DOI:
10.1039/C6CE02122K
(Paper)
CrystEngComm, 2017,
19, 99-109
Zn-MOFs containing flexible α,ω-alkane (or alkene)-dicarboxylates with 1,2-bis(4-pyridyl)ethylene: comparison with Zn-MOFs containing 1,2-bis(4-pyridyl)ethane ligands†
Received
4th October 2016
, Accepted 18th November 2016
First published on 18th November 2016
Abstract
Flexible ditopic α,ω-alkane (or alkene)-dicarboxylate bridging ligands provided the following six Zn-MOFs with a 1,2-bis(4-pyridyl)ethylene (bpe) pillar (malonate (1-bpe), succinate (2-bpe), fumarate (3-bpe), glutarate (4-bpe), adipate (5-bpe), and muconate (6-bpe)). Newly-prepared 5-bpe and 6-bpe were structurally characterized. Both 3-bpe and 6-bpe formed three-dimensional (3-D), diamond-like frameworks with a 4-connected uninodal net and a Schläfli symbol of 66. By contrast, 5-bpe formed a 3-D framework of a 6-connected uninodal net with a Schläfli symbol of 412·63. 2-bpe formed a 2-D framework with a 4-connected uninodal net with a Schläfli symbol of 44·62. 3-bpe and 6-bpe showed potential void spaces after solvent removal. Gas sorption analysis with N2, H2, and CO2 at a suitable temperature indicated that 6-bpe was an adsorbent with selectivity for CO2 adsorption at 196 K over N2 and H2. The CO2 uptake at 196 K was 86.68 cm3 g−1 (3.87 mmol g−1). 3-bpe did not exhibit appreciable uptake levels for N2, H2, and CO2, which was possibly due to the relatively rigid nonporous activated form of the framework after solvent removal. Both 3-bpe and 6-bpe exhibited a good encapsulating ability for molecular iodine in cyclohexane. The crystal structure of I2-containing 6-bpe_I2 was elucidated. A comparison with Zn-MOFs containing 1,2-bis(4-pyridyl)ethane ligands is discussed.
Introduction
Metal–organic frameworks (MOFs) are multidimensional, crystalline solid network materials that often contain large void spaces after the removal of captured solvent molecules.1–4 Well-designed MOFs are suited for a variety of sophisticated applications, including separation,5–7 CO2 capture,8–10 H2 storage,11–13 heterogeneous catalysis,14–16 and biomedical usage.17,18 Among them, the gas sorption ability is one of the most intensively investigated properties of MOFs. Selective sorption of CO2 over N2, H2, and CH4 is important for reducing greenhouse gas levels.19–23 The physicochemical properties of MOFs inherently depend on many factors, such as the metal ion type and the following framework properties: rigidity/flexibility,24–26 chemical functionality,27,28 and geometry.2,29,30 Hence, the bridging ligands have influential effects on the properties of MOFs. MOFs are continuously receiving attention because these factors can be fine-tuned for specific functions. Our group and others recently focused on the less explored α,ω-alkane (or alkene) dicarboxylates as bridging ligands to design new MOFs.31–38 Like the more popular rigid aromatic ring-containing dicarboxylate bridging ligands,1,2 they can be utilized for constructing new functional MOFs. A systematic study of MOFs with these α,ω-alkane (or alkene)-dicarboxylate features will broaden the scope of MOF materials.
Recently, we reported on the use of Cu- and Zn-MOFs constructed from flexible α,ω-alkane (or alkene)-dicarboxylates and 1,2-bis(4-pyridyl)ethane (bpa) ligands.31–33,37–39 Six flexible ditopic α,ω-alkane (or alkene)-dicarboxylates (malonate (1-bpa), succinate (2-bpa), fumarate (3-bpa), glutarate (4-bpa), adipate (5-bpa), and muconate (6-bpa)), shown in Scheme 1, provided two-dimensional (2-D) or 3-D frameworks with bpa ligands.33 The 3-D frameworks of 2-bpa, 3-bpa, 5-bpa, and 6-bpa, which are formulated as [Zn(μ2-O2CRCO2)(μ2-bpa)] with water solvates, are diamond-like networks (a Schläfli symbol of 66) with tetrahedral geometry around a ZnII ion. On the other hand, 1-bpa and 4-bpa, which are formulated as [{Zn(H2O)(O2CRCO2)}2(μ-bpa)] containing coordinated water ligands, were different structures as follows: the 3-D framework of 1-bpa contains octahedral ZnII ions with 1-D channels and 2-D layers of 4-bpa contained penta-coordinated ZnII ions. These results indicated that the coordination geometry and coordination mode play important roles in structure construction.
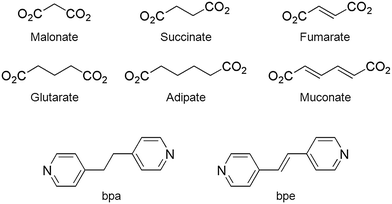 |
| Scheme 1 The chemical structures of α,ω-alkane (or alkene)-dicarboxylates, bpa and bpe linkers. | |
As an extension of our previous work,33 we used six flexible α,ω-alkane (or alkene)-dicarboxylates with a different pillar, 1,2-bis(4-pyridyl)ethylene (bpe), to prepare new functional MOFs with interesting structures. We compare the resulting structures (1-bpe to 6-bpe) with previously prepared 1-bpa to 6-bpa. Although 2-bpe and 3-bpe were previously prepared, and their structures were determined by other groups,37,38 we prepared 2-bpe and 3-bpe again by ourselves to compare the structures and properties of 1-bpe to 6-bpe with 1-bpa to 6-bpa. The bpe pillar has a –CH
CH– bond between two pyridyl groups, while the bpa pillar contains a –CH2CH2– unit, as shown in Scheme 1. Therefore, the bpe pillar is thought to be less flexible than bpa. The distinct rigidity of these two pillars resulted in significantly different framework structures. The gas sorption properties of potentially porous 3-bpe and 6-bpe were also investigated using various gases under suitable conditions. The solvent-free 6-bpe exhibited selectivity for the adsorption of CO2 over N2 and H2.
Experimental
Materials
Succinic acid, fumaric acid, adipic acid, muconic acid, Zn(NO3)2∙6H2O, and 1,2-bis(4-pyridyl)ethylene (bpe) were purchased from Sigma-Aldrich and used as received. Diethylformamide was purchased from TCI and used without further purification. Other reagent grade solvents were used as received.
Instrumentation
Elemental analysis was performed at the Organic Chemistry Research Center, Sogang University (Seoul, Korea) using an EA1112 analyzer (CE Instruments, Italy). Fourier transform infrared (FT-IR) spectra, in the attenuated total reflection (ATR) mode, were obtained on a Jasco FT/IR-4100 spectrometer. UV/vis absorption spectra were measured on a Scinco S-3100 spectrophotometer. Thermogravimetric analyses (TGA) were performed on a Shimadzu TA50 integration thermal analyzer under a constant flow of nitrogen gas. Powder X-ray diffraction (PXRD) patterns were obtained using a Rigaku MiniFlex diffractometer (30 kV, 15 mA, scan speed: 2° min−1, step-size: 0.02°). The volumetric N2 adsorption–desorption analysis was performed with a Belsorp-miniII instrument (BEL Japan) at 77 K. The as-prepared samples were dried at 353 K or 453 K under high vacuum conditions for 2 h. Low-pressure CO2 adsorption–desorption measurements were performed using a Belsorp-miniII at 196 K (2-propanol/dry ice bath), 273 K (ice bath), and 298 K (water bath). High-pressure gas sorption isotherms of 3-bpe and 6-bpe were measured on a PCTpro E&E instrument (SETARAM) equipped with a microdoser attachment. To prepare the sample for high-pressure gas sorption measurements, 3-bpe and 6-bpe were evacuated at 353 K and 453 K, respectively, for 2 h under high vacuum conditions. The dried sample was loaded into a sample holder under Ar atmosphere in a glove box. CO2 isotherm measurements were performed over a pressure range of 0 to 30 atm at 273 K.
