxidative stress, inflammation, and dermal toxicity |
665
|
12 |
The aquatic environment |
SWNTs not digested by Caenorhabditis elegans moved up the food chain to be consumed by benthivores. Thus, there is a chance that SWNTs could alter the food chain |
666
|
13 |
Platelets |
Significantly increased platelet P-selectin expression, exerted prothrombotic effects |
667
|
|
(C) In vitro study of MWNTs |
14 |
Pulmonary cells |
Induction of the overproduction of TNF-alpha by macrophages |
651
|
15 |
Human lung and immune cells |
Acute effects on human lungs and immune cells Accumulation and biopersistence leads to long-term consequences |
668
|
16 |
Lung cells |
Loss of membrane integrity, change in surface morphology, induced genotoxicity |
669
|
17 |
Human embryonic kidney cells |
Concentration-dependent cytotoxicity in cultured HEK293 cells, caused oxidative stress |
670
|
18 |
Keratinocytes |
MWNTs changed the keratinocyte cell morphology |
671
|
19 |
Keratinocytes |
Exposure to MWNTs led to altered protein expression in human keratinocytes |
672
|
20 |
Lymphocytes |
MWNTs induced apoptosis in T-lymphocytes |
673
|
21 |
Dermal cells |
Induced a massive loss of cell viability through DNA damage and programmed cell-death |
674
|
|
(D) In vivo study of MWNTs |
22 |
The aquatic environment |
Acute and long-term effects of functionalized MWNTs in zebrafish |
675
|
23 |
Pulmonary cells |
Induced inflammatory and fibrotic reactions and pulmonary lesions |
651
|
24 |
Immune cells |
Produced oxidative stress, unpurified MWNTs produced twice as much oxidation than purified CNTs; caused mitochondrial dysfunction |
676
|
6. Conclusion and future perspectives
In the present review, we summarized the various healthcare applications of CNTs. CNTs are providing numerous opportunities in diverse fields of applications including sensors, therapeutics, composite materials, tissue engineering, microbial treatment, efficient emitters, to optical tags, and nanocarriers. A number of parameters including size, shape, structure, and helicity are vital to providing these novel tubular structures with amazing chemical, optical, electrical, and mechanical properties. Numerous strategies for CNT functionalization based on covalent and non-covalent schemes by which chemical entities can also be attached have been developed and adopted for the modification of these tubular structures. The delivery of different therapeutic agents (drugs, peptides, proteins, genes, and immune modulators) through the biological membrane has also been discussed. The high loading capacity and ability to release therapeutic agents at targeted sites in a controlled manner should result in CNTs being used as preferred nanocarriers. The extraordinary optical properties of CNTs, especially in the NIR region, also helped extend their applicability toward photoacoustic imaging and photothermal ablation therapy for the selective imaging and treatment of cancer, respectively. CNTs still have immense potential in the areas of chemical force microscopy and allied fields due to their numerous merits including the high aspect ratio, elastic properties, modification flexibility, conductive nature, and ability to maintain structural integrity. The ability to transfer electrons at a rapid pace at the interface also allows them to act as electrocatalysts in the development of ultrasensitive, selective, and robust chemical sensors/biosensors.
Nanoengineering of CNT-based electrodes will result in fast, efficient, reliable, and cost-effective point-of-care devices for food, packaging, quality assurance, and diagnostics in the near future. The utilization of CNTs in antimicrobial treatment, bone scaffolds, and protein separation is also highlighted herein. CNT-nanosensors may be used to give online monitoring of blood pressure through in situ measurements of pressure inside the heart valves. CNT-based nano-wires may be utilized for the angiography and angioplasty applications. CNTs may also be utilized for hair grafting applications to support the hair roots and to generate electrical impulses as the sinoatrial (SA) nodes or artificial pacemakers. Likewise, CNTs can also be applied as ligatures and sutures through proper sterilization, as support for the fractured bone (as biocompatible supporting material), and as artificial enamel for the root canal treatment (RCT) of teeth.
Among the many issues involved in the use of CNTs, one of the most important factors is the long-term toxicity effects. Toxicity behaviors of CNTs, i.e., in terms of in vivo studies including absorption, biodistribution, accumulation, and enduring effects, have been documented to reflect their toxicity in various organs of the body. As well-functionalized CNTs exert minimum toxicity in vitro and in vivo, special attention must be paid to the optimal functionalization of CNTs with the minimization of the toxic side effects. Overall, CNTs represent a superior material with excellent properties capable of providing innovative future solutions to current problems in the healthcare industry.
Acknowledgements
Sandeep Kumar thanks DST, Govt. of India, for a research grant (letter no. SERB/ET-0038/2013 dated 16-08-2013). Ruma Rani (IF 120268) thanks DST for providing financial assistance in the form of an INSPIRE fellowship. Ki-Hyun Kim acknowledges the support made in part by grants from the National Research Foundation of Korea (NRF) funded by the Ministry of Education, Science and Technology (MEST) (No. 2006-0093848) and from the Cooperative Research Program for Agriculture Science & Technology Development (Project title: Study on model development to control odor from a hog barn, Project No. PJ010521), Rural Development Administration, Republic of Korea.
References
- S. T. Reddy, A. Rehor, H. G. Schmoekel, J. A. Hubbell and M. A. Swartz, J. Controlled Release, 2006, 112(1), 26–34 CrossRef CAS PubMed.
- S. Iijima, Nature, 1991, 354, 56–58 CrossRef CAS.
-
P. J. F. Harris, Carbon Nanotube Science – Synthesis, Properties, and Applications, Cambridge Univ. Press, Cambridge, 2009 Search PubMed.
- J. Bernholc, D. Brenner, M. B. Nardelli, V. Meunier and C. Roland, Annu. Rev. Mater. Res., 2002, 32, 347–375 CrossRef CAS.
- Y. Liang, Y. Li, H. Wang and H. Dai, J. Am. Chem. Soc., 2013, 135, 2013–2036 CrossRef CAS PubMed.
- H. L. Wang and H. J. Dai, Chem. Soc. Rev., 2013, 42, 3088–3113 RSC.
- Z. Liu, S. Tabakman, K. Welsher and H. Dai, Nano Res., 2009, 2, 85–120 CrossRef CAS PubMed.
- Z. Liu, J. T. Robinson, S. M. Tabakman, K. Yang and H. Dai, Mater. Today, 2011, 14, 316–323 CrossRef CAS.
- G. Hong, S. Diao, J. Chang, A. L. Antaris, C. Chen, B. Zhang, S. Zhao, D. N. Atochin, P. L. Huang, K. I. Andreasson, C. J. Kuo and H. Dai, Nat. Photonics, 2014, 8, 723–730 CrossRef CAS PubMed.
- A. L. Antaris, J. T. Robinson, O. K. Yaghi, G. S. Hong, S. Diao, R. Luong and H. Dai, ACS Nano, 2013, 7, 3644–3652 CrossRef CAS PubMed.
-
A. Jacoby, Global Markets and Technologies for Carbon Nanotubes. A BCC Research Nanotechnology Report, 2015, Report Code: NAN024F. http://www.bccresearch.com/market-research/nanotechnology/carbon-nantubes-global-markets-technologies-report-nan024f.html.
- J. Prasek, J. Drbohlavova, J. Chomoucka, J. Hubalek, O. Jasek, V. Adamc and R. Kizek, J. Mater. Chem., 2011, 21, 15872–15884 RSC.
- J. L. Hutchison, N. A. Kiselev, E. P. Krinichnaya, A. V. Krestinin, R. O. Loutfy, A. P. Morawsky, V. E. Muradyan, E. D. Obraztsova, J. Sloan, S. V. Terekhov and D. N. Zakharov, Carbon, 2001, 39(5), 761–770 CrossRef CAS.
- Y. L. Zhang, P. X. Hou, C. Liu and H. M. Cheng, Carbon, 2014, 74, 370–373 CrossRef CAS.
- N. Sano, J. Nakano and T. Kanki, Carbon, 2004, 42(3), 686–688 CrossRef CAS.
- K. Awasthi, A. Srivastava and O. N. Srivastava, J. Nanosci. Nanotechnol., 2005, 5, 1616–1636 CrossRef CAS PubMed.
- Y. Wang, Z. Zhang, H. Liu, X. Xu, G. Pan, Z. Guo, Y. Liu, X. Han and G. Lan, Spectrochim. Acta, Part A, 2002, 58, 2089–2095 CrossRef.
- K. Imasaka, Y. Kanatake, Y. Ohshiro, J. Suehiro and M. Hara, Thin Solid Films, 2006, 506, 250–254 CrossRef.
- C. Journet and P. Bernier, Appl. Phys. A: Mater. Sci. Process., 1998, 67, 1–9 CrossRef CAS.
- T. Sagara, S. Kurumi and K. Suzuki, Appl. Surf. Sci., 2014, 292, 39–43 CrossRef CAS.
- H. Lange, M. Sioda, A. Huczko, Y. Q. Zhu, H. W. Kroto and D. R. M. Walton, Carbon, 2003, 41(8), 1617–1623 CrossRef CAS.
- S.-D. Wang, M.-H. Chang, K. M.-D. Lan, C.-C. Wu, J.-J. Cheng and H.-K. Chang, Carbon, 2005, 43(8), 1792–1795 CrossRef CAS.
- L. Li, F. Li, C. Liu and H.-M. Cheng, Carbon, 2005, 43(3), 623–629 CrossRef CAS.
- M. Jahanshahi, J. Raoof, S. Hajizadeh and R. J. Seresht, Phys. Status Solidi A, 2009, 206(1), 101–105 CrossRef CAS.
- B. Chen, X. Zhao, S. Inoue and Y. Ando, J. Nanosci. Nanotechnol., 2010, 10, 3973–3977 CrossRef CAS PubMed.
- G. Tripathi, B. Tripathi, M. Sharma, Y. K. Vijay, A. Chandrad and I. P. Jain, Int. J. Hydrogen Energy, 2012, 37(4), 3833–3838 CrossRef CAS.
- Y. A. Kim, H. Muramatsu, T. Hayashi and M. Endo, Carbon, 2012, 50(12), 4588–4595 CrossRef CAS.
- J. Zhao, L. Wei, Z. Yang and Y. Zhang, Phys. E, 2012, 44(7), 1639–1643 CrossRef CAS.
- Y. Wu, T. Zhang, F. Zhang, Y. Wang, Y. Ma, Y. Huang, Y. Liu and Y. Chen, Nano Energy, 2012, 1(6), 820–827 CrossRef CAS.
- Y. Su, Y. Zhang, H. Wei, B. Qian, Z. Yang and Y. Zhang, Phys. E, 2012, 44(7), 1548–1551 CrossRef CAS.
- Y. Zhang, Mater. Charact., 2012, 68, 102–109 CrossRef CAS.
- M. I. Mohammad, A. A. Moosa, J. H. Potgieter and M. K. Ismael, ISRN Nanomater., 2013, 2013, 1 Search PubMed.
- L. Fang, L. Sheng, K. An, L. Yu, W. Ren, Y. Ando and X. Zhao, Phys. E, 2013, 50, 116–121 CrossRef CAS.
- Y. Su, P. Zhou, J. Zhao, Z. Yang and Y. Zhang, Mater. Res. Bull., 2013, 48(9), 3232–3235 CrossRef CAS.
- S. Yousef, A. Khattab, T. Osman and M. Zaki, J. Nanomater., 2013, 2013, 1–9 CrossRef.
- Y. Su, H. Wei, T. Li, H. Geng and Y. Zhang, Mater. Res. Bull., 2014, 50, 23–25 CrossRef CAS.
- T. Sagara, S. Kurumi and K. Suzuki, Appl. Surf. Sci., 2014, 292, 39–43 CrossRef CAS.
- Y. Su and Y. Zhang, Carbon, 2015, 83, 90–99 CrossRef CAS.
- X. Cai, H. Cong and C. Liu, Carbon, 2012, 50(8), 2726–2730 CrossRef CAS.
- K. H. Maria and T. Mieno, Vacuum, 2015, 113, 11–18 CrossRef CAS.
- B. A. Joseph, M. Jagannatham, R. D. Rohit and H. Prathap, Diamond Relat. Mater., 2015, 55, 12–15 CrossRef.
- A. B. Belgacem, I. Hinkov, S. B. Yahia, O. Brinza and S. Farha, Mater. Today Commun., 2016, 8, 183–195 CrossRef.
- J. B. Nagy, G. Bister, A. Fonseca, D. Mehn, Z. Konya, I. Kiricsi, Z. E. Horvath and L. P. Biro, J. Nanosci. Nanotechnol., 2004, 4, 326–345 CrossRef CAS PubMed.
- A. Thess, R. Lee, P. Nikolaev, H. Dai, P. Petit, J. Robert, C. H. Xu, Y. H. Lee, S. G. Kim, A. G. Rinzler, D. T. Colbert, G. E. Scuseria, D. Tomanek, J. E. Fischer and R. E. Smalley, Science, 1996, 273, 483–487 CAS.
-
H. Dai, in Top. Appl. Phys., ed. M. Dresselhaus, G. Dresselhaus and P. Avouris, Springer Verlag, 2000, vol. 80 Search PubMed.
- T. Guo, P. Nikolaev, A. Thess, D. T. Colbert and R. E. Smalley, Chem. Phys. Lett., 1995, 243, 49–54 CrossRef CAS.
- C. D. Scott, S. Arepalli, P. Nikolaev and R. E. Smalley, Appl. Phys. A: Mater. Sci. Process., 2001, 72(5), 573–580 CrossRef CAS.
- S. Arepalli, J. Nanosci. Nanotechnol., 2004, 4(4), 317–325 CrossRef CAS PubMed.
- N. E. Braidy, M. A. Khakani and G. A. Botton, J. Mater. Res., 2002, 17(9), 2189–2192 CrossRef CAS.
- M. Kusaba and Y. Tsunawaki, Thin Solid Films, 2006, 506, 255–258 CrossRef.
- L. L. Lebel, B. Aissa, M. A. E. Khakani and D. Therriault, Compos. Sci. Technol., 2010, 70, 518–524 CrossRef CAS.
- M. Karimi, N. Solati, M. Amiri, H. Mirshekari, E. Mohamed, M. Taheri, M. Hashemkhani, A. Saeidi, M. A. Estiar, P. Kiani, A. Ghasemi, S. M. M. Basri, A. R. Aref and M. R. Hamblin, Expert Opin. Drug Delivery, 2015, 12(7), 1071–1087 CrossRef CAS PubMed.
- M. Jose-Yacaman, Appl. Phys. Lett., 1993, 62, 657–659 CrossRef CAS.
- H. M. Cheng, F. Li, X. Sun, S. D. M. Brown, M. A. Pimenta, A. Marucci, G. Dresselhaus and M. S. Dresselhaus, Chem. Phys. Lett., 1998, 289, 602–610 CrossRef CAS.
- B. C. Satishkumar, A. Govindaraj, R. Sen and C. N. R. Rao, Chem. Phys. Lett., 1998, 293, 47–52 CrossRef CAS.
- A. Magrez, J. W. Seo, R. Smajda, M. Mionić and L. Forró, Materials, 2010, 3, 4871–4891 CrossRef CAS.
- M. Kumar and Y. Ando, J. Nanosci. Nanotechnol., 2010, 10, 3739–3758 CrossRef CAS PubMed.
- J. H. Hafner, M. J. Bronikowski, B. R. Azamian, P. Nikolaev, A. G. Rinzler, D. T. Colbert, K. A. Smith and R. E. Smalley, Chem. Phys. Lett., 1998, 296, 195–202 CrossRef CAS.
- E. Flahaut, A. Govindaraj, A. Peigney, C. Laurent, A. Rousset and C. N. R. Rao, Chem. Phys. Lett., 1999, 300, 236–242 CrossRef CAS.
- S. Maruyama, Y. Miyauchi, T. Edamura, Y. Igarashi, S. Chiashi and Y. Murakami, Chem. Phys. Lett., 2003, 375, 553–559 CrossRef CAS.
- S. Kumar, R. Kumar, V. K. Jindal and L. M. Bharadwaj, Mater. Lett., 2008, 62(4–5), 731–734 CrossRef CAS.
- K. A. Shah and B. A. Tali, Mater. Sci. Semicond. Process., 2016, 41, 67–82 CrossRef CAS.
- J. Liu, Z. W. Jiang, H. O. Yu and T. Tang, Polym. Degrad. Stab., 2011, 96, 1711–1719 CrossRef CAS.
- R. V. Parthasarathy, K. L. N. Phani and C. L. R. Martin, Adv. Mater., 1995, 7, 896–897 CrossRef CAS.
- T. Kyotani, L. F. Tsai and A. Tomita, Chem. Mater., 1996, 8, 2109–2113 CrossRef CAS.
- M. S. Dresselhaus, G. Dresselhaus, A. Jorio, A. G. S. Filho, M. A. Pimenta and R. Saito, Acc. Chem. Res., 2002, 35, 1070–1078 CrossRef CAS PubMed.