[Zn2(μ4-succinate)(μ3-succinate)(μ2-bpe)]·(H2O)4.5 (2-bpe).
A mixture containing Zn(NO3)2·6H2O (0.059 g, 0.2 mmol), 1,2-bis(4-pyridyl)ethylene (bpe, 0.073 g, 0.4 mmol), and succinic acid (0.024 g, 0.2 mmol) in dimethylformamide (DMF, 20 mL) was placed in a Teflon-lined high pressure bomb, which was sealed and heated at 85 °C for 72 h. Then, it was cooled to room temperature. 2-bpe crystals that were suitable for X-ray analysis were obtained. The 2-bpe yield was 10 mg (8%). IR (KBr): ν(cm−1) = 1604(s), 1570(s), 1540(w), 1507(w), 1434(s), 1384(m), 1295(m), 1220(m), 1090(s), 885(s), 840(s), and 685(m). Anal. calcd. for C20H27N2O12.5Zn2 (626.21), 2-bpe: C, 38.36; H, 4.35; and N, 4.47%. Found: C, 38.34; H, 2.96; and N, 4.86%.
[Zn(μ2-fumarate)(μ2-bpe)]·(H2O)0.5(DMF) (3-bpe).
A mixture containing Zn(NO3)2·6H2O (0.059 g, 0.2 mmol), bpe (0.073 g, 0.4 mmol), and fumaric acid (0.023 g, 0.2 mmol) in DMF (10 mL) was placed in a Teflon-lined high pressure bomb, which was sealed and heated at 85 °C for 72 h followed by cooling to room temperature. 3-bpe crystals that were suitable for X-ray analysis were obtained. The yield of 3-bpe was 45 mg (50%). IR (KBr): ν(cm−1) = 1678(s), 1602(s), 1589(s), 1507(w), 1430(m), 1376(s), 1343(s), 1258(w), 1092(m), 1070(w), 1032(w), 842(s), 800(m), 697(s), and 660(m). Anal. calcd. for C19H20N3O5.5Zn (443.77), 3-bpe: C, 51.42; H, 4.54; and N, 9.47%. Found: C, 51.28; H, 4.60; and N, 10.15%.
[Zn(μ4-adipate)0.5(μ2-adipate)0.5(μ2-bpe)]·(H2O) (5-bpe).
A mixture containing Zn(NO3)2·6H2O (0.059 g, 0.2 mmol), bpe (0.073 g, 0.4 mmol), and adipic acid (0.029 g, 0.2 mmol) in DMF/H2O (10 mL, 1
:
1 v
:
v) was placed in a Teflon-lined high pressure bomb, which was sealed and heated at 100 °C for 72 h. It was then cooled to room temperature. 5-bpe crystals that were suitable for X-ray analysis were obtained. The yield of 5-bpe was 12 mg (15%). IR (KBr): ν(cm−1) = 1593(s), 1423(m), 1392(m), 1294(w), 1213(w), 1124(w), 1071(w), 1014(m), 988(w), 833(s), and 766(w). Anal. calcd. for C18H20N2O5Zn (409.75), 5-bpe: C, 52.76; H, 4.92; and N, 6.84%. Found: C, 52.42; H, 4.28; and N, 7.36%.
[Zn(μ2-muconate)(μ2-bpe)]·(H2O)2(DEF) (6-bpe).
A mixture containing Zn(NO3)2·6H2O (0.059 g, 0.2 mmol), bpe (0.073 g, 0.4 mmol), and muconic acid (0.028 g, 0.2 mmol) in diethylformamide (DEF, 10 mL) was placed in a Teflon-lined high pressure bomb, which was sealed and heated at 100 °C for 72 h. The mixture was then cooled to room temperature. 6-bpe crystals that were suitable for X-ray analysis were obtained. The 6-bpe yield was 40 mg (40%). IR (KBr): ν(cm−1) = 1663(m), 1612(s), 1558(m), 1507(w), 1432(m), 1363(s), 1259(w), 1210(w), 1107(w), 1070(m), 1025(m), 1001(m), 955(w), 865(w), 836(m), 748(m), and 698(w). Anal. calcd. for C23H29N3O7Zn (524.88), 6-bpe: C, 52.63; H, 5.57; and N, 8.01%. Found: C, 52.04; H, 4.80; and N, 8.15%.
Iodine encapsulation
To quantify the iodine encapsulation capacity of the as-prepared 3-bpe and 6-bpe, 20 mg of as-prepared samples were immersed in 10 mL of 1.0 mM cyclohexane iodine solution (Aldrich catalog #I3380). The resulting suspension, in a closed vial, was gently shaken in the dark. An aliquot from the top portion of the suspension was periodically withdrawn for UV/vis spectroscopic analysis. The aliquot was centrifuged before measurement (13
000 rpm, 10 min). The measurement was performed in triplicate. After 5 days, X-ray quality crystals were directly chosen from this suspension for X-ray crystallography.
X-ray crystallography
The X-ray diffraction data for all four compounds were collected on a Bruker APEX-II diffractometer that was equipped with a monochromator in the Mo Kα (λ = 0.71073 Å) incident beam. Each crystal was mounted on a glass fiber or put into a Lindemann capillary. The CCD data were integrated and scaled using the Bruker-SAINT software package, and the structure was solved and refined using SHELXTL V6.12.40 All hydrogen atoms were placed in the calculated positions. The crystallographic data for compounds 1-bpe–6-bpe are listed in Table 1. The selected bond distances and angles of compounds 1-bpe–6-bpe are listed in Table 2. Structural information was deposited at the Cambridge Crystallographic Data Center (CCDC reference numbers are 1438600 for 2-bpe, 1438601 for 3-bpe, 1438602 for 5-bpe, 1438604 for 6-bpe, 1481289 for 3-bpe_I2, and 1481290 for 6-bpe_I2).
Table 1 Crystallographic data for compounds 1-bpe–6-bpe, I2-encapsulated 3-bpe_I2 and 6-bpe_I2
|
1-bpea |
2-bpe |
3-bpe |
4-bpeb |
5-bpe |
6-bpe |
3-bpe_I2 |
6-bpe_I2 |
See ref. 32.