- E. V. Lobiak, E. V. Shlyakhov, L. G. Bulushev, P. E. Plyusnin, Y. V. Shubin and A. V. Okotrub, J. Alloys Compd., 2015, 621, 351–356 CrossRef CAS.
- Q. Zhang, J. Q. Huang, M. Q. Zhao, W. Z. Qian and F. Wei, ChemSusChem, 2011, 4, 864–889 CrossRef CAS PubMed.
- N. Sano, S. Ishimaru and H. Tamaon, Mater. Chem. Phys., 2010, 122, 474–479 CrossRef CAS.
- Y. Yan, J. Miao, Z. Yang, F.-X. Xiao, H. B. Yang, B. Liu and Y. Yang, Chem. Soc. Rev., 2015, 44, 3295–3346 RSC.
-
H. G. Tennett, WO8603455A1, Hyperion Catalysis Int., 1986.
-
(a) M. Endo, Chem. Tech., 1988, 18, 568–576 CAS;
(b) M. Endo, K. Takeuchi, S. Igarashi, K. Kobori, M. Shiraishi and H. W. Kroto, J. Phys. Chem. Solids, 1993, 54, 1841–1848 CrossRef CAS.
-
(a)
D. E. Resasco, B. Kitiyanan, J. H. Harwell, W. Alvarez, D. Jang, W. E. Alvarez, J. E. Herrera, L. Balzano, D. Resasco and J. Harwell, WO200073205A, Univ. Oklahoma, 2007;
(b)
D. E. Resasco, B. Kitiyanan, W. Alvarez, L. Balzano, B. Kitivanan and W. E. Alvarez, WO200194260A, Univ. Oklahoma, 2001;
(c)
D. E. Resasco, B. Kitiyanan, W. E. Alvarez and L. Balzano, US2006039849A1, Univ. Oklahoma, 2006.
- W. E. Alvarez, B. Kitiyanan, A. Borgn and D. E. Resasco, Carbon, 2001, 39, 547–558 CrossRef CAS.
-
R. E. Smalley, K. A. Smith, D. T. Colbert, P. Nikolaev, M. J. Bronikowski, R. K. Bradley, F. Rohmund, R. Smalley, K. Smith and D. Colbert, WO200026138A, Univ. Rice, 2000.
- P. Nikolaev, M. J. Bronikowski, R. K. Bradley, F. Rohmund, D. T. Colbert, K. A. Smith and R. E. Smalley, Chem. Phys. Lett., 1999, 313, 91–97 CrossRef CAS.
-
F. Wei, G. Luo, Y. Wang, H. Yu, Z. Li, W. Qian, Z. Wang and Y. Jin, WO200294713A, Tsinghua Univ., 2002.
- Y. Wang, F. Wei, G. H. Luo, H. Yu and G. S. Gu, Chem. Phys. Lett., 2002, 364, 568–572 CrossRef CAS.
-
(a) M. Bierdel, S. Buchholz, V. Michele, L. Mleczko, R. Rudolf, M. Voetz and A. Wolf, Phys. Status Solidi
B, 2007, 244, 3939–3943 CAS;
(b)
S. Buchholz, D. G. Duff, V. Michele, L. Mleczko, C. Muennich, R. Rudolf, A. Wolf, D. Gordon, D. Duff, M. Volker, M. Leslaw, M. Christian, R. Reiner and C. Munnich, WO2006050903A2, Bayer Material Science AG, 2006;
(c)
S. Buchholz,V. Michele, L. Mleczko, C. Muennich, R. Rudolf, A. Wolf, M. Leslaw and C. Munnich, WO2007093337A2, Bayer Material Science AG, 2007;
(d)
R. Bellinghausen, S. Buchholz, V. Michele, L. Mleczko, A. Wolf, J. Buhiholcheu, R. Meulrekcheuko, P. Mihel, D. E. Wolf, D. E. S. Buchholz, D. E. V. Michele, D. E. L. Mleczko and D. E. R. Bellinghausen, WO2009043445A1, Bayer Material Science AG, 2009.
-
K. Hata, S. Yasuda and M. Yumura, WO2008096699A1, NIIT, 2008.
- K. Hata, D. N. Futaba, K. Mizuno, T. Namai, M. Yumura and S. Iijima, Science, 2004, 306, 1362–1364 CrossRef CAS PubMed.
- S. Yasuda, D. N. Futaba, T. Yamada, J. Satou, A. Shibuya, H. Takai, K. Arakawa, M. Yumura and K. Hata, ACS Nano, 2009, 3, 4164–4170 CrossRef CAS PubMed.
-
(a)
K. Jiang, S. Fan, Q. Li, and F. Y. Fan, US2004053780A1, Tsinghua Univ., 2004;
(b) X. B. Zhang, K. L. Jiang, C. Teng, P. Liu, L. Zhang, J. Kong, T. H. Zhang, Q. Q. Li and S. S. Fan, Adv. Mater., 2006, 18, 1505–1510 CrossRef CAS.
- D. S. Zhang, L. Y. Shi, J. H. Fang, K. Dai and X. K. Li, Mater. Chem. Phys., 2006, 97, 415–419 CrossRef CAS.
- D. S. Zhang, L. Y. Shi, J. H. Fang, X. K. Li and K. Dai, Mater. Lett., 2005, 59, 4044–4047 CrossRef CAS.
- Q. Jiang, L. J. Song, H. Yang, Z. W. He and Y. Zhao, Electrochem. Commun., 2008, 10, 424–427 CrossRef CAS.
- W. Z. Li, J. G. Wen, Y. Tu and Z. F. Ren, Appl. Phys. A: Mater. Sci. Process., 2001, 73, 259–264 CrossRef CAS.
- M. Kumar and Y. Ando, Carbon, 2005, 43, 533–540 CrossRef CAS.
- S. Maruyama, Y. Murakami, Y. Shibuta, Y. Miyauchi and S. Chiashi, J. Nanosci. Nanotechnol., 2004, 4, 360–367 CrossRef CAS PubMed.
- J. F. Colomer, C. Stephan, S. Lefrant, G. Van Tendeloo, I. Willems, Z. Konya, A. Fonseca, C. Laurent and J. B. Nagy, Chem. Phys. Lett., 2000, 317, 83–89 CrossRef CAS.
- E. Couteau, K. Hernadi, J. W. Seo, L. Thien-Nga, C. Miko, R. Gaal and L. Forro, Chem. Phys. Lett., 2003, 378, 9–17 CrossRef CAS.
- J. Kong, A. M. Cassell and H. J. Dai, Chem. Phys. Lett., 1998, 292, 567–574 CrossRef CAS.
- N. Q. Zhao, C. N. He, Z. Y. Jiang, J. J. Li and Y. D. Li, Mater. Lett., 2006, 60, 159–163 CrossRef CAS.
- K. Voelskow, M. J. Becker, W. Xia, M. Muhler and T. Turek, Chem. Eng. J., 2014, 244, 68–74 CrossRef CAS.
- C. B. Xu and J. Zhu, Nanotechnology, 2004, 15, 1671–1681 CrossRef CAS.
- A. T. Harris, C. H. See, J. Liu, O. Dunens and K. MacKenzie, J. Nanosci. Nanotechnol., 2008, 8, 2450–2457 CrossRef CAS PubMed.
- F. Danafar, A. Fakhru'l-Razi, M. A. M. Salleh and D. R. A. Biak, Chem. Eng. Res. Des., 2011, 89, 214–223 CrossRef CAS.
- D. Y. Kim, H. Sugime, K. Hasegawa, T. Osawa and S. Noda, Carbon, 2012, 50, 1538–1545 CrossRef CAS.
-
N. Yuca, F. Gumus and N. Karatepe, Carbon Nanotubes, Graphene, and Associated Devices VI, 2013, vol. 8814, p. 881406 Search PubMed.
- Y. C. Li, W. Z. Ruan and Z. Y. Wang, IEEE Trans. Nanotechnol., 2013, 12, 352–360 CrossRef CAS.
- W. Z. Ruan, Z. Y. Wang, J. Li, K. L. Jiang and L. T. Liu, IEEE Sens. J., 2011, 11, 3424–3425 CrossRef CAS.
- J. Fukuda, N. Takayama, Y. Asaoka, H. Suda, A. Sugawara and A. Murayama, IEEE Trans. Plasma Sci., 2011, 39, 3133–3139 CrossRef.
- M. Mao and A. Bogaerts, J. Phys. D: Appl. Phys., 2010, 43, 205201 CrossRef.
- M. Hiramatsu, T. Deguchi, H. Nagao and M. Hori, Diamond Relat. Mater., 2007, 16, 1126–1130 CrossRef CAS.
- C.-C. Chiu, M. Yoshimura and K. Ueda, Diamond Relat. Mater., 2008, 17, 611–614 CrossRef CAS.
- Y. H. Wang, J. Lin, C. H. A. Huan and G. S. Chen, Appl. Phys. Lett., 2001, 79, 680–682 CrossRef CAS.
- J. H. Yen, I. C. Leu, C. C. Lin and M. H. Hon, Appl. Phys. A: Mater. Sci. Process., 2005, 80, 415–421 CrossRef CAS.
- A. Okita, Y. Suda, A. Oda, J. Nakamura, A. Ozeki, K. Bhattacharyya, H. Sugawara and Y. Sakai, Carbon, 2007, 45, 1518–1526 CrossRef CAS.
- K. S. Kim, C. T. Kingston, D. Ruth, M. Barnes and B. Simard, Chem. Eng. J., 2014, 250, 331–341 CrossRef CAS.
- C. Castro, M. Pinault, D. Porterat, C. Reynaud and M. Mayne-L'Hermite, Carbon, 2013, 61, 585–594 CrossRef CAS.
- H. Byeon, S. Y. Kim, K. H. Koh and S. Lee, J. Nanosci. Nanotechnol., 2010, 10, 6116–6119 CrossRef CAS PubMed.
- Y. K. Moon, J. Lee, J. K. Lee, T. K. Kim and S. H. Kim, Langmuir, 2009, 25, 1739–1743 CrossRef CAS PubMed.
- G. S. B. McKee, C. P. Deck and K. S. Vecchio, Carbon, 2009, 47, 2085–2094 CrossRef CAS.
- R. N. Othman, I. A. Kinloch and A. N. Wilkinson, Carbon, 2013, 60, 461–470 CrossRef CAS.
- X. S. Yang, L. X. Yuan, V. K. Peterson, A. I. Minett, Y. B. Yin and A. T. Harris, ACS Appl. Mater. Interfaces, 2012, 4, 1417–1422 CAS.
- K. Yang and Z. Liu, Curr. Drug Metab., 2012, 13(8), 1057–1067 CrossRef CAS PubMed.
- S. T. Yang, J. Luo, Q. Zhou and H. Wang, Theranostics, 2012, 2(3), 271–282 CrossRef CAS PubMed.
- K. Donaldson, R. Aitken, L. Tran, V. Stone, R. Duffin, G. Forrest and A. Alexander, Toxicol. Sci., 2006, 92, 5–22 CrossRef CAS PubMed.
- M. R. Gwinn and V. Vallyathan, Environ. Health Perspect., 2006, 114(12), 1818–1825 CAS.
- S. T. Stem and S. E. McNeil, Toxicol. Sci., 2008, 101, 4–21 Search PubMed.
- C. Blaise, F. Gagne, J. F. Ferard and P. Eullaffroy, Environ. Toxicol., 2008, 23, 591–598 CrossRef CAS PubMed.
- B. Nowack, R. M. David, H. Fissan, H. Morris, J. A. Shatkin, M. Stintz, R. Zepp and D. Brouwer, Environ. Int., 2013, 59, 1–11 CrossRef CAS PubMed.
- J. H. Liu, S. T. Yang, H. Wang and Y. Liu, J. Nanosci. Nanotechnol., 2010, 10(12), 8469–8481 CrossRef CAS PubMed.
- G. Oberdörster, J. Intern. Med., 2010, 267(1), 89–105 CrossRef PubMed.
- T. Papp, D. Schiffmann, D. Weiss, V. Castranova, V. Vallyathan and Q. Rahman, Nanotoxicology, 2008, 2, 9–27 CrossRef CAS.
- A. D. Maynard, R. J. Aitken, T. Butz, V. Colvin, K. Donaldson, G. Oberdorster, M. A. Philbert, J. Ryan, A. Seaton, V. Stone, S. S. Tinkle, L. Tran, N. J. Walker and D. B. Warheit, Nature, 2006, 444, 267–269 CrossRef CAS PubMed.
- G. Oberdörster, E. Oberdorster and J. Oberdorster, Environ. Health Perspect., 2005, 113, 823–839 CrossRef.
- E. Heister, V. Vera Neves, C. Lamprecht, R. P. Silva, H. M. Coley and J. McFadden, Carbon, 2012, 50(2), 622–632 CrossRef CAS.
- H. C. Tsai, J. Y. Lin, F. Maryani, C. C. Huang and T. Imae, Int. J. Nanomed., 2013, 8(1), 4427–4440 CrossRef PubMed.
- C. Klumpp, K. Kostarelos, M. Prato and A. Bianco, Biochim. Biophys. Acta, 2006, 1758(3), 404–412 CrossRef CAS PubMed.
- Y. Wu, J. A. Phillips, H. Liu, R. Yang and W. Tan, ACS Nano, 2008, 2(10), 2023–2028 CrossRef CAS PubMed.
- P. Davide, R. Singh, D. McCarthy, M. Erhardt, J. P. Briand, M. Prato, K. Kostarelos and A. Bianco, Angew. Chem., Int. Ed., 2004, 43(39), 5242–5246 CrossRef PubMed.
- N. W. Shi Kam, Z. Liu and H. Dai, Angew. Chem., Int. Ed., 2006, 45(4), 577–581 CrossRef PubMed.
- Q. Lu, J. M. Moore, G. Huang, A. S. Mount, A. M. Rao, L. L. Larcom and P. C. Ke, Nano Lett., 2004, 4(12), 2473–2477 CrossRef CAS.
- Z. Zhang, X. Yang, Y. Zhang, B. Zeng, S. Wang, T. Zhu, R. B. Roden, Y. Chen and R. Yang, Clin. Cancer Res., 2006, 12(16), 4933–4939 CrossRef CAS PubMed.
- D. Pantarotto, J. P. Briand, M. Prato and A. Bianco, Chem. Commun., 2004, 16–27 RSC.
- N. W. Shi Kam and H. Dai, J. Am. Chem. Soc., 2005, 127(16), 6021–6026 CrossRef PubMed.
- D. Pantarotto, C. D. Partidos, R. Graff, J. Hoebeke, J. P. Briand, M. Prato and A. Bianco, J. Am. Chem. Soc., 2003, 125, 6160–6164 CrossRef CAS PubMed.
- C. D. Partidos, J. Hoebeke, S. Wieckowski, O. Chaloin, A. Bianco, E. Moreau, J. P. Briand, C. Desgranges and S. Muller, Methods, 2009, 49(4), 328–333 CrossRef CAS PubMed.
- A. Bianco, J. Hoebeke, S. Godefroy, O. Chaloin, D. Pantarotto, J. P. Briand, S. Muller, M. Prato and C. D. Partidos, J. Am. Chem. Soc., 2005, 127(1), 58–59 CrossRef CAS PubMed.
- F. Yang, D. L. Fu, J. Long and Q. X. Ni, Med. Hypotheses, 2008, 70(4), 765–767 CrossRef CAS PubMed.
- E. Heister, V. Neves, C. Tılmaciu, K. Lipert, V. S. Beltran, H. M. Coley, S. R. P. Silva and J. McFadden, Carbon, 2009, 47, 2152–2160 CrossRef CAS.
- P. Chaudhuri, S. Soni and S. Sengupta, Nanotechnology, 2010, 21(2), 025102 CrossRef PubMed.
- R. Li, R. Wu, L. Zhao, M. Wu, L. Yang and H. Zou, ACS Nano, 2010, 4, 1399–1408 CrossRef CAS PubMed.
- C. Samor, H. Ali-Boucetta, R. Sainz, C. Guo, M. F. Toma, C. Fabbro, T. Da Ros, M. Prato, K. Kostarelos and A. Bianco, Chem. Commun., 2010, 46, 1494–1496 RSC.
- H. Li, N. Zhang, Y. Hao, Y. Wang, S. Jia, H. Zhang, Y. Zhang and Z. Zhang, Drug Delivery, 2014, 21(5), 379–387 CrossRef CAS PubMed.
- T. Shiraki, A. Dawn, N. L. L. Thi, Y. Tsuchiya, S. Tamaru and S. Shinkai, Chem. Commun., 2011, 47, 7065–7067 RSC.
- N. W. ShiKam, M. O'Connell, J. A. Wisdom and H. Dai, Proc. Natl. Acad. Sci. U. S. A., 2005, 102(33), 11600–11605 CrossRef PubMed.
- J. W. Kim, E. I. Galanzha, V. Evgeny, E. V. Shashkov, H. M. Moon and V. P. Zharov, Nat. Nanotechnol., 2009, 4, 688–694 CrossRef CAS PubMed.