See ref. 39.
|
Empirical formula |
C11H8N2O5Zn |
C20H16N2O8Zn2 |
C16H12N2O4.5Zn |
C17H16N2O6Zn |
C18H18N2O4Zn |
C18H14N2O4Zn |
C16H12N2O4Zn |
C18H14N2O4Zn I0.1 |
Formula weight |
313.56 |
543.09 |
369.65 |
413.72 |
391.71 |
387.68 |
361.65 |
400.37 |
Temperature (K) |
170(2) |
296(2) |
296(2) |
293(2) |
296(2) |
293(2) |
296(2) |
293(2) |
Wavelength (Å) |
0.71073 |
0.71073 |
0.71073 |
0.71073 |
0.71073 |
0.71073 |
0.71073 |
0.71073 |
Space group |
Pnn
2
|
P21/c |
C2/c |
P2/n |
P![[1 with combining macron]](https://www.rsc.org/images/entities/char_0031_0304.gif) |
Pnna
|
C2/c |
Pnna
|
a (Å) |
7.3070(15) |
10.6305(8) |
8.8979(3) |
8.0460(16) |
8.1425(2) |
12.396(3) |
8.8583(2) |
12.471(3) |
b (Å) |
24.960(5) |
13.3200(10) |
21.4587(7) |
5.7930(12) |
9.1496(2) |
13.262(3) |
21.4370(5) |
13.306(3) |
c (Å) |
7.2700(15) |
16.2613(12) |
13.4851(4) |
19.177(4) |
11.5118(3) |
15.772(3) |
13.5925(3) |
15.711(3) |
α (°) |
90.00 |
90.00 |
90.00 |
90.00 |
97.1850(16) |
90.00 |
90.00 |
90.00 |
β (°) |
90.00 |
107.408(4) |
96.7804(16) |
97.46(3) |
96.2776(17) |
90.00 |
95.7932(12) |
90.00 |
γ (°) |
90.00 |
90.00 |
90.00 |
90.00 |
102.7454(16) |
90.00 |
90.00 |
90.00 |
Volume (Å3) |
1325.9(5) |
2197.1(3) |
2556.80(14) |
886.3(3) |
821.58(4) |
2592.9(9) |
2567.97(10) |
2607.1(9) |
Z
|
4 |
4 |
4 |
2 |
2 |
4 |
4 |
4 |
Density (calc.) (Mg m−3) |
1.571 |
1.642 |
0.960 |
1.550 |
1.583 |
0.993 |
0.935 |
0.988 |
Absorption coeff. (mm−1) |
1.868 |
2.232 |
0.976 |
1.422 |
1.521 |
0.963 |
0.969 |
0.958 |
Crystal size (mm3) |
0.20 × 0.12 × 0.10 |
0.08 × 0.08 × 0.48 |
0.24 × 0.12 × 0.12 |
0.20 × 0.10 × 0.08 |
0.02 × 0.12 × 0.16 |
0.28 × 0.08 × 0.08 |
0.10 × 0.16 × 0.28 |
0.28 × 0.16 × 0.10 |
Reflections collected |
6804 |
62 550 |
36 532 |
4697 |
19 976 |
76 212 |
43 787 |
62 046 |
Independent reflections |
2533 [R(int) = 0.0400] |
5571 [R(int) = 0.2053] |
3063 [R(int) = 0.0647] |
1741 [R(int) = 0.0226] |
2789 [R(int) = 0.1189] |
3200 [R(int) = 0.1297] |
3173 [R(int) = 0.0549] |
2197 [R(int) = 0.4013] |
Data/restraints/parameters |
2533/17/149 |
5571/4/288 |
3063/0/110 |
1741/2/125 |
2789/0/227 |
3200/0/114 |
3173/0/105 |
2197/0/118 |
Goodness-of-fit on F2 |
1.062 |
0.985 |
1.106 |
1.134 |
1.041 |
0.859 |
1.137 |
1.051 |
Final R indices [I > 2σ(I)] |
R
1 = 0.0433, wR2 = 0.1066 |
R
1 = 0.0739, wR2 = 0.1791 |
R
1 = 0.0494, wR2 = 0.1399 |
R
1 = 0.0299, wR2 = 0.0859 |
R
1 = 0.0650, wR2 = 0.1444 |
R
1 = 0.0753, wR2 = 0.1912 |
R
1 = 0.0412, wR2 = 0.0899 |
R
1 = 0.0894, wR2 = 0.1491 |
R indices (all data) |
R
1 = 0.0570, wR2 = 0.1127 |
R
1 = 0.1686, wR2 = 0.2190 |
R
1 = 0.0596, wR2 = 0.1450 |
R
1 = 0.0326, wR2 = 0.0870 |
R
1 = 0.0892, wR2 = 0.1590 |
R
1 = 0.2084, wR2 = 0.2263 |
R
1 = 0.0549, wR2 = 0.0934 |
R
1 = 0.2318, wR2 = 0.2079 |
Absolute structure parameter |
0.52(5) |
— |
— |
— |
— |
— |
|
|
Extinction coefficient |
— |
0.0380(30) |
— |
— |
0.0920(90) |
— |
|
|
Largest diff. peak and hole (e Å−3) |
0.675 and −0.429 |
0.825 and −0.505 |
0.734 and −0.391 |
0.322 and −0.297 |
0.869 and −0.484 |
0.373 and −0.278 |
0.413 and −0.345 |
0.257 and −0.195 |
CCDC |
887800 |
1438600
|
1438601
|
911186 |
1438602
|
1438604
|
1481289
|
1481290
|
Table 2 Selected bond distances and angles for compounds 1-bpe–6-bpe
|
1-bpea |
2-bpe |
3-bpe |
4-bpeb |
5-bpe |
6-bpe |
See ref. 32.
See ref. 39.
|
Zn–O (Å) |
Zn–Omalo |
Zn1–Osucc |
Zn–Ofuma |
Zn–Oglut |
Zn–Oadip |
Zn–Omuco |
2.085(7)–2.137(3) |
1.936(5)–1.977(5) |
1.9558(19) |
1.9508(15) |
1.993(4)–2.008(4) |
1.993(3), 2.51(1) |
Zn2–Osucc |
2.006(5)–2.424(5) |
Zn–N (Å) |
2.139(3) |
Zn1–N |
2.024(2) |
2.0550(17) |
2.187(4), 2.206(4) |
2.068(3) |
2.004(5) |
Zn2–N |
2.157(5) |
N–Zn–N (°) |
— |
— |
112.50(13) |
103.51(10) |
176.25(18) |
96.17(18) |
O–Zn–O (°) |
85.71(11)–96.18(12) |
89.44(16)–158.5(2) |
99.96(12) |
104.04(10) |
98.50(18)–133.3(2) |
150.0(2) |
Results and discussion
Preparation of Zn-MOFs
To systematically investigate the framework structure variations and gas sorption abilities of potentially porous Zn-MOFs bearing a relatively rigid bpe pillar and a series of flexible α,ω-alkane (or alkene)-dicarboxylate bridging ligands (Scheme 1), four new Zn-MOFs were thermally prepared and structurally characterized by X-ray crystallography. All four Zn-MOFs, 2-bpe, 3-bpe, 5-bpe, and 6-bpe, were obtained as crystalline solids suitable for X-ray analysis. The Fourier-transform infrared (FT-IR) absorption spectra of the as-prepared Zn-MOFs were fully consistent with their formulations based on the crystal structures. Very strong, yet slightly broadened, absorption bands at ∼1678 cm−1 and ∼1400 cm−1 can be assigned as the asymmetric and symmetric C
O stretching modes, respectively, of the coordinated α,ω-alkane (or alkene)-dicarboxylate moieties.41–43 The lack of observable bands in the ∼1710 cm−1 region indicates that full deprotonation of all carboxylic acid bridging ligands occurred during MOF formation. The phase purity of the bulk Zn-MOFs was checked by PXRD. The PXRD patterns of as-prepared Zn-MOFs were in good agreement with the simulated data from X-ray crystallography (Fig. 1). Therefore, each bulk Zn-MOF was proved to be a pure phase.
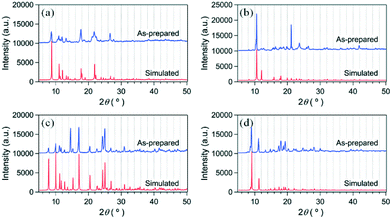 |
| Fig. 1 PXRD patterns of as-prepared Zn-MOFs and simulated patterns from single-crystal X-ray diffraction. (a) 2-bpe, (b) 3-bpe, (c) 5-bpe, and (d) 6-bpe. | |
Structure description
[Zn2(μ4-succinate)(μ3-succinate)(μ2-bpe)]·4.5(H2O) (2-bpe).
The asymmetric unit contains two ZnII ions, two succinate ligands and a bpe ligand. Succinate ligands bridge ZnII ions to form 1-D double chains. These chains are connected by bpe bridging ligands to form 2-D sheets (Fig. 2). The number of solvent water molecules was calculated from elemental analysis and TGA. The coordination mode of one bridging succinate is bridging, and that of the other succinate is chelating/bridging and chelating. The Zn1 ion is penta-coordinate bound by four succinate oxygen atoms (Zn–O 1.936(5)–1.977(5) Å) and a bpe nitrogen atom (Zn–N 2.004(5) Å), and the Zn2 ion is a distorted octahedron that is constructed equatorially by four succinate oxygen atoms (Zn–O 1.977(5)–2.160(5) Å) and axially by succinate oxygen and bpe nitrogen atoms (Zn–O 2.424(5) Å, Zn–N 2.157(5) Å) (Table 2). The structure indicated a 4-connected uninodal net (sql/Shubnikov tetragonal plane net) with a Schläfli symbol of 44·62 assuming Zn2 units as nodes, which act as two nodes without any simplification based on the ToposPro analysis (Table S1†).44
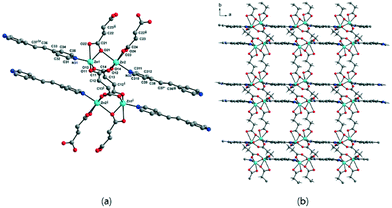 |
| Fig. 2 (a) Environment of the succinate-bridged tetranuclear 2-bpe unit. All hydrogen atoms were omitted. Symmetry codes: i (1 − x, 1 − y, 1 − z), ii (1 − x, 2 − y, 1 − z), iii (1 + x, y, 1 + z), and iv (−1 + x, y, −1 + z). (b) 2-D sheet of 2-bpe. | |
[Zn(μ2-fumarate)(μ2-bpe)]·(H2O)0.5(DMF) (3-bpe).
Two carboxylates from two different fumarates and two pyridyl nitrogen atoms from two different bpe ligands coordinate to a ZnII ion with a distorted tetrahedral geometry, forming a 3-D framework (Fig. 3). The 3-D frameworks are three-fold interpenetrated (Fig. 3c). The coordination modes for carboxyl groups are monodentate. The asymmetric unit contains a ZnII ion, a fumarate, a bpe, and half of a water solvent molecule. The solvent-free 3-D framework indicates a void volume of 49.2% (1258.3 Å3/2558.8 Å3) based on PLATON analysis.45 DMF molecules are present in the voids, but they could not be refined as a result of the disordering problem. The total number of electrons for the disordered DMF molecules can be counted using PLATON SQUEEZE. The estimated number of DMF molecules was calculated from elemental analysis and TGA, which can support the presence of certain DMF guests in the pore. The structure indicates a 4-connected uninodal net with a Schläfli symbol of 66 assuming the ZnII ions act as nodes in the absence of simplification based on ToposPro analysis (Table S2†).44 The N–Zn–N and O–Zn–O bond angles as well as the Zn–O and Zn–N bond distances are listed in Table 2.
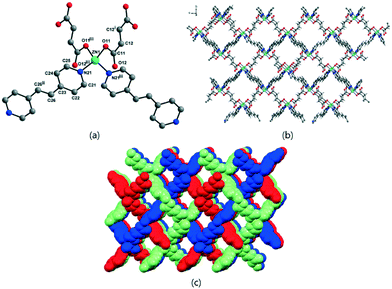 |
| Fig. 3 (a) Tetrahedral geometry around the ZnII ion of 3-bpe. Symmetry codes: i (1.5 − x, 1.5 − y, 2 − z), ii (−x, 1 − y, 2 − z), and iii (1 − x, y, 1.5 − z). All hydrogen atoms were omitted. (b) 3-D framework along the a-axis. (c) The three-fold interpenetrated 3-D frameworks are shown in different colors. Solvent molecules were omitted for clarity. | |
[Zn(μ4-adipate)0.5(μ2-adipate)0.5(μ2-bpe)]·(H2O) (5-bpe).
Two adipates bridge dinuclear units to form 1-D chains, and the other two adipates connect these 1-D chains through chelating coordination of carboxyl groups to form 2-D sheets. The adipate-bridged 2-D sheets are connected by bpe ligands to form a 3-D framework (Fig. 4). The asymmetric unit contains a ZnII ion, adipate, and a bpe ligand. The number of solvent water molecules was calculated from the elemental analysis and TGA. The 3-D frameworks are two-fold interpenetrated (Fig. 4c). The coordination geometry of a ZnII ion is a distorted octahedral constructed by four adipate oxygen atoms and two bpe nitrogen atoms (Fig. 4a).
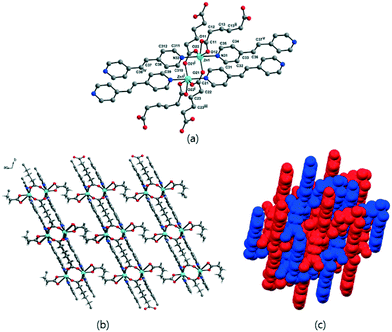 |
| Fig. 4 (a) Environment around the dinuclear unit of 5-bpe. Symmetry codes: i (1 − x, −y, 1 − z), ii (−x, −y, −z), iii (2 − x, 1 − y, 1 − z), iv (x, 1 + y, 1 + z), and v (x, 1 + y, 1 + z). All hydrogen atoms were omitted. (b) 3-D framework. (c) The two-fold interpenetrated 3-D frameworks are shown in different colors. | |
The solvent-free 3-D framework does not contain void volumes. The structure indicates that there is a 6-connected uninodal net with a Schläfli symbol of 412·63 (pcu α-Po primitive cubic; 6/4/c1; sqc1) when assuming that the Zn2 units act as nodes based on ToposPro analysis (Table S3†).44 The typical N–Zn–N and O–Zn–O bond angles as well as the typical Zn–O and Zn–N bond distances are listed in Table 2.
[Zn(μ2-muconate)(μ2-bpe)]·(H2O)2(DEF) (6-bpe).
Two carboxylates from two different muconates and two pyridyl nitrogen atoms from two different bpe ligands coordinate to a ZnII ion with a distorted tetrahedral geometry, forming a 3-D framework (Fig. 5). The 3-D frameworks are four-fold interpenetrated (Fig. 5c). The asymmetric unit contains a ZnII ion, muconate, and bpe. Water and DEF molecules are present in the voids, but they could not be refined due to the disordering problem. The total number of electrons for the disordered solvent molecules can be counted using PLATON SQUEEZE. The estimated number of solvent molecules was calculated from elemental analysis, which can support the presence of specific water and DEF guests in the pore. The solvent-free 3-D framework indicates a void volume of 42.7% (1108.4 Å3/2592.9 Å3), which is based on PLATON analysis.45 The structure is similar to 3-bpe, indicating a 4-connected uninodal net with a Schläfli symbol of 66 when assuming that the ZnII ions act as nodes and without any simplification based on ToposPro analysis (Table S4†).44 The N–Zn–N and O–Zn–O bond angles as well as the Zn–O and Zn–N bond distances are listed in Table 2.