- A. Zerda, C. Zavaleta, S. Keren, S. Vaithilingam, S. Bodapati, Z. Liu, J. Levi, T. J. Ma, O. Oralkan, Z. Cheng, X. Chen, H. Dai, B. T. K. Yakub and S. S. Gambhir, Nat. Nanotechnol., 2008, 3(9), 557–562 CrossRef PubMed.
- F. Zhou, D. Xing, Z. Ou, B. Wu, D. E. Resasco and W. R. Chen, J. Biomed. Opt., 2009, 14(2), 021009 CrossRef PubMed.
- F. L. Qu, M. H. Yang, J. H. Jiang, G. L. Shen and Q. Yu, Anal. Biochem., 2005, 344, 108–114 CrossRef CAS PubMed.
-
Y. Lin, F. Lu, J. Wang, Electroanal (Invited, Special Issue: Nanotechnology), 2004, 16, 145–149.
- X. W. Tang, S. Bansaruntip, N. Nakayama, E. Yenilmez, Y. L. Chang and Q. Wang, Nano Lett., 2006, 6, 1632–1636 CrossRef CAS PubMed.
- J. Li, H. T. Ng, A. Cassell, W. Fan, H. Chen, Q. Ye, J. Koehne, J. Han and M. Meyyappan, Nano Lett., 2003, 3, 597–602 CrossRef CAS.
- X. Yu, B. Munge, V. Patel, G. Jensen, A. Bhirde, J. D. Gong, S. N. Kim, J. Gillespie, J. S. Gutkind, F. Papadimitrakopoulos and J. F. Rusling, J. Am. Chem. Soc., 2006, 128, 11199–11205 CrossRef CAS PubMed.
- C. L. Justino, T. A. R. Santos and A. C. Duarte, Trends Anal. Chem., 2013, 45, 24–36 CrossRef CAS.
- E. H. Espinosa, R. Ionescu, B. Chambon, G. Bedis, E. Sotter, C. Bittencourt, A. Felten, J. J. Pireaux, X. Correig and E. Llobet, Sens. Actuators, B, 2007, 127(1), 137 CrossRef CAS.
- N. V. Hieu, L. T. B. Thuy and N. D. Chien, Sens. Actuators, B, 2008, 129(2), 888–895 CrossRef.
- L. P. Zanello, B. Zhao, H. Hu and R. C. Haddon, Nano Lett., 2006, 6(3), 562–567 CrossRef CAS PubMed.
- E. Mooney, P. Dockery, U. Greiser, M. Murphy and V. Barron, Nano Lett., 2008, 8(8), 2137–2143 CrossRef CAS PubMed.
- J. Venkatesan, Z. J. Qian, B. Ryu, N. A. Kumar and S. K. Kim, Carbohydr. Polym., 2011, 83, 569–577 CrossRef CAS.
- F. Yu, Y. Wu, J. Ma and C. Zhang, J. Environ. Sci., 2013, 25(1), 195–203 CrossRef CAS.
- S. A. Ntim and S. Mitra, J. Colloid Interface Sci., 2012, 375, 154–159 CrossRef PubMed.
- J. Deng, Y. Shao, N. Gao, Y. Deng, C. Tan, S. Zhou and X. Hu, Chem. Eng. J., 2012, 193–194, 339–347 CrossRef CAS.
- Y. Li, Q. Du, T. Liu, X. Peng, J. Wang, J. Sun, Y. Wang, S. Wu, Z. Wang, Y. Xia and L. Xia, Chem. Eng. Res. Des., 2013, 91(2), 361–368 CrossRef CAS.
- Z. Wang, G. Luo, J. Chen, S. Xiao and Y. Wang, Electrophoresis, 2003, 24, 4181–4188 CrossRef CAS PubMed.
- F. Jiang, Y. Wang, X. Hu, N. Shao, J. R. Delanghe and J. Ouyang, J. Sep. Sci., 2010, 33(21), 3393–3399 CrossRef CAS PubMed.
- J. D. Schiffman and E. Menachem, ACS Appl. Mater. Interfaces, 2011, 3(2), 462–468 CAS.
- C. D. Vecitis, M. H. Schnoor, M. S. Rahaman, J. D. Schiffman and M. Elimelech, Environ. Sci. Technol., 2011, 45, 3672–3679 CrossRef CAS PubMed.
- M. Benincasa, S. Pacor, W. Wu, M. Prato, A. Bianco and R. Gennaro, ACS Nano, 2011, 5(1), 199–208 CrossRef CAS PubMed.
- V. P. Torchilin, Handb. Exp. Pharmacol., 2010, 197, 3–53 CAS.
- X. Wang and Y. Wang, Cancer Res. Treat., 2009, 41(1), 1–11 CrossRef CAS PubMed.
- I. Goyal, S. Srivastava and K. Tankeshwar, Nano-Micro Lett., 2012, 4(3), 154–157 CrossRef.
- R. Devi, S. Srivastava and K. Tankeshwar, J. Chem. Phys., 2015, 143(2), 024506 CrossRef PubMed.
- N. Aggarwal, J. Sood and K. Tankeshwar, Nanotechnology, 2007, 18(33), 335707 CrossRef.
- R. Devi, J. Sood, S. Srivastava and K. Tankeshwar, Microfluid. Nanofluid., 2010, 9(4), 737–742 CrossRef.
- R. Devi, S. Srivastava and K. Tankeshwar, Nano Biomed. Eng., 2011, 3(1), 47–52 CAS.
- V. P. Torchilin, Nat. Rev. Drug Discovery, 2014, 13, 813–827 CrossRef CAS PubMed.
- E. G. Kelley, J. N. Albert, M. O. Sullivan and T. H. Epps III, Chem. Soc. Rev., 2013, 42, 7057–7071 RSC.
- Y. Shen, X. Fu, W. Fu and Z. Li, Chem. Soc. Rev., 2015, 44, 612–622 RSC.
-
G. Caneba, S. Verlag and B. Heidelberg, 2010, 1, ISBN 978-3-642-03024-6.
- D. Schmaljohann, Adv. Drug Delivery Rev., 2006, 58, 1655–1670 CrossRef CAS PubMed.
- S. D. Fitzpatrick, L. E. Fitzpatrick, A. Thakur, M. A. J. Mazumder and H. Sheardown, Expert Rev. Med. Devices, 2012, 9, 339–351 CrossRef CAS PubMed.
- M. Freeman, A. Arrott and J. Watson, J. Appl. Phys., 1960, 31, S404–S405 CrossRef.
- S. Louguet, B. Rousseau, R. Epherre, N. Guidolin, G. Goglio, S. Mornet, E. Duguet, S. Lecommandoux and C. Schatz, Polym. Chem., 2012, 3, 1408–1417 RSC.
- B. Koppolu, Z. Bhavsar, A. S. Wadajkar, S. Nattama, M. Rahimi, F. Nwariaku and K. T. Nguyen, J. Biomed. Nanotechnol., 2012, 8, 983–990 CrossRef CAS PubMed.
- J. S. Im, B. C. Bai and Y. S. Lee, Biomaterials, 2010, 31, 1414–1419 CrossRef CAS PubMed.
- J. Jiang, B. Qi, M. Lepage and Y. Zhao, Macromolecules, 2007, 40, 790–792 CrossRef CAS.
- H. Zhao, E. S. Sterner, E. B. Coughlin and P. Theato, Macromolecules, 2012, 45, 1723–1736 CrossRef CAS.
- B. Yan, J. C. Boyer, N. R. Branda and Y. Zhao, J. Am. Chem. Soc., 2011, 133, 19714–19717 CrossRef CAS PubMed.
- L. Wang, M. Zhang, N. Zhang, J. Shi, H. Zhang, M. Li, C. Lu and Z. Zhang, Int. J. Nanomed., 2011, 6, 2641–2652 CrossRef CAS PubMed.
- J. You, R. Zhang, G. Zhang, M. Zhong, Y. Liu, C. S. Van Pelt, D. Liang, W. Wei, A. K. Sood and C. Li, J. Controlled Release, 2012, 158(2), 319–328 CrossRef CAS PubMed.
- S. H. Hu, R. H. Fang, Y. W. Chen, B. J. Liao, I. W. Chen and S. Y. Chen, Adv. Funct. Mater., 2014, 24, 4144–4155 CrossRef CAS.
- S. Thamphiwatana, V. Fu, J. Zhu, D. Lu, W. Gao and L. Zhang, Langmuir, 2013, 29, 12228–12233 CrossRef CAS PubMed.
- P. Rajpoot, V. Bali and K. Pathak, Int. J. Pharm., 2012, 426, 219–230 CrossRef CAS PubMed.
- D. Pornpattananangkul, S. Olson, S. Aryal, M. Sartor, C. M. Huang, K. Vecchio and L. Zhang, ACS Nano, 2010, 4(4), 1935–1942 CrossRef CAS PubMed.
- G. Gao, H. M. J. Park, Y. Li, G. H. Im, J.-H. Kim, H. N. Kim, J. W. Lee, P. Jeon, O. Y. Bang, J. H. Lee and D. S. Lee, Biomaterials, 2012, 33, 9157–9164 CrossRef CAS PubMed.
- F. Meng, R. Cheng, C. Deng and Z. Zhong, Mater. Today, 2012, 15, 436–442 CrossRef CAS.
- M. Aon, S. Cortassa and B. O'Rourke, Biochim. Biophys. Acta, Bioenerg., 2010, 1797, 865–877 CrossRef CAS PubMed.
- W. R. Zhao, H. T. Zhang, Q. J. He, Y. S. Li, J. L. Gu and L. Li, Chem. Commun., 2011, 47, 9459–9461 RSC.
- Y. Yao, L. Zhao, J. Yang and J. Yang, Biomacromolecules, 2012, 1837–1844 CrossRef CAS PubMed.
- L. Zhao, J. X. Ding, C. S. Xiao, P. He, Z. H. Tang, X. Pang, X. L. Zhuang and X. S. Chen, J. Mater. Chem., 2012, 22, 12319–12328 RSC.
- B. Kang, J. Li, S. Chang, M. Dai, C. Ren, Y. Dai and D. Chen, Small, 2012, 8(5), 777–782 CrossRef CAS PubMed.
- A. Servant, C. Bussy, K. A. Jamal and K. Kostarelos, J. Mater. Chem. B, 2013, 1, 4593–4600 RSC.
- G. Cirillo, S. Hampel, U. G. Spizzirri, O. I. Parisi, N. Picci and F. Iemma, BioMed Res. Int., 2014, 2014, 1–17 CrossRef PubMed.
- M. Mohseni, K. Gilani, Z. Bahrami, N. Bolourchian and S. A. Mortazavi, Iran. J. Pharm. Res., 2015, 14(2), 359–371 CAS.
- X. Peng, Q. Zhuang, D. Peng, Q. Dong, L. Tan, F. Jiao, L. Liu, J. Liu, C. Zhao and X. Wang, Iran. J. Pharm. Res., 2013, 12(4), 581–586 CAS.
- A. Giri, M. Bhowmick, S. Pal and A. Bandyopadhyay, Int. J. Biol. Macromol., 2011, 49(5), 885–893 CrossRef CAS PubMed.
- A. Giri, T. Bhunia, S. R. Mishra, L. Goswami, A. B. Panda and A. Bandyopadhyay, RSC Adv., 2014, 4, 13546–13556 RSC.
- A. Giri, T. Bhunia, S. R. Mishra, L. Goswami, A. B. Panda and A. Bandyopadhyay, RSC Adv., 2015, 5, 41736–41744 RSC.
- T. Bhunia, A. Giri, T. Nasim, D. Chattopadhyay and A. Bandyopadhyay, Carbon, 2013, 52, 305–315 CrossRef CAS.
- A. Giri, T. Bhunia, A. Pal, L. Goswami and A. Bandyopadhyay, Eur. Polym. J., 2016, 74, 13–25 CrossRef CAS.
- H. Li, J. He, Y. Zhao, G. Wang and Q. Wei, J. Inorg. Organomet. Polym. Mater., 2011, 21(4), 890–892 CrossRef CAS.
- G. Cirillo, O. Vittorio, S. Hampel, F. Iemma, P. Parchi, M. Cecchini, F. Puoci and N. Picci, Eur. J. Pharm. Sci., 2013, 49(3), 359–365 CrossRef CAS PubMed.
- J. Wu, K. S. Paudel, C. Strasinger, D. Hammell, A. L. Stinchcomb and B. J. Hinds, Proc. Natl. Acad. Sci. U. S. A., 2010, 107, 11698–11702 CrossRef CAS PubMed.
- A. Servant, L. Methven, R. P. Williams and K. Kostarelos, Adv. Healthcare Mater., 2013, 2, 806–811 CrossRef CAS PubMed.
- B. Mandal, D. Das, A. P. Rameshbabu, S. Dhara and S. Pal, RSC Adv., 2016, 6, 19605–19611 RSC.
- Z. Liu, W. B. Cai, L. N. He, N. Nakayama, K. Chen, X. M. Sun, X. Y. Chen and H. J. Dai, Nat. Nanotechnol., 2007, 2, 47–52 CrossRef CAS PubMed.
- M. R. McDevitt, D. Chattopadhyay, B. J. Kappel, J. S. Jaggi, S. R. Schiffman, C. Antczak, J. T. Njardarson, R. Brentjens and D. A. Scheinberg, J. Nucl. Med., 2007, 48, 1180–1189 CrossRef CAS PubMed.
-
K. T. Cheng, M. R. McDevitt and D. A. Scheinberg, Molecular Imaging and Contrast Agent Database (MICAD), National Center for Biotechnology Information (US), Bethesda (MD), 2007, pp. 2004–2013 Search PubMed.
-
K. T. Cheng, J. Guo and X. Zhang, Molecular Imaging and Contrast Agent Database (MICAD), National Center for Biotechnology Information (US), Bethesda (MD), 2007, pp. 2004–2013 Search PubMed.
- J. T. Wang, C. Fabbro, E. Venturelli, C. Ménard-Moyon, O. Chaloin, T. D. Ros, L. Methven, A. Nunes, J. K. Sosabowski, S. J. Mather, M. K. Robinson, J. Amadou, M. Prato, A. Bianco, K. Kostarelos and K. T. A. Jamal, Biomaterials, 2014, 35(35), 9517–9528 CrossRef CAS PubMed.
- H. Wu, G. Liu, X. Wang, J. Zhang, Y. Chen, J. Shi, H. Yang, H. Hu and S. Yang, Acta Biomater., 2011, 7(9), 3496–3504 CrossRef CAS PubMed.
- N. W. S. Kam, Z. Liu and H. Dai, Angew. Chem., Int. Ed., 2006, 45, 577–581 CrossRef CAS PubMed.
- K. Kostarelos, L. Lacerda, G. Pastorin, W. Wu, S. Wieckowski, J. Luangsivilay, S. Godefroy, D. Pantarotto, J. P. Briand, S. Muller, M. Prato and A. Bianco, Nat. Nanotechnol., 2007, 2, 108–113 CrossRef CAS PubMed.
- R. Singh, D. Pantarotto, L. Lacerda, G. Pastorin, C. Klumpp, M. Prato, A. Bianco and K. Kostarelos, Proc. Natl. Acad. Sci. U. S. A., 2006, 103, 3357–3362 CrossRef CAS PubMed.
- L. Lacerda, H. Ali-Boucetta, M. A. Herrero, G. Pastorin, A. Bianco, M. Prato and K. Kostarelos, Nanomedicine, 2008, 3, 149–161 CrossRef CAS PubMed.
- L. Lacerda, H. Ali-Boucetta, M. A. Herrero, G. Pastorin, A. Bianco, M. Prato and K. Kostarelos, Nanomedicine, 2008, 3(2), 149–161 CrossRef CAS PubMed.
- C. Fabbro, H. Ali-Boucetta, T. D. Ros, K. Kostarelos, A. Bianco and M. Prato, Chem. Commun., 2012, 48(33), 3911–3926 RSC.
- D. J. Lim, M. Sim, L. Oh, K. Lim and H. Park, Arch. Pharmacal Res., 2014, 37(1), 43–52 CrossRef CAS PubMed.
- R. P. Feazell, N. Nakayama-Ratchford, H. Dai and S. J. Lippard, J. Am. Chem. Soc., 2007, 129, 8438–8439 CrossRef CAS PubMed.
- S. Dhar, Z. Liu, J. Thomale, H. Dai and S. J. Lippard, J. Am. Chem. Soc., 2008, 130(34), 11467–11476 CrossRef CAS PubMed.
- H. Huang, Q. Yuan, J. S. Shah and R. D. K. Misra, Adv. Drug Delivery Rev., 2011, 63, 1332–1339 CrossRef CAS PubMed.
- J. E. Podesta, K. T. A-Jamal, M. A. Herrero, B. Tian, H. Ali-Boucetta, V. Hegde, A. Bianco, M. Prato and K. Kostarelos, Small, 2009, 5(10), 1176–1185 CrossRef CAS PubMed.
- X. Wang, J. Ren and X. Qu, ChemMedChem, 2008, 3(6), 940–945 CrossRef CAS PubMed.