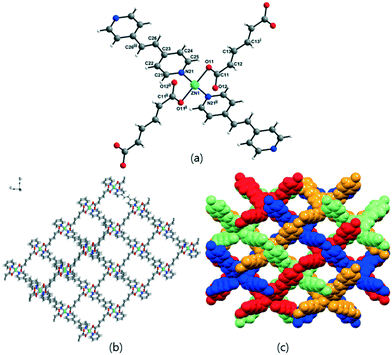 |
| Fig. 5 (a) Tetrahedral geometry around the ZnII ion of 6-bpe. Symmetry codes: i (2 − x, 1 − y, −z), ii (x, 0.5 − y, 0.5 − z), and iii (1 − x, 1 − y, 1 − z). All hydrogen atoms and disordered atoms were omitted. (b) 3-D framework along the c-axis. (c) The four-fold interpenetrated 3-D frameworks are shown in different colors. | |
Previously, six flexible α,ω-alkane (or alkene)-dicarboxylates (malonate (1-bpa), succinate (2-bpa), fumarate (3-bpa), glutarate (4-bpa), adipate (5-bpa), and muconate (6-bpa)) were employed to form 2-D or 3-D frameworks with 1,2-(4-pyridyl)ethane (bpa) ligands.33 Both dicarboxylate and bpa ligands bridge ZnII ions in the formation of the 3-D frameworks of 2-bpa, 3-bpa, 5-bpa, and 6-bpa, which are formulated as [Zn(μ2-O2CRCO2)(μ2-bpa)] with water solvates and four-fold or five-fold interpenetrated, uninodal, 4-connected dia networks (a Schläfli symbol of 66 by ToposPro analysis) as well as a tetrahedral geometry around a ZnII ion. On the other hand, 1-bpa and 4-bpa formulated as [{Zn(H2O)(O2CRCO2)}2(μ-bpa)] contain coordinated water ligands with different structures from the diamond-like structures of 2-bpa, 3-bpa, 5-bpa, and 6-bpa. The 3-D framework of 1-bpa containing octahedral ZnII ions showed 1-D channels and 2-D layers of 4-bpa with penta-coordinated ZnII ions. These results indicated that the coordination geometry and mode play important roles in structure construction.
To further investigate the effects on the construction of a variety of Zn-MOFs containing flexible α,ω-alkane (or alkene)-dicarboxylates, a different pillar, 1,2-(4-pyridyl)ethylene (bpe), was used in this system (Table 3). In contrast to the previous bpa pillar system, only fumarate and muconate provide diamond-like structures with bpe pillars. 3-D frameworks of 3-bpe and 6-bpe formulated as [Zn(μ2-O2CRCO2)(μ2-bpe)] with water and DMF (or DEF) solvates show three-fold or four-fold interpenetrated, unimodal, 4-connected dia networks.
Table 3 α,ω-Alkane (or alkene)-dicarboxylates and the related structures of Zn-MOFs
Dicarboxylate (MOF no.) |
Structure of Zn-MOF |
Schläfli symbol |
Void volume |
See ref. 32.
See ref. 39.
|
Malonatea (1-bpe) |
3D |
7-c net, 36·48·57 |
384.9 Å/1325.9 Å (29.0%) |
Succinate (2-bpe)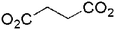 |
2D |
4-c net, 44·62 |
Nonporous |
sql/Shubnikov tetragonal plane net |
Fumarate (3-bpe)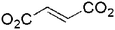 |
Three-fold 3D |
4-c net, 66 |
1258.3 Å/2558.8 Å (49.2%) squeezed |
Glutarateb (4-bpe) |
2D |
4-c net, 44·62 |
132.8 Å/886.3 Å (15.0%) |
Adipate (5-bpe) |
Two-fold 3D |
6-c net, 412·63 |
Nonporous |
pcu α-Po primitive cubic; sqc1 |
Muconate (6-bpe) |
Four-fold 3D |
4-c net, 66 |
1108.4 Å/2592.9 Å (42.7%) squeezed |
In diamond-like structures, the coordination geometry around a ZnII ion is a distorted tetrahedral. The previously reported 1-bpe32 showed a 3-D framework formulated as [{Zn(H2O)(μ2-malonate)}2(μ2-bpe)] with octahedral geometry around a ZnII ion, and 4-bpe39 formulated as [Zn(μ2-glutarate)(μ2-bpe)]·2H2O has been previously reported as a 2-D layer compound with a tetrahedral geometry around a ZnII ion from two glutarate oxygen atoms and two bpe nitrogen atoms. 2-bpe formulated as [Zn2(μ4-succinate)(μ3-succinate)(μ2-bpe)] shows a 2-D layer compound containing two asymmetric ZnII ions with penta-coordinate and octahedral geometries. 5-bpe with the [Zn(μ4-adipate)0.5(μ2-adipate)0.5(μ2-bpe)]·(H2O) formula contains two adipate types; one adipate bridges four ZnII ions and the other adipate bridges two ZnII ions with a chelating mode for each carboxylate.
Table 4 shows the relationship between structures and coordination geometry and the coordination modes of carboxylates. This relationship suggests that the 3-D diamond-like structure should have a tetrahedral geometry around ZnII ions with the monodentate coordination mode (η1:η0)(η1:η0) of carboxylates. Zn-MOFs constructed from succinate (2-bpa), fumarate (3-bpa), adipate (5-bpa), muconate (6-bpa) with bpa and fumarate (3-bpe), and muconate (6-bpe) with bpe satisfy this relationship. Both 3-D Zn-MOFs of 1-bpa and 1-bpe constructed from malonate have an octahedral geometry around ZnII ions with a special μ3-bridging mode [(η1:η1):(η1:η1):μ3] of carboxylates. This special μ3-bridging mode is possible because of malonate, for which only one methylene group (–CH2–) exists between two carboxylates. Zn-MOFs from succinate (2-bpe) and adipate (5-bpe) with bpe have an octahedral or the combination of octahedral and penta-coordinate geometries around ZnII ions and different coordination modes ((η1:η1:μ2)(η1:η1:μ2) and (η1:η1)(η1:η1) as well as (η1:η1:μ2)(η1:η1:μ2) and (η1:η1)(η2:η1:μ2)). Glutarate provides 2-D layer structures with both bpa (4-bpa) and bpe (4-bpe), but they have different geometries and coordination modes. 4-bpe has a tetrahedral geometry and monodentate mode (η1:η0)(η1:η0), while 4-bpa has a (η1:η1)(η1:η0) mode.
Table 4 Relationship between the geometry around a ZnII ion and carboxylate coordination modes
Geometry around ZnII ion |
MOF no. |
Structure of Zn-MOF |
Carboxylate coordination modes |
See ref. 33.
See ref. 39.
See ref. 32.
|
Tetrahedral |
2-bpaa, 3-bpaa, 5-bpaa, 6-bpaa, 3-bpe, 6-bpe |
3-D diamond-like structure |
μ2-Bridging with each monodentate mode (η1:η0)(η1:η0)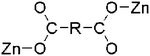 |
4-bpeb |
2-D |
Octahedral |
1-bpac, 1-bpec |
3-D |
μ3-Bridging [(η1:η1):(η1:η1):μ3]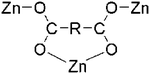 |
5-bpe |
3-D |
μ4-Bridging with each bridging mode (η1:η1:μ2)(η1:η1:μ2) and μ2-bridging with each chelating mode (η1:η1)(η1:η1)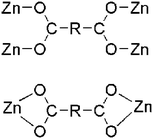 |
Octahedral and penta-coordinate |
2-bpe |
2-D |
μ4-Bridging with each bridging mode (η1:η1:μ2)(η1:η1:μ2) and μ3-bridging with each chelating and chelating/bridging modes (η1:η1)(η2:η1:μ2)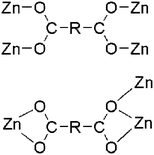 |
penta-coordinate |
4-bpaa |
2-D |
μ2-Bridging with chelating and monodentate modes (η1:η1)(η1:η0)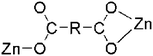 |
The results from bpa and bpe systems with the same dicarboxylate series support the same conclusion that there is a deep relationship between the structural dimensions and the coordination geometry/coordination modes of dicarboxylates. Most of all, the 3-D diamond-like structures should have a tetrahedral coordination geometry around ZnII ions and monodentate coordination modes of carboxylates are preferred.
Gas sorption analysis
The gas sorption properties of 3-bpe and 6-bpe, which have large potential void volumes, were investigated using N2, CO2, and H2 gases at suitable temperatures (Fig. 6). The Connolly surfaces of the two solvent-free MOFs are shown in Fig. S1 and S2.† Despite having the same framework structures and similar void volumes (49.2% for 3-bpevs. 42.7% for 6-bpe), the activation conditions for the removal of solvate molecules are different because they have different solvates. For 3-bpe, the as-prepared sample was simply heated at 80 °C for 2 h, resulting in a solvent-free activated sample that was suitable for gas sorption measurement. On the other hand, an elevated temperature of 180 °C (2 h) was required for complete evacuation of 6-bpe. For N2 adsorption–desorption measurement at 77 K, neither activated MOF had a meaningful uptake level. This phenomenon can be attributed to the structural change for each MOF into the nonporous state of the framework after activation. Additionally, 3-bpe did not show an appreciable uptake of H2 gas at 77 K (Fig. 6b). However, there is a significant hysteresis between the adsorption and desorption branches, which can be attributed to the small kinetic diameter of H2 (2.89 Å) compared to N2 (3.64 Å). Similarly, 6-bpe did not sorb appreciable H2 levels at 77 K. Additionally, it has hysteresis for H2 sorption as for 3-bpe. However, for CO2 sorption, 6-bpe displayed meaningful uptake levels of 86.68 cm3 g−1 (3.87 mmol g−1) at 196 K, 28.48 cm3 g−1 (1.27 mmol g−1) at 273 K, and 17.56 cm3 g−1 (0.78 mmol g−1) at 298 K.
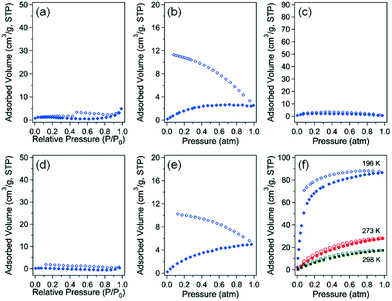 |
| Fig. 6 Gas sorption isotherms for 3-bpe (a) N2 (77 K), (b) H2 (77 K), and (c) CO2 (196 K) and for 6-bpe (d) N2 (77 K), (e) H2 (77 K), and (f) CO2. Solid and open symbols indicate adsorption and desorption isotherms, respectively. | |
The low surface coverage heat of CO2 adsorption is 25.9 kJ mol−1 based on data at 273 K and 298 K calculated by the Clausius–Clapeyron equation (Fig. 7). The initial value decreased with increasing CO2 uptake, but it gradually increased up to 26.6 kJ mol−1. The preadsorbed CO2 molecules may have stronger interactions with the newly entering CO2 molecules. These results reveal that 6-bpe selectively sorbed CO2 over N2 and H2.
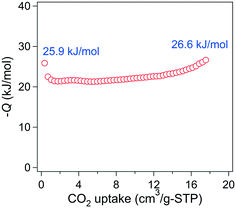 |
| Fig. 7 Heats of adsorption for 6-bpe as a function of the CO2 uptake. | |
It is noteworthy that two-fold interpenetrated 3-D 5-bpe did not give a porous structure compared with the previous four-fold interpenetrated 3-D 5-bpa that had 66dia-topology. 5-bpa and 6-bpa exhibited CO2 adsorption capacities of 79.9 cm3 g−1 (3.56 mmol g−1) and 81.5 cm3 g−1 (3.60 mmol g−1) at 196 K, respectively.33 Despite the similar levels of CO2 uptake levels at 196 K, the most prominent difference in the CO2 adsorption between 6-bpe and 6-bpa is the isotherm shape. In the low pressure region, 6-bpe did not show an S-shape adsorption isotherm compared with 6-bpa. This difference can be due to the lower flexibility of bpe compared to the bpa pillar. As a result, the framework change from the activated form with increasing CO2 pressure is not as large in 6-bpe as in 6-bpa. These results indicate that both α,ω-alkane (or alkene)-dicarboxylate bridging ligands and the pillar rigidity may be critical factors that determine the framework structure and gas sorption properties of this MOF system.
Because very weak hysteretic behavior for 3-bpe was observed in N2 adsorption–desorption isotherms (Fig. 6a), we additionally checked the high-pressure volumetric adsorption capacity of 3-bpe up to 30 atm using CO2 at 273 K (Fig. S3†). Although the final uptake level at 30 atm was not high (3.79 wt%), the adsorption isotherm displayed an interesting stepped isotherm that depended on the supplied CO2 pressure. Two prominent steps appeared at approximately 5.3 atm and 10.6 atm. These steps imply that there are changes in the activated form of the framework with increasing CO2 pressure. For 6-bpe, the uptake at 30 atm and 273 K was 9.44 wt%, and this value is much larger than for 3-bpe. Unlike for 3-bpe, the adsorption isotherm did not show any steps. These results suggest that the activated framework of 6-bpe is more rigid than that of 3-bpe.
Thermogravimetric analysis
To study the thermal stabilities of 2-bpe, 3-bpe, 5-bpe, and 6-bpe, TGAs were performed. For 2-bpe (Fig. S4†), the 12% weight loss in the range of 99 to 345 °C may be attributed to the removal of four and a half solvated water molecules (calcd. 12.3%). The TGA curve of 3-bpe (Fig. S5†) showed a weight loss of 18% in the temperature range of 81 to 143 °C, corresponding to the removal of half the solvated water molecules and a DMF molecule (calcd. 18.5%). The TGA curve for 5-bpe (Fig. S6†) showed a weight loss of 4% from 240 to 298 °C, which is attributed to the loss of one water molecule (calcd. 4.4%). The TGA profile of 6-bpe (Fig. S7†) indicated a loss of two water molecules and one DEF molecule between 120 and 280 °C, as the observed weight loss (25.8%) was identical to the calculated value (calcd. 26.1%).