- J. Qiao, T. Hong, T. S. Triana, H. Guo, C. H. Dai and Y.-Q. Xu, RSC Adv., 2013, 3, 4544–4551 RSC.
- C. Spinato, D. Giust, I. A. Vacchi, C. Moyon, K. Kostarelos and A. Bianco, J. Mater. Chem. B, 2016, 4, 431–441 RSC.
- M. J. O'Connell, S. M. Bachilo, C. B. Huffman, V. C. Moore, M. S. Strano, E. H. Haroz, K. L. Rialon, P. J. Boul, W. H. Noon, C. Kittrell, J. P. Ma, R. H. Hauge, R. B. Weisman and R. E. Smalley, Science, 2002, 297(5581), 593–596 CrossRef PubMed.
- N. W. Shi Kam, M. O'Connell, J. A. Wisdom and H. Dai, Proc. Natl. Acad. Sci. U. S. A., 2005, 102(33), 11600–11605 CrossRef PubMed.
- X. Zhang, L. Meng, Q. Lu, Z. Fei and P. J. Dyson, Biomaterials, 2009, 30, 6041–6047 CrossRef CAS PubMed.
- Z. Liu, X. Sun, N. Nakayama-Ratchford and H. Dai, ACS Nano, 2007, 1, 50–56 CrossRef CAS PubMed.
- P. Jeyamohan, T. Hasumura, Y. Nagaoka, Y. Yoshida, T. Maekawa and D. S. Kumar, Int. J. Nanomed., 2013, 8, 2653–2667 Search PubMed.
- S. Wen, H. Liu, H. Cai, M. Shen and X. Shi, Adv. Healthcare Mater., 2013, 2(9), 1267–1276 CrossRef CAS PubMed.
- D. Yang, F. Yang, J. Hu, J. Long, C. Wang, D. Fu and Q. Ni, Chem. Commun., 2009, 4447–4449 RSC.
- F. Guo, X. Mao, J. Wang, F. Luo and Z. Wang, J. Int. Med. Res., 2011, 39(6), 2217–2227 CrossRef CAS PubMed.
- J. Chen, S. Chen, X. Zhao, L. V. Kuznetsova, S. S. Wong and I. Ojima, J. Am. Chem. Soc., 2008, 130, 16778–16785 CrossRef CAS PubMed.
- Z. Liu, K. Chen, C. Davis, S. Sherlock, Q. Cao, X. Chen and H. Dai, Cancer Res., 2008, 68, 6652–6660 CrossRef CAS PubMed.
- Z. Sobhani, R. Dinarvand, F. Atyabi, M. Ghahremani and M. Adeli, Int. J. Nanomed., 2011, 6705–6719 Search PubMed.
- C. L. Lay, H. Q. Liu, H. R. Tan and Y. Liu, Nanotechnology, 2010, 21(6), 065101 CrossRef PubMed.
- C. Chen, L. Hou, H. Zhang, L. Zhu, H. Zhang, C. Zhang, J. Shi, L. Wang, X. Jia and Z. Zhang, J. Drug Targeting, 2013, 21(9), 809–821 CrossRef CAS PubMed.
- S. Hampel, D. Kunze, D. Haase, K. Krämer, M. Rauschenbach, M. Ritschel, A. Leonhardt, J. Thomas, S. Oswald, V. Hoffmann and B. Büchner, Nanomedicine, 2008, 3, 175–182 CrossRef CAS PubMed.
- H. Li, N. Zhang, Y. Hao, Y. Wang, S. Jia, H. Zhang, Y. Zhang and Z. Zhang, Drug Delivery, 2014, 21(5), 379–387 CrossRef CAS PubMed.
- W. Wu, R. Li, X. Bian, Z. Zhu, D. Ding, X. Li, Z. Jia, X. Jiang and Y. Hu, ACS Nano, 2009, 3, 2740–2750 CrossRef CAS PubMed.
- M. Marsh and H. T. McMahon, Science, 1999, 285(5425), 215–220 CrossRef CAS PubMed.
- R. G. Parton and K. Simons, Nat. Rev. Mol. Cell Biol., 2007, 8(3), 185–194 CrossRef CAS PubMed.
- V. G. Walker, Z. Li, T. Hulderman, D. S. Berry, M. L. Kashon and P. P. Simeonova, Toxicol. Appl. Pharmacol., 2009, 236, 319–328 CrossRef CAS PubMed.
- M. Pacurari, X. J. Yin, J. Zhao, M. Ding, S. S. Leonard, D. S. Berry, B. S. Ducatman, D. Sbarra, M. D. Hoover, V. Castranova and V. Vallyathan, Environ. Health Perspect., 2008, 116, 1211–1217 CrossRef CAS PubMed.
- D. Cui, F. Tian, C. S. Ozkan, M. Wang and H. Gao, Toxicol. Lett., 2005, 155, 73–85 CrossRef CAS PubMed.
- R. Singh, D. Pantarotto, D. McCarthy, O. Chaloin, J. Hoebeke, D. C. Partidos, J. P. Briand, M. Prato, A. Bianco and K. Kostarelos, J. Am. Chem. Soc., 2005, 127(12), 4388–4396 CrossRef CAS PubMed.
- L. Gao, L. Nie, T. Wang, Y. Qin, Z. Guo, D. Yang and X. Yan, ChemBioChem, 2006, 7, 239–242 CrossRef CAS PubMed.
- N. W. S. Kam, Z. Liu and H. Dai, Angew. Chem., Int. Ed., 2006, 45(4), 577–581 CrossRef CAS PubMed.
- T. Ohta, Y. Hashida, F. Yamashita and M. Hashida, J. Pharm. Sci., 2016, 105(9), 2815–2824 CrossRef CAS PubMed.
- D. Cai, J. M. Mataraza, Z. H. Qin, Z. Huang, J. Huang, T. C. Chiles, D. Carnahan, K. Kempa and Z. Ren, Nat. Methods, 2005, 2(6), 449–454 CrossRef CAS PubMed.
- P. Davide, R. Singh, D. McCarthy, M. Erhardt, J. P. Briand, M. Prato, K. Kostarelos and A. Bianco, Angew. Chem., Int. Ed., 2004, 43(39), 5242–5246 CrossRef PubMed.
- Y. Wu, A. J. Phillips, L. Haipeng, R. Yang and W. Tan, ACS Nano, 2008, 2(10), 2023–2028 CrossRef CAS PubMed.
- A. Nunes, N. Amsharov, C. Guo, J. V. D. Bossche, P. Santhosh, K. Karachalios, S. F. Nitodas, M. Burghard, K. Kostarelos and K. T. A. Jamal, Small, 2010, 6(20), 2281–2291 CrossRef CAS PubMed.
- C. Geyik, S. Evran, S. Timur and A. Telefoncu, Biotechnol. Prog., 2014, 30(1), 224–232 CrossRef CAS PubMed.
- Q. Lu, J. M. Moore, G. Huang, A. S. Mount, A. M. Rao, L. L. Larcom and P. C. Ke, Nano Lett., 2004, 4(12), 2473–2477 CrossRef CAS.
- B. Pan, D. Cui, P. Xu, C. Ozkan, G. Feng, M. Ozkan, T. Huang, B. Chu, Q. Li, R. He and G. Hu, Nanotechnology, 2009, 20(12), 125101 CrossRef PubMed.
- Y. Hao, P. Xu, C. He, X. Yang, M. Huang, J. Xing and J. Chen, Nanotechnology, 2011, 22(28), 285103 CrossRef PubMed.
- S. Taghavi, A. H. Nia, F. Mosaffa, S. Askarian, K. Abnous and M. Ramezani, Colloids Surf., B, 2016, 140, 28–39 CrossRef CAS PubMed.
- N. W. S. Kam, L. Zhuang and D. Hongjie, J. Am. Chem. Soc., 2005, 127(36), 12492–12493 CrossRef CAS PubMed.
- R. Yang, X. Yang, Z. Zhang, Y. Zhang, S. Wang, Z. Cai, Y. Jia, Y. Ma, C. Zheng, Y. Lu, R. Roden and Y. Chen, Gene Ther., 2006, 13(24), 1714–1723 CrossRef CAS PubMed.
- Y. P. Huang, I. J. Lin, C. C. Chen, Y. C. Hsu, C. C. Chang and M. J. Lee, Nanoscale Res. Lett., 2013, 8(1), 267 CrossRef PubMed.
- C. Spinato, D. Giust, I. A. Vacchi, C. Ménard-Moyon, K. Kostarelos and A. Bianco, J. Mater. Chem. B, 2016, 4, 431–441 RSC.
- T. Anderson, R. Hu, C. Yang, H. S. Yoon and K.-T. Yong, Biomater. Sci., 2014, 2, 1244–1253 RSC.
- C. Guo, W. T. A. Jamal, F. M. Toma, A. Bianco, M. Prato, K. T. A. Jamal and K. Kostarelos, Bioconjugate Chem., 2015, 26(7), 1370–1379 CrossRef CAS PubMed.
- M. Pescatori, D. Bedognetti, E. Venturelli, C. Ménard-moyon, C. Bernardini, E. Muresu, A. Piana, G. Maida, R. Manetti, F. Sgarrella, A. Bianco and L. Gemma, Biomaterials, 2013, 34, 4395–4403 CrossRef CAS PubMed.
- D. Zhao, D. Alizadeh, L. Zhang, W. Liu, O. Farrukh, E. Manuel, D. J. Diamond and B. Badie, Clin. Cancer Res., 2011, 17(4), 771–782 CrossRef CAS PubMed.
- L. G. Delogu, E. Venturelli, R. Manetti, C. Carru, R. Madeddu, L. Murgia, F. Sgarrella and A. Bianco, Nanomedicine, 2012, 7, 231–243 CrossRef CAS PubMed.
- C. H. Villa, T. Dao, I. Ahearn, N. Fehrenbacher, E. Casey, D. Rey, T. Korontsvit, V. Zakhaleva, C. Batt, M. R. Philips and D. Scheinberg, ACS Nano, 2011, 5, 5300–5311 CrossRef CAS PubMed.
- R. Gottardi and B. Douradinha, J. Nanobiotechnol., 2013, 11, 30–37 CrossRef PubMed.
- D. Pantarotto, C. D. Partidos, J. Hoebeke, F. Brown, E. Kramer, J. P. Briand, S. Muller, M. Prato and A. Bianco, Chem. Biol., 2003, 10, 961–966 CrossRef CAS PubMed.
- D. M. Klinman, Nat. Rev. Immunol., 2004, 4, 249–258 CrossRef CAS PubMed.
- C. C. Chou, H. Y. Hsiao, Q. S. Hong, C. H. Chen, Y. W. Peng, H. W. Chen and P. C. Yang, Nano Lett., 2008, 8, 437–445 CrossRef CAS PubMed.
- M. Matloubian, R. J. Concepcion and R. Ahmed, J. Virol., 1994, 68, 8056–8063 CAS.
- W. Barchet, S. Oehen, P. Klenerman, D. Wodarz, G. Bocharov, A. L. Lloyd, M. A. Nowak, H. Hengartner, R. M. Zinkernagel and S. Ehl, Eur. J. Immunol., 2000, 30, 1356–1363 CrossRef CAS PubMed.
- D. Pantarotto, C. D. Partidos, R. Graff, J. Hoebeke, J. P. Briand, M. Prato and A. Bianco, J. Am. Chem. Soc., 2003, 125, 6160–6164 CrossRef CAS PubMed.
- R. L. Coffman, A. Sher and R. A. Seder, Immunity, 2010, 33(4), 492–503 CrossRef CAS PubMed.
- D. E. Speiser, D. Liénard, N. Rufer, V. R. Godoy and D. Rimoldi, J. Clin. Invest., 2005, 115, 739–746 CrossRef CAS PubMed.
-
A. Bianco, W. Wei, G. Pastorin, C. Klumpp, L. Lacerda, C. D. Partidos, K. Kostarelos and M. Prato, Nanomaterials for Medical Diagnosis and Therapy, 2007, vol. 10, pp. 85–142 Search PubMed.
- H. Fan, I. X. Zhang, L. Chen, H. Zhang, A. D. Wang, E. R. Fonseca, D. J. Manuel, A. D. Raubitschek and B. Badie, Clin. Cancer Res., 2012, 18(20), 5628–5638 CrossRef CAS PubMed.
- G. Hong, S. Diao, A. L. Antaris and H. Dai, Chem. Rev., 2015, 115(19), 10816–10906 CrossRef CAS PubMed.
-
A. Liu and H. Qiu, in Application of CNTs in Biosensing and Nanomedicine. Graphene, Carbon Nanotubes and Nanostructures: Techniques and Applications, ed. J. E. Morris and K. Iniewski, Taylor & Francis Group, Boca Raton, London, New York, 2016 Search PubMed.
- Z. Qin and J. C. Bischof, Chem. Soc. Rev., 2012, 41, 1191–1217 RSC.
- E. Boisselier and D. Astruc, Chem. Soc. Rev., 2009, 38, 1759–1782 RSC.
- L. Dykman and N. Khlebtsov, Chem. Soc. Rev., 2012, 41, 2256–2282 RSC.
- X. Huang and M. A. E. Sayed, J. Adv. Res., 2010, 1, 13–28 CrossRef.
- X. Wang, Y. Pang, G. Ku, X. Xie, G. Stoica and L. V. Wang, Nat. Biotechnol., 2003, 21(7), 803–806 CrossRef CAS PubMed.
- J. T. Oh, M. L. Li, H. F. Zhang, K. Maslov, G. Stoica and L. V. Wang, J. Biomed. Opt., 2006, 11(3), 34032 CrossRef PubMed.
- H. F. Zhang, K. Maslov, G. Stoica and L. V. Wang, J. Biomed. Opt., 2006, 11(5), 054033 CrossRef PubMed.
- C. Haisch, Anal. Bioanal. Chem., 2009, 393(2), 473–479 CrossRef CAS PubMed.
- J. Jose, R. G. Willemink, W. Steenbergen, C. H. Slump, T. G. Leeuwen and S. Manohar, Med. Phys., 2012, 39(12), 7262–7271 CrossRef PubMed.
- J. R. Rajian, G. Girish and X. Wang, J. Biomed. Opt., 2012, 17(9), 96013–96021 CrossRef PubMed.
- P. Wang, P. Wang, H. W. Wang and J. X. Cheng, J. Biomed. Opt., 2012, 17(9), 96010–96011 Search PubMed.
- C. Kim, S. Park, J. Kim, S. Lee, C. Lee, M. Jeon, J. Kim and K. Oh, J. Biomed. Opt., 2013, 18(1), 10501 CrossRef PubMed.
- G. Xu, J. R. Rajian, G. Girish, M. J. Kaplan, J. B. Fowlkes, P. L. Carson and X. Wang, J. Biomed. Opt., 2013, 18(1), 10502 CrossRef PubMed.
- C. Kim, E. C. Cho, J. Chen, K. H. Song, L. Au, C. Favazza, Q. Zhang, C. M. Cobley, F. Gao, Y. Xia and L. V. Wang, ACS Nano, 2010, 4(8), 4559–4564 CrossRef CAS PubMed.
- E. I. Galanzha, E. Shashkov, M. Sarimollaoglu, K. E. Beenken, A. G. Basnakian, M. E. Shirtliff, J. W. Kim, M. S. Smeltzer and V. P. Zharov, PLoS One, 2012, 7(9), e45557 CAS.
- L. Xiang, Y. Yuan, D. Xing, Z. Ou, S. Yang and F. Zhou, J. Biomed. Opt., 2009, 14(2), 021008 CrossRef PubMed.
- J. W. Kim, E. I. Galanzha, E. V. Shashkov, H. M. Moon and V. P. Zharov, Nat. Nanotechnol., 2009, 4, 688–694 CrossRef CAS PubMed.
- X. Wang, C. Wang, L. Cheng, S. T. Lee and Z. Liu, J. Am. Chem. Soc., 2012, 134, 7414–7422 CrossRef CAS PubMed.
- C. Wang, J. Li, C. Amatore, Y. Chen, H. Jiang and X. M. Wang, Angew. Chem., Int. Ed., 2011, 50, 11644–11648 CrossRef CAS PubMed.
- S. H. Hu, Y. W. Chen, W. T. Hung, I. W. Chen and S. Y. Chen, Adv. Mater., 2012, 24, 1748–1754 CrossRef CAS PubMed.
- G. Wang, F. Zhang, R. Tian, L. Zhang, G. Fu, L. Yang and L. Zhu, ACS Appl. Mater. Interfaces, 2016, 8(8), 5608–5617 CAS.
- L. Xie, G. Wang, H. Zhou, F. Zhang, Z. Guo, C. Liu, X. Zhang and L. Zhu, Biomaterials, 2016, 103, 219–228 CrossRef CAS PubMed.
- M. E. Brennan, J. N. Coleman, A. Drury, B. Lahr, T. Kobayashi and W. J. Blau, Opt. Lett., 2003, 28(4), 266–268 CrossRef CAS PubMed.
- C. J. Gannon, P. Cherukuri, B. I. Yakobson, L. Cognet, J. S. Kanzius, C. Kittrell, R. B. Weisman, M. Pasquali, H. K. Schmidt, R. E. Smalley and S. A. Curley, Cancer, 2007, 110(12), 2654–2665 CrossRef CAS PubMed.
- S. V. Torti, F. Byrne, O. Whelan, N. Levi, B. Ucer, M. Schmid, F. M. Torti, S. Akman, J. Liu, P. M. Ajayan, O. Nalamasu and D. L. Carroll, Int. J. Nanomed., 2007, 2(4), 707–714 CAS.
- F. Zhou, D. Xing, Z. Ou, B. Wu, D. E. Resasco and W. R. Chen, J. Biomed. Opt., 2009, 14(2), 021009 CrossRef PubMed.
- N. H. Levi-Polyachenko, E. J. Merkel, B. T. Jones, D. L. Carroll and J. H. Stewart, Mol. Pharmaceutics, 2009, 6(4), 1092–1099 CrossRef CAS PubMed.
- C.-H. Wang, Y.-J. Huang, C.-W. Chang, W.-M. Hsu and C. A. Peng, Nanotechnology, 2009, 20(31), 315101 CrossRef PubMed.
- A. Burke, X. Ding, R. Singh, R. A. Kraft, N. Levi-Polyachenko, M. N. Rylander, C. Szot, C. Buchanan, J. Whitney, J. Fisher, H. C. Hatcher, R. D'Agostino Jr., N. D. Kock, P. M. Ajayan, D. L. Carroll, S. Akman, F. M. Torti and S. V. Torti, Proc. Natl. Acad. Sci. U. S. A., 2009, 106, 12897 CrossRef CAS PubMed.
- S. Ghosh, S. Dutta, E. Gomes, D. Carroll, R. D'Agostino, J. J. Olson, M. Guthold and W. H. Gmeiner, ACS Nano, 2009, 3, 2667 CrossRef CAS PubMed.
- Y. Xiao, X. Gao, O. Taratula, S. Treado, A. Urbas, R. D. Holbrook, R. E. Cavicchi, C. T. Avedisian, S. Mitra, R. Savla, P. D. Wagner, S. Srivastava and H. Heet, BMC Cancer, 2009, 9, 351 CrossRef PubMed.
- S. Burlaka, S. Lukin, S. Prylutska, O. Remeniak, Y. Prylutskyy, M. Shuba, S. Maksimenko, U. Ritter and P. Scharff, Exp. Oncol., 2010, 32(1), 48–50 Search PubMed.
- N. Huang, H. Wang, J. Zhao, H. Lui, M. Korbelik and H. Zeng, Lasers Surg. Med., 2010, 42(9), 638–648 CrossRef PubMed.
- H. T. Chou, T. P. Wang, C. Y. Lee, N. H. Tai and H. Y. Chang, Mater. Sci. Eng., C, 2013, 33(2), 989–995 CrossRef CAS PubMed.
- L. Wang, J. Shi, H. Zhang, H. Li, Y. Gao, Z. Wang, H. Wang, L. Li, C. Zhang, C. Chen, Z. Zhang and Y. Zhang, Biomaterials, 2013, 34(1), 262–274 CrossRef CAS PubMed.
- N. W. S. Kam, M. O'Connell, J. A. Wisdom and H. Dai, Proc. Natl. Acad. Sci. U. S. A., 2005, 102, 11600 CrossRef CAS PubMed.
-
J. D. Jackson, Classsical electrodynamics, John Wiley and Sons, New York, 3rd edn, 1999 Search PubMed.
- P. Chakravarty, R. Marches, N. S. Zimmerman, A. D. E. Swafford, P. Bajaj, I. H. Musselman, P. Pantano, R. K. Draper and E. S. Vitetta, Proc. Natl. Acad. Sci. U. S. A., 2008, 105, 8697 CrossRef CAS PubMed.
- C. H. Wang, Y. J. Huang, C. W. Chang, W. M. Hsu and C. A. Peng, Nanotechnology, 2009, 20, 315101 CrossRef PubMed.
- N. Shao, S. Lu, E. Wickstrom and B. Panchapakesan, Nanotechnology, 2007, 18, 315101 CrossRef.
- H. K. Moon, S. H. Lee and H. C. Choi, ACS Nano, 2009, 3, 3707 CrossRef CAS PubMed.
- L. Zhang, P. Rong, M. Chen, S. Gao and L. Zhu, Nanoscale, 2015, 7(39), 16204–16213 RSC.
- Q. Wang, W. L. Li, H. Y. Zou, H. Liu and C. Z. Huang, New J. Chem., 2016, 40, 6315–6324 RSC.
- R. M. Stevens, Mater. Today, 2009, 12(10), 42–45 CrossRef CAS.
- N. R. Wilson and J. V. Macpherson, Nat. Nanotechnol., 2009, 4, 483–491 CrossRef CAS PubMed.
- D. Keller and C. Chou, Surf. Sci., 1992, 268, 333–339 CrossRef CAS.
- Y. Akama, E. Nishimura, A. Sakai and H. Murakami, J. Vac. Sci. Technol., A, 1990, 8, 429–433 CAS.
- M. Zhao, V. Sharma, H. Wei, R. R. Birge, J. A. Stuart, F. Papadimitrakopoulos and B. D. Huey, Nanotechnology, 2008, 19(23), 235704 CrossRef PubMed.
- C. Bustamante, D. Keller and G. Yang, Curr. Opin. Struct. Biol., 1993, 3, 363–372 CrossRef CAS.
- H. Dai, J. H. Hafner, A. G. Rinzler, D. T. Colbert and R. E. Smalley, Nature, 1996, 384, 147–150 CrossRef CAS.
- S. S. Wong, J. D. Harper, P. T. Lansbury and C. M. Lieber, J. Am. Chem. Soc., 1998, 120, 603–604 CrossRef CAS.
- S. S. Wong, A. T. Woolley, T. W. Odom, J. L. Huang, P. Kim, D. V. Vezenov and C. M. Lieber, Appl. Phys. Lett., 1998, 73, 3465–3467 CrossRef CAS.
- H. J. Dai, N. Franklin and J. Han, Appl. Phys. Lett., 1998, 73, 1508–1510 CrossRef CAS.
- H. Nishijima, S. Kamo, S. Akita, Y. Nakayama, K. I. Hohmura, S. H. Yoshimura and K. Takeyasu, Appl. Phys. Lett., 1999, 74, 4061–4063 CrossRef CAS.
- J. K. Campbell, L. Sun and R. M. Crooks, J. Am. Chem. Soc., 1999, 121, 3779–3780 CrossRef CAS.
- S. S. Wong, E. Joselevich, A. T. Woolley, C. L. Cheung and C. M. Lieber, Nature, 1998, 394, 52–55 CrossRef CAS PubMed.
- S. S. Wong, A. T. Woolley, E. Joselevich, C. L. Cheung and C. M. Lieber, J. Am. Chem. Soc., 1998, 120, 8557–8558 CrossRef CAS.
- J. H. Hafner, C. L. Cheung and C. M. Lieber, Nature, 1999, 398, 761–762 CrossRef CAS.
- C. L. Cheung, J. H. Hafner and C. M. Lieber, Proc. Natl. Acad. Sci. U. S. A., 2000, 97(8), 3809–3813 CrossRef CAS PubMed.
- H. J. Yi, D. Ghosh, M. H. Ham, J. F. Qi, P. W. Barone, M. S. Strano and A. M. Belcher, Nano Lett., 2012, 12, 1176–1183 CrossRef CAS PubMed.
- Q. Li, T. Y. Ohulchanskyy, R. L. Liu, K. Koynov, D. Q. Wu, A. Best, R. Kumar, A. Bonoiu and P. N. Prasad, J. Phys. Chem. C, 2010, 114, 12062–12068 CAS.
- S. Iwabuchii, T. Mori, K. Ogawa, K. Sato, M. Saito, Y. Morita, T. Ushiki and E. Tamiya, Arch. Histol. Cytol., 2002, 65(5), 473–479 CrossRef PubMed.
- R. M. Stevens, C. V. Nguyen and M. Meyyappan, IEEE Trans Nanobioscience, 2004, 3(1), 56–60 CrossRef PubMed.
- J. E. Koehne, R. M. Stevens, T. Zink, Z. Deng, H. Chen, I. C. Weng, F. T. Liu and G. Y. Liu, Ultramicroscopy, 2011, 111(8), 1155–1162 CrossRef CAS PubMed.
- S. Kumar, W. Ahlawat, R. Kumar and N. Dilbaghi, Biosens. Bioelectron., 2015, 70, 498–503 CrossRef CAS PubMed.
- H. Shi, T. Xia, A. E. Nel and J. I. Yeh, Nanomedicine, 2007, 2, 599–614 CrossRef CAS PubMed.
- T. Dastagir, E. S. Forzani, R. Zhang, I. Amlani, L. A. Nagahara, R. Tsuib and N. Tao, Analyst, 2007, 132, 738–740 RSC.
- J. Wang and M. Musameh, Anal. Chem., 2003, 75(9), 2075–2079 CrossRef CAS PubMed.
- B. Pérez, M. Pumera, M. del Valle, A. Merkoi and S. Alegret, J. Nanosci. Nanotechnol., 2005, 5(10), 1694–1698 CrossRef.
- G. Liu and Y. Lin, Electrochem. Commun., 2006, 8, 251–256 CrossRef CAS.
- X. Pang, D. He, S. Luo and Q. Cai, Sens. Actuators, B, 2009, 137, 134–138 CrossRef.
- J. Lin, C. He, Y. Zhao and S. Zhang, Sens. Actuators, B, 2009, 137, 768–773 CrossRef CAS.
- J. C. Claussen, A. D. Franklin, A. U. Haque, D. M. Porterfield and T. S. Fisher, ACS Nano, 2009, 3, 37–44 CrossRef CAS PubMed.
- L. Meng, P. Wu, G. Chen, C. Cai and Y. Sun, Biosens. Bioelectron., 2009, 24, 1751–1756 CrossRef CAS PubMed.
- Y. Gao, C. Shen, J. Di and Y. Tu, Mater. Sci. Eng., C, 2009, 29, 2213–2216 CrossRef CAS.
- Z. H. Wen, S. Q. Ci and J. H. Li, J. Phys. Chem. C, 2009, 113, 13482–13487 CAS.
- W. Zhang, L. L. Wang, N. Zhang, W. F. Wang and B. Fang, Electroanalysis, 2009, 21, 2325–2330 CrossRef CAS.
- R. B. Rakhi, K. Sethupathi and S. R. Prabhu, J. Phys. Chem. B, 2009, 113, 3190–3194 CrossRef CAS PubMed.
- C. Hu, Y. Ding, Y. Ji, J. Xu and S. Hu, Carbon, 2010, 48, 1345–1352 CrossRef CAS.
- S. Palanisamy, S. Cheemalapati and S. M. Chen, Int. J. Electrochem. Sci., 2012, 7, 8394–8407 CAS.
- J. H. Kim, S. A. Jun, Y. Kwon, S. Ha, B. Y. Sang and J. Kim, Bioelectrochemistry, 2015, 101, 114–119 CrossRef CAS PubMed.
- S. Sanchez, M. Pumera, E. Cabruja and E. F`abregas, Analyst, 2007, 132(2), 142–147 RSC.
- J. Wang, G. Liu and Y. Lin, Analyst, 2006, 131, 477–483 RSC.
- P. N. Manikandan, H. Imrana and V. Dharuman, Anal. Methods, 2016, 8, 2691–2697 RSC.
- M. Tominaga, S. Nomura and I. Taniguchi, Biosens. Bioelectron., 2009, 24, 1184–1188 CrossRef CAS PubMed.
- Y. K. Park, B. Bold, W. K. Lee, M. H. Jeon, K. H. An, S. Y. Jeong and Y. K. Shim, Int. J. Mol. Sci., 2011, 12(5), 2946–2957 CrossRef CAS PubMed.
- S. Alwarappan, G. Liu and C. Z. Li, Nanomedicine, 2010, 6(1), 52–57 CAS.
- Z. A. Alothman, N. Bukhari, S. M. Wabaidur and S. Haider, Sens. Actuators, B, 2010, 146, 314–320 CrossRef CAS.
- R. Manjunatha, G. S. Suresh, J. S. Melo, S. F. D'Souza and T. V. Venkatesha, Sens. Actuators, B, 2010, 145, 643–650 CrossRef CAS.
- A. Azadbakht, M. Roushani, A. R. Abbasi, S. Menati and Z. Derikv, Mater. Sci. Eng., C, 2016, 68, 585–593 CrossRef CAS PubMed.
- W. Xue and T. Cui, Sens. Actuators, B, 2008, 134, 981–987 CrossRef CAS.
- M. Kesik, F. E. Kanik, J. Turan, M. Kolb, S. Timur, M. Bahadir and L. Toppare, Sens. Actuators, B, 2014, 205, 39–49 CrossRef CAS.
- J. Ding, H. Zhang, F. Jia, W. Qin and D. Du, Sens. Actuators, B, 2014, 199, 284–290 CrossRef CAS.
- H. C. Yi, D. Y. Zheng, C. G. Hu and S. S. Hu, Electroanalysis, 2008, 20, 1143–1146 CrossRef CAS.
- H. R. Zare and N. Nasirizadeh, Sens. Actuators, B, 2010, 143, 666–672 CrossRef CAS.
- H. Li, X. Q. Ma, F. Ye, J. Zhang, X. Zhou, Z. H. Wang, Y. M. Li and G. Y. Zhang, J. Zhejiang Univ., Sci., B, 2009, 10(3), 203–210 CrossRef CAS PubMed.
- L. Meng, P. Wu, G. Chen, C. Cai and Y. Sun, Biosens. Bioelectron., 2009, 24, 1751–1756 CrossRef CAS PubMed.
- B. Batra and C. S. Pundir, Biosens. Bioelectron., 2013, 47, 496–501 CrossRef CAS PubMed.
- S. Lata, B. Batra, P. Kumar and C. S. Pundir, Anal. Biochem., 2013, 437(1), 1–9 CrossRef CAS PubMed.
- S. Lata, B. Batra, N. Singala and C. S. Pundir, Sens. Actuators, B, 2013, 188, 1080–1088 CrossRef CAS.
- J. P. Kim, B. Y. Lee, S. Hong and S. J. Sim, Anal. Biochem., 2008, 381, 193–198 CrossRef CAS PubMed.
- R. A. Villamizar, J. Braun, B. Gompf, M. Dressel and F. X. Rius, Biosens. Bioelectron., 2009, 25, 161–166 CrossRef CAS PubMed.
- K. Maehashi, K. Matsumoto, Y. Takamura and E. Tamiya, Electroanalysis, 2009, 21, 1285–1290 CrossRef CAS.
- Y. T. Chang, J. H. Huang, M. C. Tu, P. Chang and T. R. Yew, Biosens. Bioelectron., 2013, 15(41), 898–902 CrossRef PubMed.
- Y. Wang and J. Li, Anal. Chim. Acta, 2009, 650(1), 49–53 CrossRef CAS PubMed.
- S. Sánchez, M. Roldán, S. Pérez and E. Fàbregas, Anal. Chem., 2008, 80(17), 6508–6514 CrossRef PubMed.
- T. Yuichi, M. Kenzo, M. Kazuhiko, C. Miyuki, T. Yuzuru and T. Eiichi, Jpn. J. Appl. Phys., 2009, 48, 6S Search PubMed.
- R. K. Gupta, A. Periyakaruppan, M. Meyyappan and J. E. Koehne, Biosens. Bioelectron., 2014, 59, 112–119 CrossRef CAS PubMed.
- Y. E. Choi, J. W. Kwak and J. W. Park, Sensors, 2010, 10(1), 428–455 CrossRef CAS PubMed.
- M. R. Kierny, T. D. Cunningham and B. K. Kay, Nano Rev., 2012, 3, 1–24 CrossRef PubMed.
- C. Aljaro, L. N. Cella, D. J. Shirale, M. Park, F. J. Muñoz, M. V. Yates and A. Mulchandan, Biosens. Bioelectron., 2010, 26(4), 1437–1441 CrossRef PubMed.
- S. Timur, U. Anik, D. Odaci and L. Gorton, Electrochem. Commun., 2007, 9(7), 1810–1815 CrossRef CAS.
- N. Zhou, T. Yang, C. Jiang, M. Du and K. Jiao, Talanta, 2009, 77(3), 1021–1026 CrossRef CAS PubMed.
- J. Li, Q. Liu, Y. Liu, S. Liu and S. Yao, Anal. Biochem., 2005, 346(1), 107–114 CrossRef CAS PubMed.
- R. Manjunatha, G. S. Suresh, J. S. Melo, S. F. D'Souza and T. V. Venkatesha, Sens. Actuators, B, 2010, 145, 643–650 CrossRef CAS.
- S. H. Huang, H. H. Liao and D. H. Chen, Biosens. Bioelectron., 2010, 25, 2351–2355 CrossRef CAS PubMed.