Encapsulation of iodine
Molecular iodine is a good model compound for MOF encapsulation application due to its volatile nature. Therefore, the efficient capture of a volatile iodine molecule is a good measure for stabilizing volatile guests inside MOF channels and has potential applicability for the removal of toxic iodine and other harmful species.46–48 MOFs are an ideal platform for capturing iodine in either solution or the gas phase. For this reason, iodine encapsulation for 3-bpe and 6-bpe was tested in cyclohexane. Each MOF sample of as-prepared 3-bpe and 6-bpe (20 mg) was immersed in 1.0 mM iodine solution at room temperature for 48 h with gentle shaking. The final uptake level for 3-bpe (54%, 0.12 mmol of I2 per mmol of 3-bpe) was lower than that for 6-bpe (61%, 0.16 mmol of I2 per mmol of 6-bpe), as depicted in Fig. 8. The initial uptake rate for 3-bpe was faster than that for 6-bpe. The UV/vis raw spectra for iodine encapsulation measurements are shown in Fig. S8.† The color of crystal images of as-prepared 6-bpe and 6-bpe_I2 is shown in Fig. 9. The iodine-captured framework of 3-bpe and 6-bpe was structurally characterized by X-ray crystallography (Table 1).
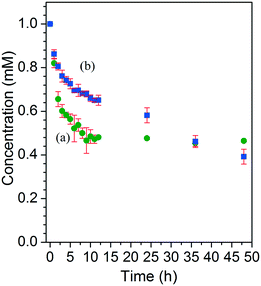 |
| Fig. 8 Time courses of iodine encapsulation by as-prepared 3-bpe (a) and 6-bpe (b). | |
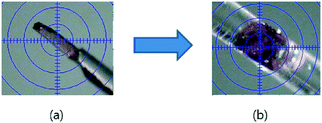 |
| Fig. 9 Images of the color-changed crystals of as-prepared 6-bpe (a, yellow on the fiber) and I2-containing 6-bpe_I2 (b, purple in a Lindemann capillary). | |
Both single crystals of 3-bpe_I2 and 6-bpe_I2 were placed into Lindemann capillaries with the mother liquor for data collection. Iodine molecules in 3-bpe_I2 could not be found or refined due to the high disordering; as a result, SQUEEZE/PLATON was used in structural refinement in the X-ray experiment. Fortunately, iodine molecules of 6-bpe_I2 were refined, and the occupancies of I2 were fixed to obtain the best fit with the largest residual peak. However, the actual occupancies should be larger than the fixed occupancies. The I–I distance is 3.297(1) Å. This distance is somewhat longer than the previous values (2.7–2.8 Å) reported in the literature.47,48 The iodine molecules may occupy the pore through weak non-classical H-bonding interactions (Fig. 10).49 The closest distances from the muconate C–H to the I atom and from the bpe C–H to the I atom are 4.030(1) Å and 4.429(1) Å, respectively, and these relatively long distances show weak non-classical H-bonding interactions between the framework and iodine molecules.
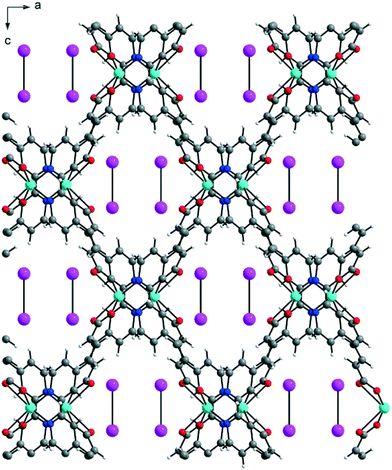 |
| Fig. 10 Structure of 6-bpe_I2 along the b-axis. Purple spheres represent iodine atoms. | |
Conclusions
A series of new Zn-MOFs containing a flexible α,ω-alkane (or alkene)-dicarboxylate and a relatively rigid bpe bridging linker were prepared and structurally characterized by X-ray crystallography. These include the following: succinate (2-bpe), fumarate (3-bpe), adipate (5-bpe), and muconate (6-bpe). 3-bpe and 6-bpe, which had a 3-D diamond-like framework structure and showed potential void volumes. However, only 6-bpe displayed a meaningful sorption behavior for CO2 at 196 K. 6-bpe selectively sorbed CO2 over N2 and H2 at a low temperature. 5-bpe also showed a 3-D framework structure that was different from the diamond-like structure. 5-bpe contained a dimensionally similar adipate linker compared with muconate in 6-bpe and sorbed none of CO2, N2 or H2. 2-bpe formed a 2-D framework. Both 3-bpe and 6-bpe showed a good encapsulating ability for volatile molecular iodine in cyclohexane. The iodine molecules captured inside 6-bpe channels were structurally confirmed.
Acknowledgements
This work was supported by the Basic Science Research Program of the National Research Foundation of Korea (NRF) funded by the Ministry of Science, ICT & Future Planning (2015R1C1A2A01053025) and by the Ministry of Education, Science and Technology (2013R1A1A2006914 and 2015R1D1A1A01058136). Financial support from Kwangwoon University in the year 2016 is also gratefully acknowledged.
Notes and references
- O. M. Yaghi, M. O'Keeffe, N. W. Ockwig, H. K. Chae, M. Eddaoudi and J. Kim, Nature, 2003, 423, 705 CrossRef CAS PubMed.
- J. Kim, B. Chen, T. M. Reineke, H. Li, M. Eddaoudi, D. B. Moler, M. O'Keeffe and O. M. Yaghi, J. Am. Chem. Soc., 2001, 123, 8239 CrossRef CAS PubMed.
- M. L. Foo, R. Matsuda and S. Kitagawa, Chem. Mater., 2014, 26, 310 CrossRef CAS.
- M. Eddaoudi, D. F. Sava, J. F. Eubank, K. Adila and V. Guillerm, Chem. Soc. Rev., 2015, 44, 228 RSC.
- Y. He, W. Zhou, R. Krishnad and B. Chen, Chem. Commun., 2012, 48, 11813 RSC.
- A. Cadiau, K. Adil, P. M. Bhatt, Y. Belmabkhout and M. Eddaoudi, Science, 2016, 353, 137 CrossRef CAS PubMed.
- X. Cui, K. Chen, H. Xing, Q. Yang, R. Krishna, Z. Bao, H. Wu, W. Zhou, X. Dong, Y. Han, B. Li, Q. Ren, M. J. Zaworotko and B. Chen, Science, 2016, 353, 141 CrossRef CAS PubMed.
- J.-R. Li, Y. Ma, M. C. McCarthy, J. Sculley, J. Yu, H.-K. Jeong, P. B. Balbuena and H.-C. Zhou, Coord. Chem. Rev., 2011, 255, 1791 CrossRef CAS.
- K. Sumida, D. L. Rogow, J. A. Mason, T. M. McDonald, E. D. Bloch, Z. R. Herm, T. H. Bae and J. R. Long, Chem. Rev., 2012, 112, 724 CrossRef CAS PubMed.
- Y.-S. Bae and R. Q. Snurr, Angew. Chem., Int. Ed., 2011, 50, 11586 CrossRef CAS PubMed.
- U. Eberle, M. Felderhoff and F. Schüth, Angew. Chem., Int. Ed., 2009, 48, 6608 CrossRef CAS PubMed.
- A. M. Seayad and D. M. Antonelli, Adv. Mater., 2004, 16, 765 CrossRef CAS.
- M. P. Suh, H. J. Park, T. K. Prasad and D.-W. Lim, Chem. Rev., 2012, 112, 782 CrossRef CAS PubMed.
- D. Farrusseng, S. Aguado and C. Pinel, Angew. Chem., Int. Ed., 2009, 48, 7502 CrossRef CAS PubMed.
- A. Dhakshinamoorthy, M. Opanasenko, J. Čejka and H. Garcia, Catal. Sci. Technol., 2013, 3, 2509 CAS.
- J.-M. Gu, W.-S. Kim and S. Huh, Dalton Trans., 2011, 40, 10826 RSC.