- D. Ragupathy, A. I. Gopalan and K. P. Lee, Sens. Actuators, B, 2010, 143, 696–703 CrossRef CAS.
- A. I. Gopalan, K. P. Lee and D. Ragupathy, Biosens. Bioelectron., 2009, 24, 2211–2217 CrossRef CAS PubMed.
- C. L. Dhand, S. K. Arya, M. Datta and B. D. Malhotra, Anal. Biochem., 2008, 383(2), 194–199 CrossRef CAS PubMed.
- A. A. Ensafi and H. K. Maleh, J. Electroanal. Chem., 2010, 640, 75–83 CrossRef CAS.
- L. Mirmoghtadaie, N. Shamaeizadeh and N. Mirzanasiri, Int. J. Prev. Med., 2015, 6, 100 Search PubMed.
- H. Li, J. He, Q. Wei, S. Li and A. P. F. Turner, Biosens. Bioelectron., 2013, 43, 25–29 CrossRef CAS PubMed.
-
Y. Lin, F. Lu, J. Wang, Electroanal (Invited, Special Issue: Nanotechnology), 2004, 16, 145–149.
- S. Fennouh, V. Casimiri and C. Burstein, Biosens. Bioelectron., 1997, 12, 97–104 CrossRef CAS.
- C. Cremisini, S. Disario, J. Mela, R. Pilloton and G. Palleschi, Anal. Chim. Acta, 1995, 311, 273–280 CrossRef CAS.
- J. Li, H. T. Ng, A. Cassell, W. Fan, H. Chen, Q. Ye, J. Koehne, J. Han and M. Meyyappan, Nano Lett., 2003, 3, 597 CrossRef CAS.
- N. Zhu, Z. Chang, P. He and Y. Fang, Anal. Chim. Acta, 2005, 545, 21–26 CrossRef CAS.
- S. Niu, M. Zhao, L. Hu and S. Zhang, Sens. Actuators, B, 2008, 135(1), 200–205 CrossRef CAS.
- S. Niu, M. Zhao, R. Ren and S. Zhang, J. Inorg. Biochem., 2009, 103(1), 43–49 CrossRef CAS PubMed.
- Y. Zhang, J. Wang and M. Xu, Colloids Surf., B, 2010, 75(1), 179–185 CrossRef CAS PubMed.
- G. Li, X. Li, J. Wan and S. Zhang, Biosens. Bioelectron., 2009, 24(11), 3281–3287 CrossRef CAS PubMed.
- J. Wang, S. Li and Y. Zhang, Electrochim. Acta, 2010, 55(15), 4436–4440 CrossRef CAS.
- M. A. Mehrgardi and L. E. Ahangar, Biosens. Bioelectron., 2011, 26(11), 4308–4313 CrossRef CAS PubMed.
- S. Mohan, P. Srivastava, S. N. Maheshwari, S. Sundar and R. Prakash, Analyst, 2011, 136(13), 2845–2851 RSC.
- J. Nakashima, M. Tachibana, Y. Horiguchi, M. Oya, T. Ohigashi, H. Asakura and M. Murai, Clin. Cancer Res., 2000, 6, 2702–2706 CAS.
- L. S. Angelo, M. Talpaz and R. Kurzrock, Cancer Res., 2002, 62(3), 932–940 CAS.
- T. J. Polascik, J. E. Oesterling and A. W. Partin, J. Urol., 1999, 162, 293–306 CrossRef CAS PubMed.
- S. F. Kingsmore, Nat. Rev. Drug Discovery, 2006, 5, 310–320 CrossRef CAS PubMed.
- J. F. Rusling, C. V. Kumar, J. S. Gutkind and V. Patel, Analyst, 2010, 135, 2496–2511 RSC.
- A. Ghosh and W. D. Heston, J. Cell. Biochem., 2004, 91, 528–539 CrossRef CAS PubMed.
- S. S. Chang, V. E. Reuter, W. D. Heston, N. H. Bander, L. S. Grauer and P. B. Gaudin, Cancer Res., 1999, 59, 3192–3198 CAS.
- Z. Mujagic, H. Mujagic and B. Prnjavorac, Med. Arh., 2004, 58, 23–26 Search PubMed.
- F. Riedel, I. Zaiss, D. Herzog, K. Gotte, R. Naim and K. Hormann, Anticancer Res., 2005, 25, 2761–2765 CAS.
- M. A. St John, Y. Li, X. Zhou, P. Denny, C. M. Ho, C. Montemagno, W. Shi, F. Qi, B. Wu, U. Sinha, R. Jordan, L. Wolinsky, N. H. Park, H. Liu, E. Abemayor and D. T. Wong, Arch. Otolaryngol., Head Neck Surg., 2004, 130(8), 929–935 CrossRef PubMed.
- D. Cervi, T. T. Yip, N. Bhattacharya, V. N. Podust, J. Peterson, A. A. Slaybi, G. N. Naumov, E. Bender, N. Almog, J. E. Italiano Jr., J. Folkman and G. L. Klement, Blood, 2008, 111(3), 1201–1207 CrossRef CAS PubMed.
- G. Cwik, G. Wallner, T. Skoczylas, A. Ciechanski and K. Zinkiewicz, Arch. Surg., 2006, 141(10), 968–973 CrossRef PubMed.
- K. B. Paul, V. Singh, S. R. Vanjari and S. G. Singh, Biosens. Bioelectron., 2016 Search PubMed , S0956–5663(16), 30745–30750.
- K. H. Allin and B. G. Nordestgaard, Crit. Rev. Clin. Lab. Sci., 2011, 48(4), 155–170 CrossRef CAS PubMed.
- R. Bei and G. J. Mizejewski, Curr. Mol. Med., 2011, 11(7), 564–581 CrossRef CAS PubMed.
- G. Bertoli, C. Cava and I. Castiglioni, Int. J. Mol. Sci., 2016, 17(3), 421 CrossRef PubMed.
- C. Yang, C. Wang, X. Chen, S. Chen, Y. Zhang, F. Zhi, J. Wang, L. Li, X. Zhou, N. Li, H. Pan, J. Zhang, K. Zen, C. Y. Zhang and C. Zhang, Int. J. Cancer, 2013, 132(1), 116–127 CrossRef CAS PubMed.
- F. Zhi, N. Shao, R. Wang, D. Deng, L. Xue, Q. Wang, Y. Zhang, Y. Shi, X. Xia, S. Wang, Q. Lan and Y. Yang, Neuro-Oncology, 2015, 17(3), 383–391 Search PubMed.
- C. L. Justino, T. A. R. Santos and A. C. Duarte, TrAC, Trends Anal. Chem., 2013, 45, 24–36 CrossRef CAS.
- X. Yu, B. Munge, V. Patel, G. Jensen, A. Bhirde, J. D. Gong, S. N. Kim, J. Gillespie, J. S. Gutkind, F. Papadimitrakopoulos and J. F. Rusling, J. Am. Chem. Soc., 2006, 128(34), 11199–11205 CrossRef CAS PubMed.
- N. Sardesai, S. M. Pan and J. Rusling, Chem. Commun., 2009, 4968–4970 RSC.
- R. Akter, M. A. Rahman and C. K. Rhee, Anal. Chem., 2012, 84, 6407–6415 CrossRef CAS PubMed.
- J. P. Kim, B. Y. Lee, J. Lee, S. Hong and S. J. Sim, Biosens. Bioelectron., 2009, 24(11), 3372–3378 CrossRef CAS PubMed.
- R. Malhotra, V. Patel, J. P. Vaqué, J. S. Gutkind and J. F. Rusling, Anal. Chem., 2010, 82(8), 3118–3123 CrossRef CAS PubMed.
- Y. Wan, W. Deng, Y. Su, X. Zhu, C. Peng, H. Hu, H. Peng, S. Song and C. Fan, Biosens. Bioelectron., 2011, 30(1), 93–99 CrossRef CAS PubMed.
- J. Lin, C. He, L. Zhang and S. Zhang, Anal. Biochem., 2009, 384, 130–135 CrossRef CAS PubMed.
- W. Jiang, R. Yuan, Y. Q. Chai and B. Yin, Anal. Biochem., 2010, 407(1), 65–71 CrossRef CAS PubMed.
- J. Han, L. Jiang, F. Li, P. Wang, Q. Liu, Y. Dong, Y. Li and Q. Wei, Biosens. Bioelectron., 2016, 77, 1104–1111 CrossRef CAS PubMed.
- B. S. Munge, C. E. Krause, R. Malhotra, V. Patel, J. S. Gutkind and J. F. Rusling, Electrochem. Commun., 2009, 11, 1009–1012 CrossRef CAS PubMed.
- S. Viswanathan, C. Rani, A. V. Anand and J. A. A. Ho, Biosens. Bioelectron., 2009, 24, 1984–1989 CrossRef CAS PubMed.
- H. V. Tran, B. Piro, S. Reisberg, L. D. Tran, H. T. Duc and M. C. Pham, Biosens. Bioelectron., 2013, 49, 164–169 CrossRef CAS PubMed.
- S. Kang, M. Pinault, L. D. Pfefferle and M. Elimelech, Langmuir, 2007, 23, 8670–8673 CrossRef CAS PubMed.
- S. Aslan, M. Deneufchatel, S. Hashmi, N. Li, L. D. Pfefferle, M. Elimelech, E. Pauthe and P. R. T. Van, J. Colloid Interface Sci., 2012, 388, 268–273 CrossRef CAS PubMed.
- S. Aslan, J. Määttä, B. Z. Haznedaroglu, J. P. Goodman, L. D. Pfefferle, M. Elimelech, E. Pauthe, M. Sammalkorpi and P. R. T. Van, Soft Matter, 2013, 9, 2136–2144 RSC.
- C. Yang, J. Mamouni, Y. Tang and L. Yang, Langmuir, 2010, 26(20), 16013–16019 CrossRef CAS PubMed.
- S. Liu, L. Wei, L. Hao, N. Fang, M. W. Chang, R. Xu, Y. Yang and Y. Chen, ACS Nano, 2009, 3, 3891–3902 CrossRef CAS PubMed.
- S. Liu, A. K. Ng, R. Xu, J. Wei, C. M. Tan, Y. Yang and Y. Chen, Nanoscale, 2010, 2(12), 2744–2750 RSC.
- A. Amiri, H. Z. Zardini, M. Shanbedi, M. Maghrebi, M. Baniadam and B. Tolueinia, Mater. Lett., 2012, 72, 153–156 CrossRef CAS.
- M. Benincasa, S. Pacor, W. Wu, M. Prato, A. Bianco and R. Gennaro, ACS Nano, 2011, 5(1), 199–208 CrossRef CAS PubMed.
- V. K. Prajapati, K. Awasthi, S. Gautam, T. P. Yadav, M. Rai, O. N. Srivastava and S. Sundar, J. Antimicrob. Chemother., 2011, 66, 874–879 CrossRef CAS PubMed.
- A. S. B. Estévez, M. H. Schnoor, C. D. Vecitis, N. B. Saleh and M. Elimelech, Langmuir, 2010, 26(18), 14975–14982 CrossRef PubMed.
- C. D. Vecitis, M. H. Schnoor, M. S. Rahaman, J. D. Schiffman and M. Elimelech, Environ. Sci. Technol., 2011, 45(8), 3672–3679 CrossRef CAS PubMed.
- Q. Ma, Y. Qu, W. Shen, J. Wang, Z. Zhang, X. Zhang, H. Zhou and J. Zhou, Water Sci. Technol., 2015, 71(8), 1235–1240 CrossRef CAS PubMed.
- L. Gu, T. Elkin, X. Jiang, H. Li, Y. Lin, L. Qu, T. J. Tzeng, R. Josepha and Y. Sun, Chem. Commun, 2005, 874–876 RSC.
- N. Grover, I. V. Borkar, C. Z. Dinu, R. S. Kane and J. S. Dordick, Enzyme Microb. Technol., 2012, 50(6–7), 271–299 CrossRef CAS PubMed.
- N. Grover, M. P. Douaisi, I. V. Borkar, L. Lee, C. Z. Dinu, R. S. Kane and J. S. Dordick, Appl. Microbiol. Biotechnol., 2013, 9(19), 8813–8821 CrossRef PubMed.
- X. Qi, G. Poernomo, K. Wang, Y. Chen, M. B. C. Park, R. Xu and M. W. Chang, Nanoscale, 2011, 3(4), 1874–1880 RSC.
- X. Dong, E. McCoy, M. Zhang and L. Yang, J. Environ. Sci., 2014, 26(12), 2526–2534 CrossRef PubMed.
- H. Z. Zardini, M. Davarpanah, M. Shanbed, A. Amiri, M. Aghrebi and L. Ebrahimi, J. Biomed. Mater. Res., Part A, 2014, 102(6), 1774–1781 CrossRef PubMed.
- J. Zhou and X. Qi, Lett. Appl. Microbiol., 2011, 52(1), 76–83 CrossRef CAS PubMed.
- H. Z. Zardini, A. Amiri, M. Shanbedi, M. Maghrebi and M. Baniadam, Colloids Surf., B, 2012, 92, 196–202 CrossRef CAS PubMed.
- R. Mohan, A. M. Shanmugharaj and R. S. Hun, J. Biomed. Mater. Res., Part B, 2011, 96(1), 119–126 CrossRef PubMed.
- B. S. Harrison and A. Atala, Biomaterials, 2007, 28, 344–353 CrossRef CAS PubMed.
- Y. Cao, Y. M. Zhou, Y. Shan, H. X. Ju and X. J. Xue, J. Nanosci. Nanotechnol., 2007, 7(2), 447–451 CrossRef CAS PubMed.
- R. A. MacDonald, B. F. Laurenzi, G. Viswanathan, P. M. Ajayan and J. P. Stegemann, J. Biomed. Mater. Res., Part A, 2005, 74(3), 489–496 CrossRef PubMed.
- Z. Tosun and P. S. McFetridge, J. Neural Eng., 2010, 7(6), 66002 CrossRef CAS PubMed.
- E. Mooney, P. Dockery, U. Greiser, M. Murphy and V. Barron, Nano Lett., 2008, 8(8), 2137–2143 CrossRef CAS PubMed.
- J. Venkatesan and S. K. Kim, Mar. Drugs, 2010, 8, 2252–2266 CrossRef CAS PubMed.
- J. Venkatesan, Z. J. Qian, B. Ryu, N. A. Kumar and S. K. Kim, Carbohydr. Polym., 2011, 83, 569–577 CrossRef CAS.
- Q. Chenq, K. Rutledge and E. Jabbarzadeh, Ann. Biomed. Eng., 2013, 41(5), 904–916 CrossRef PubMed.
- S. Namgung, K. Y. Baik, J. Park and S. Hong, ACS Nano, 2011, 5(9), 7383–7390 CrossRef CAS PubMed.
- S. Pok, F. Vitale, S. L. Eichmann, O. M. Benavides, M. Pasquali and J. G. Jacot, ACS Nano, 2014, 8(10), 9822–9832 CrossRef CAS PubMed.
- R. A. MacDonald, B. F. Laurenzi, G. Viswanathan, P. M. Ajayan and J. P. Stegemann, J. Biomed. Mater. Res., 2005, 74, 489–496 CrossRef PubMed.
- M. A. C. Duarte, N. Wagner, J. R. Chapana, C. Morsczeck, M. Thie and M. Giersig, Nano Lett., 2004, 4(11), 2233–2236 CrossRef.
- H. Hu, Y. C. Ni, V. Montana, R. C. Haddon and V. Parpura, Nano Lett., 2004, 4(3), 507–511 CrossRef CAS PubMed.
- Y. Sharma, A. Tiwari, S. Hattori, D. Terada, M. Ramalingam and H. Kobayashi, Int. J. Biol. Macromol., 2012, 51, 627–663 CrossRef CAS PubMed.
- S. F. Wang, L. Shen, W. D. Zhang and Y. J. Tong, Biomacromolecules, 2005, 6, 3067–3072 CrossRef CAS PubMed.
- P. E. Mikael, A. R. Amini, J. Basu, M. J. A. Jimenez, C. T. Laurencin, M. M. Sanders, C. B. Carter and S. P. Nukavarapu, Biomed. Mater., 2014, 9(3), 035001 CrossRef PubMed.
- E. M. Gonalves, F. J. Oliveira, R. F. Silva, M. A. Neto, M. H. Fernandes, M. Amaral, M. V. Regí and M. Vila, J. Biomed. Mater. Res., Part B, 2016, 104(6), 1210–1219 CrossRef PubMed.
- M. Jafari, Z. Paknejad, M. R. Rad, S. R. Motamedian, M. J. Eghbal, N. Nadjmi and A. Khojasteh, J. Biomed. Mater. Res., Part B, 2015 DOI:10.1002/jbm.b.33547.
- C. Lin, Y. Wang, Y. Lai, W. Yang, F. Jiao, H. Zhang, S. Ye and Q. Zhang, Colloids Surf., B, 2011, 83(2), 367–375 CrossRef CAS PubMed.
- N. Goonoo, A. B. Luximon, P. Passanha, S. R. Esteves and D. Jhurry, J. Biomed. Mater. Res., Part B, 2016 DOI:10.1002/jbm.b.33674.
- Z. Wang, G. Luo, J. Chen, S. Xiao and Y. Wang, Electrophoresis, 2003, 24(24), 4181–4188 CrossRef CAS PubMed.
- A. Joshi, S. Punyani, S. S. Bale, H. Yang, T. B. Tasciuc and R. S. Kane, Nat. Nanotechnol., 2008, 3, 41–45 CrossRef CAS PubMed.
- Z. Du, Y. L. Yu, X. W. Chen and J. H. Wang, Chem. – Eur. J., 2007, 13, 9679–9685 CrossRef CAS PubMed.
- A. Chrambach and D. Rodbard, Science, 1971, 172, 440–451 CAS.
- A. L. Shapiro and J. V. Maizel, Anal. Biochem., 1969, 29, 505–514 CrossRef CAS PubMed.
- T. Tanaka, Sci. Am., 1981, 244, 124–138 CrossRef CAS PubMed.
- M. Gunavandhi, L. Arul, A. Maria, V. N. Chamundeswari and M. Parthasarathy, Electrophoresis, 2012, 33(8), 1271–1275 CrossRef PubMed.
- J. J. Simhadri, H. A. Stretz, M. Oyanader and P. E. Arce, Ind. Eng. Chem. Res., 2010, 49(23), 11866–11877 CrossRef CAS.
- G. Huang, Y. Zhang, J. Ouyang, W. R. G. Baeyens and J. R. Delanghe, Anal. Chim. Acta, 2006, 557, 137–145 CrossRef CAS.
- M. Shim, N. W. S. Kam, R. J. Chen, Y. M. Li and H. J. Dai, Nano Lett., 2002, 2, 285–288 CrossRef CAS.
- W. J. Huang, S. Taylor, K. Fu, Y. Lin, D. H. Zhang, T. W. Hanks, A. M. Rao and Y. P. Sun, Nano Lett., 2002, 2, 311–314 CrossRef CAS.
- Z. Du, Y. L. Yu, X. W. Chen and J. H. Wang, Chem. – Eur. J., 2007, 13, 9679–9685 CrossRef CAS PubMed.
- L. E. Valenti, P. A. Fiorito, C. D. García and C. E. J. Giacomelli, J. Colloid Interface Sci., 2007, 307, 349–356 CrossRef CAS PubMed.
- Y. Guo, L. Huang, W. R. G. Baeyens, J. J. R. Delanghe, D. He and J. Ouyang, Nano Lett., 2009, 9(4), 1320–1324 CrossRef CAS PubMed.
- F. Jiang, Y. Wang, X. Hu, N. Shao, J. R. Delanghe and J. Ouyang, J. Sep. Sci., 2010, 33(21), 3393–3399 CrossRef CAS PubMed.
- M. Parthasarathy, J. Debgupta, B. Kakade, A. A. Ansary, M. I. Khan and V. K. Pillai, Anal. Biochem., 2011, 409(2), 230–235 CrossRef CAS PubMed.
- J. Du, C. Ge, Y. Liu, R. Bai, D. Li, Y. Yang, L. Liao and C. Chen, J. Nanosci. Nanotechnol., 2011, 11(11), 10102–10110 CrossRef CAS PubMed.
- M. Gunavadhi, L. A. Maria, V. N. Chamundeswari and M. Parthasarathy, Electrophoresis, 2012, 33(8), 1271–1275 CrossRef CAS PubMed.
- A. Hirsch and O. Vostrowsky, Top. Curr. Chem., 2005, 245, 193–237 CAS.
- J. Chen, M. A. Hamon, H. Hu, Y. Chen, A. M. Rao, P. C. Eklund and R. C. Haddon, Science, 1998, 282(5386), 95–98 CrossRef CAS PubMed.
- J. Zhang, H. Zou, Q. Qing, Y. Yang, Q. Li, Z. Liu, X. Guo and Z. Du, J. Phys. Chem. B, 2003, 107(16), 3712–3718 CrossRef CAS.
- M. Sano, A. Kamino, J. Okamura and S. Shinkai, Science, 2001, 293, 1299–1301 CrossRef CAS PubMed.
- E. T. Mickelson, C. B. Huffman, A. G. Rinzler, R. E. Smalley, R. H. Hauge and J. L. Margrave, Chem. Phys. Lett., 1998, 296(1–2), 188–194 CrossRef CAS.
- V. N. Khabashesku, W. E. Billups and J. L. Margrave, Acc. Chem. Res., 2002, 35(12), 1087–1095 CrossRef CAS PubMed.
- S. Osuna, M. T. Sucarrat, M. Solà, P. Geerlings, C. P. Ewels and G. V. Lier, J. Phys. Chem. C, 2010, 114(8), 3340–3345 CAS.
- J. M. Yuan, X. H. Chen, X. H. Chen, Z. F. Fan, X. G. Yang and Z. H. Chen, Carbon, 2008, 46(9), 1266–1269 CrossRef CAS.
- J. Barkauskas, I. Stankevičiene and A. Selskis, Sep. Purif. Technol., 2010, 71(3), 331–336 CrossRef CAS.
- I. Pełech, R. Pełech, U. Narkiewicz, D. Moszyński, A. Jdrzejewska and B. Witkowski, J. Nanomater., 2013, 12, 1–9 CrossRef.
- L. G. Bulusheva, A. V. Okotrub, E. Flahaut, I. P. Asanov, P. N. Gevko, V. O. Koroteev, Y. V. Fedoseeva, A. Yaya and C. P. Ewels, Chem. Mater., 2012, 24(14), 2708–2715 CrossRef CAS.
- V. E. Diyuk, A. N. Zaderko, K. I. Veselovska and V. Lisnyak, J. Therm. Anal. Calorim., 2015, 120, 1665–1678 CrossRef CAS.
- J. Strauch, B. Anis and C. A. Kuntscher, Phys. Status Solidi B, 2014, 251, 2378–2383 CrossRef CAS.
- K. Kan, T. Xia, L. Li, H. Bi, H. Fu and K. Shi, Nanotechnology, 2009, 20(18), 185502 CrossRef PubMed.
- P. Lu, R. Zhou, W. Guo and X. C. Zeng, J. Phys. Chem. C, 2012, 116(25), 13722–13730 CAS.
- M. H. Hsu, H. Chuang, F. Y. Cheng, Y. P. Huang, C. C. Han, K. C. Pao, S. C. Chou, F. K. Shieh, F. Y. Tsai, C. C. Lin, D. S. Wu and C. C. Chang, RSC Adv., 2014, 4, 14777–14780 RSC.
- S. A. Curran, J. Cech, D. Zhang, J. L. Dewald and S. Roth, J. Mater. Res., 2006, 21(4), 1012–1018 CrossRef CAS.
- G. P. Miller, J. Kintigh, E. Kim, P. F. Weck, S. Berber and D. Tomanek, J. Am. Chem. Soc., 2008, 130, 2296–2303 CrossRef CAS PubMed.
- A. V. Talyzin, S. Luzan, I. V. Anoshkin, A. G. Nasibulin, H. Jiang, E. I. Kauppinen, V. M. Mikoushkin, V. V. Shnitov, D. E. Marchenko and D. Noréus, ACS Nano, 2011, 5(6), 5132–5140 CrossRef CAS PubMed.
- P. Umek, J. W. Seo, K. Hernadi, A. Mrzel, P. Pechy, D. D. Mihailovic and L. Forró, Chem. Mater., 2003, 15(25), 4751–4755 CrossRef CAS.
- B. Vanhorenbeke, C. Vriamont, F. Pennetreau, M. Devillers, O. Riant and S. Hermans, Chem. – Eur. J., 2013, 19(3), 852–856 CrossRef CAS PubMed.
- H. F. Bettinger, Chem. – Eur. J., 2006, 12(16), 4372–4379 CrossRef CAS PubMed.
- R. Kakkar, S. Sharma and B. Badhani, Can. Chem. Trans., 2014, 2(4), 434–449 Search PubMed.
- M. Holzinger, J. Abraham, P. Whelan, R. Graupner, L. Ley, F. Hennrich, M. Kappes and A. Hirsch, J. Am. Chem. Soc., 2003, 125(28), 8566–8580 CrossRef CAS PubMed.
- C. Gao, H. He, L. Zhou, X. Zheng and Y. Zhang, Chem. Mater., 2009, 21(2), 360–370 CrossRef CAS.
- G. Hong, J. C. Lee, J. T. Robinson, U. Raaz, L. Xie, N. F. Huang, J. P. Cooke and H. Dai, Nat. Med., 2012, 18(12), 1841–1846 CrossRef CAS PubMed.
- J. T. Robinson, G. Hong, Y. Liang, B. Zhang, O. K. Yaghi and H. Dai, J. Am. Chem. Soc., 2012, 134(25), 10664–10669 CrossRef CAS PubMed.
- K. Welsher, S. P. Sherlock and H. Dai, Proc. Natl. Acad. Sci. U. S. A., 2011, 108(22), 8943–9848 CrossRef CAS PubMed.
- G. Hong and H. Dai, Methods Mol. Biol., 2016, 1444, 167–181 Search PubMed.
- M. F. Islam, E. Rojas, D. M. Bergey, A. T. Johnson and A. G. Yodh, Nano Lett., 2003, 3(2), 269–273 CrossRef CAS.
- J. R. Yu, N. Grossiord, C. E. Koning and J. Loos, Carbon, 2007, 45(3), 618–623 CrossRef CAS.
- Y. Bai, D. Lin, F. Wu, Z. Wang and B. Xing, Chemosphere, 2010, 79(4), 362–367 CrossRef CAS PubMed.
- P. C. Ma, N. A. Siddiqui, G. Marom and J. K. Kim, Composites, Part A, 2010, 41, 1345–1367 CrossRef.
- J. S. Shim and C. H. Ahn, Biosens. Bioelectron., 2012, 34(1), 208–214 CrossRef CAS PubMed.
- E. Heister, V. Neves, C. Lamprecht, R. P. Silva, H. M. Coley and J. McFadden, Carbon, 2012, 50(2), 622–632 CrossRef CAS.
- M. A. Kadir, J. Park, B. S. Kim, S. G. Lee and H. J. Paik, Isr. J. Chem., 2012, 52(3–4), 359–363 CrossRef.
- H. T. Ham, Y. S. Choi and I. J. Chung, J. Colloid Interface Sci., 2005, 286, 216–223 CrossRef CAS PubMed.
- V. Moore, M. Strano, E. Haroz, R. Hauge and R. Smalley, Nano Lett., 2003, 3, 1379–1382 CrossRef CAS.
- L. Vaisman, H. D. Wagner and G. Marom, Adv. Colloid Interface Sci., 2006, 128–130, 37–46 CrossRef CAS PubMed.
- J. Rausch, R. C. Zhuang and E. Mäder, Composites, Part A, 2010, 41(9), 1038–1046 CrossRef.
- P. S. Goh, B. C. Ng, A. F. Ismail, M. Aziz and S. M. Sanip, Solid State Sci., 2010, 12(12), 2155–2162 CrossRef CAS.
- K. Fu and Y. P. Sun, J. Nanosci. Nanotechnol., 2003, 3, 351–364 CrossRef CAS PubMed.
- K. Ausman, R. Piner, O. Lourie and R. Ruoff, J. Phys. Chem. B, 2000, 104, 8911–8915 CrossRef CAS.
- D. S. Kim, D. Nepal and K. E. Geckeler, Small, 2005, 1, 1117–1124 CrossRef CAS PubMed.
- C. A. Dyke and J. M. Tour, Chem. – Eur. J., 2004, 10, 812–817 CrossRef CAS PubMed.
- K. A. Fernando, Y. Lin and Y. P. Sun, Langmuir, 2004, 20, 4777–4778 CrossRef CAS PubMed.
- H. Peng, L. B. Alemany, J. L. Margrave and V. N. Khabashesku, J. Am. Chem. Soc., 2003, 125, 15174–15182 CrossRef CAS PubMed.
- B. Gigliotti, B. Sakizzie, D. S. Bethune, R. M. Shelby and J. N. Cha, Nano Lett., 2006, 6, 159–164 CrossRef CAS PubMed.
- S. Moulton, A. Minett, R. Murphy, K. Ryan, D. McCarthy, J. Coleman, W. J. Blau and G. G. Wallace, Carbon, 2005, 43(9), 1879–1884 CrossRef CAS.
- M. Zheng, A. Jagota, E. D. Semke, B. A. Diner, R. S. McLean, S. R. Lustig, R. E. Richardson and N. G. Tassi, Nat. Mater., 2003, 2, 338–342 CrossRef CAS PubMed.
- G. R. Dieckmann, A. B. Dalton, P. A. Johnson, J. Razal, J. Chen, G. M. Giordano, E. Munoz, I. H. Musselman, R. H. Baughman and R. Draper, J. Am. Chem. Soc., 2003, 125, 1770–1777 CrossRef CAS PubMed.
- S. S. Karajanagi, H. Yang, P. Asuri, E. Sellitto, J. S. Dordick and R. S. Kane, Langmuir, 2006, 22, 1392–1395 CrossRef CAS PubMed.
- O. V. Kharissova, B. I. Kharisov and E. G. C. Ortiz, RSC Adv., 2013, 3, 24812–24852 RSC.
- F. Tardani, L. Gentile, G. A. Ranieri and C. L. Mesa, J. Phys. Chem. C, 2013, 117(16), 8556–8562 CAS.
- F. Tardani and C. L. Mesa, J. Phys. Chem. C, 2011, 115(19), 9424–9431 CAS.
- X.-S. Wang, Engineering, 2011, 3, 50–54 CrossRef CAS.
- A. J. Blanch, C. E. Lenehan and J. S. Quinton, J. Phys. Chem. B, 2010, 114(30), 9805–9811 CrossRef CAS PubMed.
- S. Ghorabi, L. Rajabi, S. S. Madaeni, S. Zinadini and A. A. Derakhshan, Iran. Polym. J., 2012, 21(2), 121–130 CrossRef CAS.
- A. Mohamed, A. K. Anas, S. A. Bakar, T. Ardyani, W. M. W. Zin, S. Ibrahim, M. Sagisaka, P. Brown and J. Easto, Adv. Colloid Interface Sci., 2015, 455, 179–187 CrossRef CAS PubMed.
- A. Bahader, G. Haoguan, F. H. Goa, W. Ping, W. Shaojun and D. Yunsheng, J. Polym. Res., 2016, 23, 184 CrossRef.
- K. D. Ausman, R. Piner, O. Lourie and R. S. Ruoff, J. Phys. Chem. B, 2000, 104(38), 8911–8915 CrossRef CAS.
- L. Vaisman, G. Marom and H. D. Wagner, Adv. Funct. Mater., 2006, 16, 357 CrossRef CAS.
- A. A. Bhirde, S. Patel, A. A. Sousa, V. Patel, A. A. Molinolo, Y. Ji, R. D. Leapman, J. S. Gutkind and J. F. Rusling, Nanomedicine, 2010, 5(10), 1535–1546 CrossRef CAS PubMed.
- N. S. Azar and M. Pourfath, J. Phys. Chem. C, 2016, 120(30), 16804–16814 CAS.
- G. T. Caneba, C. Dutta, V. Agrawal and M. Rao, J. Miner. Mater. Charact. Eng., 2010, 9(3), 165–181 Search PubMed.
- M. Farbod, S. K. Tadavani and A. Kiasat, Colloids Surf., A, 2011, 384(1–3), 685–690 CrossRef CAS.
- X.-Q. Li, C.-Y. Sun, Q. Zhao, Z.-S. Wu, D.-K. Xu and W.-Y. Zhong, Chem. Res. Chin. Univ., 2013, 34(4), 763–770 CrossRef CAS.
- H. Ltzen, M. Wirts-Rtters and A. Hartwig, Soft Mater., 2012, 10(4), 462–471 CrossRef.
- M. K. Okajima, A. Kumar, A. Fujiwara, T. Mitsumata, D. Kaneko, T. Ogawa, H. Kurata, S. Isoda and T. Kaneko, Biopolymers, 2013, 99(1), 1–9 CrossRef CAS PubMed.
- R. Bandyopadhyaya, E. Nativ-Roth, O. Regev and R. Yerushalmi-Rozen, Nano Lett., 2002, 2, 25–28 CrossRef CAS.
- M. J. O'Connell, P. Boul, L. M. Ericson, C. Huffman, Y. Wang, E. Haroz, C. Kuper, J. Tour, K. V. Ausman and R. E. Smalley, Chem. Phys. Lett., 2001, 342, 265–271 CrossRef.
- Y. Kang and T. A. Taton, J. Am. Chem. Soc., 2003, 125, 5650–5651 CrossRef CAS PubMed.
- M. Yudasaka, M. Zhang, C. Jabs and S. Iijima, Appl. Phys. A: Mater. Sci. Process., 2000, 71, 449–451 CrossRef CAS.