- P. Horcajada, R. Gref, T. Baati, P. K. Allan, G. Maurin, P. Couvreur, G. Férey, R. E. Morris and C. Serre, Chem. Rev., 2012, 112, 1232 CrossRef CAS PubMed.
- D. Cunha, M. B. Yahia, S. Hall, S. R. Miller, H. Chevreau, E. Elkaïm, G. Maurin, P. Horcajada and C. Serre, Chem. Mater., 2013, 25, 2767 CrossRef CAS.
- B. Bhattacharya and D. Ghoshal, CrystEngComm, 2015, 17, 8388 RSC.
- M. G. Cowan, R. G. Miller, P. D. Southon, J. R. Price, O. Yazaydin, J. R. Lane, C. J. Kepert and S. Brooker, Inorg. Chem., 2014, 53, 12076 CrossRef CAS PubMed.
- W. Lu, J. P. Sculley, D. Yuan, R. Krishna, Z. Wei and H.-C. Zhou, Angew. Chem., Int. Ed., 2012, 51, 7480 CrossRef CAS PubMed.
- J.-M. Gu, T.-H. Kwon, J.-H. Park and S. Huh, Dalton Trans., 2010, 39, 5608 RSC.
- S. H. Chae, H.-C. Kim, Y. S. Lee, S. Huh, S.-J. Kim, Y. Kim and S. J. Lee, Cryst. Growth Des., 2015, 15, 268 CAS.
- R. Haldar, M. Inukai, S. Horike, K. Uemura, S. Kitagawa and T. K. Maji, Inorg. Chem., 2016, 55, 4166 CrossRef CAS PubMed.
- K. Fukuhara, S.-I. Noro, K. Sugimoto, T. Akutagawa, K. Kubo and T. Nakamura, Inorg. Chem., 2013, 52, 4229 CrossRef CAS PubMed.
- L. Sarkisov, R. L. Martin, M. Haranczyk and B. Smit, J. Am. Chem. Soc., 2014, 136, 2228 CrossRef CAS PubMed.
- Y. Kim and S. Huh, CrystEngComm, 2016, 18, 3524 RSC.
- A. G. Slater and A. I. Cooper, Science, 2015, 348, aaa8075 CrossRef PubMed.
- M. O'Keeffe, M. A. Peskov, S. J. Ramsden and O. M. Yaghi, Acc. Chem. Res., 2008, 41, 1782 CrossRef PubMed.
- S. Natarajan and P. Mahata, Chem. Soc. Rev., 2009, 38, 2304 RSC.
- I. H. Hwang, J. M. Bae, W.-S. Kim, Y. D. Jo, C. Kim, Y. Kim, S.-J. Kim and S. Huh, Dalton Trans., 2012, 41, 12759 RSC.
- M. Y. Hyun, I. H. Hwang, M. M. Lee, H. Kim, K. B. Kim, C. Kim, H.-Y. Kim, Y. Kim and S.-J. Kim, Polyhedron, 2013, 53, 166 CrossRef CAS.
- I. H. Hwang, H.-Y. Kim, M. M. Lee, Y. J. Na, J. H. Kim, H.-C. Kim, C. Kim, S. Huh, Y. Kim and S.-J. Kim, Cryst. Growth Des., 2013, 13, 4815 CAS.
- B. Bozbiyik, J. Lannoeye, D. E. De Vos, G. V. Baron and J. F. M. Denayer, Phys. Chem. Chem. Phys., 2016, 18, 3294 RSC.
- T. K. Kim, K. J. Lee, M. Choi, N. Park, D. Moon and H. R. Moon, New J. Chem., 2013, 37, 4130 RSC; D. Sun, M.-Z. Xu, S.-S. Liu, S. Yuan, H.-F. Lu, S.-Y. Feng and D.-F. Sun, Dalton Trans., 2013, 42, 12324 RSC; L. Fan, X. Zhang, D. Li, D. Sun, W. Zhang and J. Dou, CrystEngComm, 2013, 15, 349 RSC; D. Sun, N. Zhang, R.-B. Huang and L.-S. Zheng, Cryst. Growth Des., 2010, 10, 3699 Search PubMed.
- B. Ugale and C. M. Nagaraja, RSC Adv., 2016, 6, 28854 RSC.
- X. Yin, J. Tao, R.-B. Huang and L.-S. Zheng, Main Group Met. Chem., 2002, 25, 705 CAS.
- J.-Y. Xu, E. J. Hurtado, E. B. Lobkovsky and B. Chen, Acta Crystallogr., Sect. E: Struct. Rep. Online, 2007, 63, m2205 CAS.
- I. H. Hwang, J. M. Bae, Y.-K. Hwang, H.-Y. Kim, C. Kim, S. Huh, S.-J. Kim and Y. Kim, Dalton Trans., 2013, 42, 15645 RSC.
-
Bruker, SHELXTL/PC. Version 6.12 for Windows XP, Bruker AXS Inc., Madison, Wisconsin, USA, 2001 Search PubMed.
-
K. Nakamoto, Infrared and Raman Spectra of Inorganic and Coordination Compounds, John Wiley & Sons, New York, 1986 Search PubMed.
- J. Tao, M.-L. Tong and X.-M. Chen, J. Chem. Soc., Dalton Trans., 2000, 3669 RSC.
- W. Chen, J.-Y. Wang, C. Chen, Q. Yue, H.-M. Yuan, J.-S. Chen and S.-N. Wang, Inorg. Chem., 2003, 42, 944 CrossRef CAS PubMed.
- V. A. Blatov, A. P. Shevchenko and D. M. Proserpio, Cryst. Growth Des., 2014, 14, 3576 CAS ; http://topospro.com; Website access date is 09.24.2016.
-
A. L. Spek, PLATON-A multipurpose Crystallographic Tool, Utrecht University, Utrecht, The Netherlands, 2004 Search PubMed.
- P.-S. Huang, C.-H. Kuo, C.-C. Hsieh and Y.-C. Horng, Chem. Commun., 2012, 48, 3227 RSC.
- D. F. Sava, M. A. Rodriguez, K. W. Chapman, P. J. Chupas, J. A. Greathouse, P. S. Crozier and T. M. Nenoff, J. Am. Chem. Soc., 2011, 133, 12398 CrossRef CAS PubMed.
- D. F. Sava, K. W. Chapman, M. A. Rodriguez, J. A. Greathouse, P. S. Crozier, H. Zhao, P. J. Chupas and T. M. Nenoff, Chem. Mater., 2013, 25, 2591 CrossRef CAS; S. Yuan, Y.-K. Deng and D. Sun, Chem. – Eur. J., 2014, 20, 10093 CrossRef PubMed; S. Yuan, Y.-K. Deng, W.-M. Xuan, X.-P. Wang, S.-N. Wang, J.-M. Dou and D. Sun, CrystEngComm, 2014, 16, 3829 RSC.
- H. Kwak, S. H. Lee, S. H. Kim, Y. M. Lee, E. Y. Lee, B. K. Park, E. Y. Kim, C. Kim, S.-J. Kim and Y. Kim, Eur. J. Inorg. Chem., 2008, 408 CrossRef CAS.
Footnote |
† Electronic supplementary information (ESI) available: Connolly surfaces. High-pressure CO2 adsorption isotherms. TGA profiles for 2-bpe, 3-bpe, 5-bpe, and 6-bpe. CCDC 1438600–1438602, 1438604, 1481289 and 1481290. For ESI and crystallographic data in CIF or other electronic format see DOI: 10.1039/c6ce02122k |
|
This journal is © The Royal Society of Chemistry 2017 |