- J. Rausch, R. C. Zhuang and E. Mäder, J. Appl. Polym. Sci., 2010, 117(5), 2583–2590 CAS.
- Q. Zhang, S. Zhang and L. Zhang, J. Mater. Sci. Chem. Eng., 2014, 2, 7–12 CAS.
- N. G. Sahoo, S. Rana, J. W. Cho, L. Li and S. H. Chan, Prog. Polym. Sci., 2010, 35(7), 837–867 CrossRef CAS.
- D. Banerjee, A. Jha and K. K. Chattopadhyay, Macromol. Res., 2012, 20(10), 1021–1028 CrossRef CAS.
- I. Kalinina, K. Worsley, C. Lugo, S. Mandal, E. Bekyarova and R. C. Haddon, Chem. Mater., 2011, 23(5), 1246–1253 CrossRef CAS.
- B. Wang, Y. Han, K. Song and T. Zhang, J. Nanosci. Nanotechnol., 2012, 12(6), 4664–4669 CrossRef CAS PubMed.
- M. T. Kim, H. S. Park, D. Hui and K. Y. Rhee, J. Nanosci. Nanotechnol., 2011, 11(8), 7369–7373 CrossRef CAS PubMed.
- A. Indhuja, K. S. Suganthi, S. Manikandan and K. S. Rajan, J. Taiwan Inst. Chem. Eng., 2013, 44(3), 474–479 CrossRef.
- L. Lu and W. Chen, ACS Nano, 2010, 4(2), 1042–1048 CrossRef CAS PubMed.
- M. G. Adsul, D. A. Rey and D. V. Gokhale, J. Mater. Chem., 2011, 21(7), 2054–2056 RSC.
- O. Rochez, G. Zorzini, J. Amadou, M. Claes and A. Richel, J. Mater. Sci., 2013, 48(14), 4962–4964 CrossRef CAS.
- T. Zhang, L. Zou, X. Ling and X. Li, Appl. Mech. Mater., 2013, 275–277, 1785–1788 CrossRef.
- A. V. Venediktova, A. Y. Vlasov, E. D. Obraztsova, D. A. Videnichev, I. M. Kislyakov and E. P. Sokolova, Appl. Phys. Lett., 2012, 100(25), 251903 CrossRef.
- S. M. Taghdisi, P. Lavaee, M. Ramezani and K. Abnous, Eur. J. Pharm. Biopharm., 2011, 77(2), 200–206 CrossRef CAS PubMed.
- E. N. Primo, P. Canete-Rosales, S. Bollo, M. D. Rubianes and G. A. Rivas, Colloids Surf., B, 2013, 108, 329–336 CrossRef CAS PubMed.
- C. W. Huang, M. G. Mohamed, C. Y. Zhu and S. W. Kuo, Macromolecules, 2016, 49(15), 5374–5385 CrossRef CAS.
- K. Mukhopadhyay, C. D. Dwivedi and G. N. Mathur, Carbon, 2002, 40, 1373–1376 CrossRef CAS.
- F. H. Gojny, M. H. G. Wichmann, U. Köpke, B. Fiedler and K. Schulte, Compos. Sci. Technol., 2004, 64, 2363–2371 CrossRef CAS.
- Y. B. Li, B. Q. Wei, J. Liang, Q. Yu and D. H. Wu, Carbon, 1999, 37, 493–497 CrossRef CAS.
- Y. A. Kim, T. Hayashi, Y. Fukai, M. Endo, T. Yanagisawa and M. S. Dresselhaus, Chem. Phys. Lett., 2002, 355, 279–284 CrossRef CAS.
- P. C. Ma, S. Q. Wang, B. Z. Tang and J. K. Kim, J. Nanosci. Nanotechnol., 2009, 9, 749–753 CrossRef CAS PubMed.
- K. Awasthi, R. Kamalakaran, A. K. Singh and O. N. Srivastava, Int. J. Hydrogen Energy, 2002, 27, 425–432 CrossRef CAS.
- J. K. W. Sandler, M. S. P. Shaffer, T. Prasse, W. Bauhofer, K. Schulte and A. H. Windle, Polymer, 1999, 40, 5967–5971 CrossRef CAS.
- P. He, Y. Gao, J. Lian, L. Wang, D. Qian, J. Zhao, W. Wang, M. J. Schulz, X. P. Zhou and D. Shi, Composites, Part A, 2006, 37, 1270–1275 CrossRef.
- S. C. Yoon, Y. G. Jeong and H. S. Kim, Transactions of Materials Processing, 2006, 15(5), 360–365 CrossRef.
- M. D. H. Beg, A. K. M. M. Alam, R. M. Yunus and M. F. Mina, J. Nanopart. Res., 2015, 17, 53–66 CrossRef.
- H. Yazdani, B. E. Smith and K. Hatami, Composites, Part A, 2016, 82, 65–77 CrossRef CAS.
- Z. Li, G. Luo, W. Zhuo, F. Wei, R. Xiang and Y. Liu, Nanotechnology, 2006, 17, 3692–3698 CrossRef CAS.
- A. Ryabenko, T. Dorofeeva and G. Zvereva, Carbon, 2004, 42, 1523–1535 CrossRef CAS.
- J. Riggs, D. Walker, D. Carroll and Y. Sun, J. Phys. Chem. B, 2000, 104, 7071–7076 CrossRef CAS.
- J. A. Fagan, B. J. Landi, I. Mandelbaum, J. R. Simpson, V. Bajpai, B. J. Bauer, K. Migler, A. R. Walker, R. Raffaelle and E. K. Hobbie, J. Phys. Chem. B, 2006, 110, 23801–23805 CrossRef CAS PubMed.
- J. J. Park, J. A. Fagan, J. Y. Huh, K. B. Migler, A. Karim and D. Raghavan, Soft Matter, 2010, 6(21), 5581 RSC.
- T. Dooher and D. Dixon, Polym. Compos., 2011, 32, 1895–1903 CrossRef CAS.
- P. Kar and A. Choudhury, Sens. Actuators, B, 2013, 183, 25–33 CrossRef CAS.
- G. Alonso-Nunez, L. M. de la Garza, E. Rogel-Hernandez, E. Reynoso, A. Licea-Claverie, R. M. Felix-Navarro, G. Berhault and F. Paraguay-Delgado, J. Nanopart. Res., 2011, 13, 3643–3656 CrossRef CAS.
- A. E. Frise, G. Pages, M. Shtein, I. P. Bar, O. Regev and I. Furo, J. Phys. Chem. B, 2012, 116, 2635–2642 CrossRef CAS PubMed.
- K. Shen, S. Curran, H. Xu, S. Rogelj, Y. Jiang, J. Dewald and T. Pietrass, J. Phys. Chem. B, 2005, 109, 4455–4463 CrossRef CAS PubMed.
- V. Weiss, R. Thiruvengadathan and O. Regev, Langmuir, 2006, 22, 854–856 CrossRef CAS PubMed.
- A. W. Musumeci, E. R. Waclawik and R. L. Frost, Spectrochim. Acta, Part A, 2008, 71(1), 140–142 CrossRef PubMed.
- B. Bauer, E. Bobbie and M. Mecker, Macromolecules, 2006, 39, 2637–2642 CrossRef CAS.
- K. Yurekli, C. A. Mitchell and R. Krishnamoorti, J. Am. Chem. Soc., 2004, 126, 9902–9903 CrossRef CAS PubMed.
- E. S. K. Guyton, L. Chen, S. E. Rogers, T. Cosgrove and J. S. V. Duijneveldt, Langmuir, 2015, 31(10), 3262–3268 CrossRef PubMed.
- E. S. K. Guyton, L. Chen, S. E. Rogers, T. Cosgrove and J. S. V. Duijneveldt, J. Colloid Interface Sci., 2016, 472, 1–7 CrossRef PubMed.
- R. H. Basurto, Á. I. López-Lorente and M. Valcárcel, Microchem. J., 2015, 122, 137–143 CrossRef.
- S. Amemiya, R. Chen, N. Nioradze and J. Kim, Acc. Chem. Res., 2016, 49(9), 2007–2014 CrossRef CAS PubMed.
- F. Solá, Z. H. Xia, M. Lebrón-Colón and M. A. Meador, Phys. Status Solidi RRL, 2012, 8, 349–351 CrossRef.
- D. M. Tang, C. L. Ren, R. Lv, W. Yu, P. X. Hou, M. S. Wang, X. Wei, Z. Xu, N. Kawamoto, Y. Bando, M. Mitome, C. Liu, H. M. Cheng and D. Golberg, Nano Lett., 2015, 15(8), 4922–4927 CrossRef CAS PubMed.
- A. Nel, T. Xia, L. Madler and N. Li, Science, 2006, 311, 622–627 CrossRef CAS PubMed.
- K. Donaldson, V. Stone, C. L. Tran, W. Kreyling and P. J. A. Borm, Occup. Environ. Med., 2004, 61, 727–728 CrossRef CAS PubMed.
- G. Jia, H. F. Wang, L. Yan, X. Wang, R. J. Pei, T. Yan, Y. L. Zhao and X. B. Guo, Environ. Sci. Technol., 2005, 39, 1378–1383 CrossRef CAS PubMed.
- A. Magrez, S. Kasas, V. Salicio, N. Pasquier, J. W. Seo, M. Celio, S. Catsicas, B. Schwaller and L. Forro, Nano Lett., 2006, 6, 1121–1125 CrossRef CAS PubMed.
- S. T. Yang, X. Wang and G. Jia, Toxicol. Lett., 2008, 181(3), 182–189 CrossRef CAS PubMed.
- C. A. Poland, R. Duffin and I. Kinloch, Nat. Nanotechnol., 2008, 3, 423–428 CrossRef CAS PubMed.
- M. L. Schipper, N. N. Ratchford and C. R. Davis, Nat. Nanotechnol., 2008, 3, 216–221 CrossRef CAS PubMed.
- C. S. Sharma, S. Sarkar, A. Periyakaruppan, J. Barr, K. Wise, R. Thomas, B. L. Wilson and G. T. Ramesn, J. Nanosci. Nanotechnol., 2007, 7(7), 2466–2472 CrossRef CAS PubMed.
- P. Møller, D. V. Christophersen, D. M. Jensen, A. Kermanizadeh, M. Roursgaard, N. R. Jacobsen, J. G. Hemmingsen, P. H. Danielsen, Y. Cao, K. Jantzen, H. Klingberg, L. G. Hersoug and S. Loft, Arch. Toxicol., 2014, 88(11), 1939–1964 CrossRef PubMed.
- K. Pulskamp, S. Diabaté and H. F. Krug, Toxicol. Lett., 2007, 168(1), 58–74 CrossRef CAS PubMed.
- J. S. Kim and I. J. Yu, J. Toxicol. Environ. Health, Part A, 2014, 77(19), 1141–1153 CrossRef CAS PubMed.
- J. Muller, F. Huaux, N. Moreau, P. Misson, J. F. Heilier, M. Delos, M. Arras, A. Fonseca, J. B. Nagy and D. Lison, Toxicol. Appl. Pharmacol., 2005, 207(3), 221–231 CrossRef CAS PubMed.
- K. H. Kim, S. M. Yeon, H. G. Kim, H. Lee, S. K. Kim, S. H. Han, K. J. Min, Y. Byun, E. H. Lee, K. S. Lee, S. H. Yuk, U. H. Ha and Y. W. Jung, Inflammation, 2014, 37(1), 44–54 CrossRef CAS PubMed.
- I. Fenoglio, M. Tomatis, D. Lison, J. Muller, A. Fonseca, J. B. Nagy and B. Fubini, Free Radical Biol. Med., 2006, 40, 1227–1233 CrossRef CAS PubMed.
- P. Nymark, K. A. Jensen, S. Suhonen, Y. Kembouche, M. Vippola, J. Kleinjans, J. Catalán, H. Norppa, J. V. Delft and J. J. Briedé, Part. Fibre Toxicol., 2014, 11(4), 1–18 Search PubMed.
- K. Pulskamp, J. M. W. Knirsch, F. Hennrich, K. Kern and H. F. Krug, Carbon, 2007, 45, 2241–2249 CrossRef CAS.
- A. R. Murray, E. Kisin, S. S. Leonard, S. H. Young, C. Kommineni, V. E. Kagan, V. Castranova and A. A. Shvedova, Toxicology, 2009, 257(3), 161–171 CrossRef CAS PubMed.
- O. Zeni, R. Palumbo, L. Zeni, M. Sarti and M. R. Scarfi, Sensors, 2008, 8, 488–499 CrossRef CAS.
- L. Dong, K. L. Joseph, C. M. Witkowski and M. M. Craig, Nanotechnology, 2008, 19(25), 255702 CrossRef PubMed.
- F. Tian, D. Cui, H. Schwarz, G. G. Estrada and H. K. Kobayashi, Toxicol. In Vitro, 2006, 20, 1202–1212 CrossRef CAS PubMed.
- D. Cui, F. Tian, C. S. Ozkan, M. Wang and H. Gao, Toxicol. Lett., 2005, 155, 73–85 CrossRef CAS PubMed.
- Y. Zhang, S. Ali, E. Dervishi, Y. Xu, Z. Li, D. Casciano and A. S. Birls, ACS Nano, 2010, 4(6), 3181–3186 CrossRef CAS PubMed.
- L. Belyanskaya, S. Weigel, C. Hirsch, U. Tobler, H. F. Krug and P. Wick, Neurotoxicology, 2009, 30(4), 702–711 CrossRef CAS PubMed.
- R. Y. Yury, B. U. Daniel, C. M. Bibiana, L. M. Rebeca, G. M. Stuart, C. Bulmaro and A. Arnulfo, Toxicol. In Vitro, 2015, 29(5), 1201–1214 CrossRef PubMed.
- C. C. Chou, H. Y. Hsiao, Q. S. Hong, C. H. Chen, Y. W. Peng, H. W. Chen and P. C. Yang, Nano Lett., 2008, 8(2), 437–445 CrossRef CAS PubMed.
- A. R. Murray, E. Kisin, S. S. Leonard, S. H. Young, C. Kommineni, V. E. Kagan, V. Castranova and A. A. Shvedova, Toxicology, 2009, 257(3), 161–171 CrossRef CAS PubMed.
- G. Oberdörster, E. Oberdorster and J. Oberdorster, Nanotoxicology, 2005, 113, 823–839 Search PubMed.
- P. Biharia, M. Holzera, M. Praetnera, J. Fentb, M. Lerchenbergera, C. A. Reichela, M. Rehberga, S. Lakatosb and F. Krombacha, Toxicology, 2010, 269(2–3), 148–154 CrossRef PubMed.
- T. Thurnherr, C. Brandenberger, K. Fischer, L. Diener, P. Manser, X. Maeder-Althaus, J. P. Kaiser, H. F. Krug, B. R. Rutishauser and P. Wicka, Toxicol. Lett., 2011, 200(3), 176–186 CrossRef CAS PubMed.
- D. Cavallo, C. Fanizza, C. L. Ursini, S. Casciardi, E. Paba, A. Ciervo, A. M. Fresegna, R. Maiello, A. M. Marcelloni, G. Buresti, F. Tombolini, S. Bellucci and S. Iavicoli, J. Appl. Toxicol., 2012, 32(6), 454–464 CrossRef CAS PubMed.
- A. R. Reddy, Y. N. Reddy, D. R. Krishna and V. Himabindu, Toxicology, 2010, 272(1–3), 11–16 CrossRef CAS PubMed.
- N. A. M. Riviere, R. J. Nemanich, A. O. Inman, Y. Y. Wang and J. E. Riviere, Toxicol. Lett., 2005, 155, 377–384 CrossRef PubMed.
- F. A. Witzmann and N. A. M. Riviere, Nanomedicine, 2006, 2(3), 158–168 CAS.
- M. Bottini, S. Bruckner, K. Nika, N. Bottini, S. Bellucci, A. Magrini, A. Bergamaschi and T. Mustelin, Toxicol. Lett., 2006, 160(2), 121–126 CrossRef CAS PubMed.
- A. Patlolla, B. Knighten and P. Tchounwou, Ethn. Dis., 2010, 20(suppl 1), S1-65–72 Search PubMed.
- J. Cheng, C. M. Chan, L. M. Veca, W. L. Poon, P. K. Chan, L. Qu, Y. P. Sun and S. H. Cheng, Toxicol. Appl. Pharmacol., 2009, 235(2), 216–225 CrossRef CAS PubMed.
- T. I. Vitkina, V. I. Yankova, T. A. Gvozdenko, V. L. Kuznetsov, D. V. Krasnikov, A. V. Nazarenko, V. V. Chaika, S. V. Smagin, A. M. Tsatsakis, A. B. Engin, S. P. Karakitsios, D. A. Sarigiannis and K. S. Golokhvast, Food Chem. Toxicol., 2016, 87, 38–147 CrossRef PubMed.
Footnote |
† Electronic supplementary information (ESI) available. See DOI: 10.1039/c6cs00517a |
|
This journal is © The Royal Society of Chemistry 2017 |