DOI:
10.1039/C6CC09934C
(Communication)
Chem. Commun., 2017,
53, 2182-2185
The polyketide backbone of thiolactomycin is assembled by an unusual iterative polyketide synthase†
Received
14th December 2016
, Accepted 17th January 2017
First published on 26th January 2017
Abstract
Following the in vivo investigation of thiotetronate assembly in Lentzea sp. and in S. thiolactonus NRRL 15439 (Havemann et al., Chem. Commun., 2017, DOI: 10.1039/c6cc09933e), the minimal set of genes required for thiolactomycin production was determined through heterologous expression and the mechanism for polyketide assembly was established in vitro through incubation of recombinant TlmB with its substrates in the presence of either nonhydrolysable or hydrolysable chemical probes. The results presented here constitute unequivocal evidence of enzymatic processing by an unusual iterative polyketide synthase.
In 2014, the World Health Organisation (WHO) declared antimicrobial resistance a global health crisis, with a ‘post-antibiotic era’ looming unless critical measures are taken.2 While rigorous changes in policy are required, there is also an urgent need for effective new antibiotics, and in particular, for compounds that act on biological targets that are underexploited, highly conserved, essential for survival, and unique to bacteria.3 Thiolactomycin (TLM, 1, Fig. 1C), a thiotetronate antibiotic first discovered in 1982 from a soil Nocardia strain (ATCC 31319,4 since re-named Lentzea sp.) reversibly inhibits the β-ketoacyl-acyl carrier protein synthase (KAS) enzymes of type II (dissociated) fatty acid synthase (FAS), an essential metabolic pathway for bacterial cell viability.5 By binding preferentially to the acyl–enzyme intermediate of the KAS enzymes,6 TLM and other thiotetronates, such as Tü 3010,7 confer broad antimicrobial activity against both Gram-positive and Gram-negative bacteria and in murine models of infection.8 Additionally, thiotetronates show promise as a Mycobacterium tuberculosis therapeutic9 as well as anti-malarial and anti-trypanosomal activity through the inhibition of apicoplast type II FAS.10 The broad spectrum antimicrobial activity displayed by TLM, its effectiveness in murine models of infection, and its favourable physical properties, have all established a rationale for the development of TLM derivatives. To date, the more ramified thiotetronate, Tü 3010, has been reported to be 15-fold more effective as an antibacterial in vivo, and various recent synthetic modifications to the TLM C3- and C5-methyl groups have led to increased activity against Plasmodium falciparum, Francisella tularensis, methicillin-sensitive Staphyloccus aureus (MSSA), and methicillin-resistant Staphyloccus aureus (MRSA).8b,11 The C5-chiral centre of thiotetronates highly complicates analogue synthesis,12 making this class of natural products an attractive target for genetic engineering. Early experiments with isotopically-labelled precursors indicated that this class of molecules were likely of polyketide origin.13 While the genetic cluster was not initially identified, it was predicted that 1 would be possibly assembled by a multimodular type I polyketide synthase (PKS) as various levels of β-keto reduction would be expected within the TLM polyketide scaffold. In 2015 the biosynthetic gene clusters for TLM (tlm) and Tü 3010 (tue, stu, ssu) were identified by comparative genetic analysis by our group14 and others.15In silico analysis of the predicted tlm biosynthetic gene cluster within the TLM producing strain Lentzea sp. revealed only four open reading frames (ORFs): a gene encoding a copy of the known TLM intracellular target KASI/II enzyme, tlmF; a gene encoding an unexpected cytochrome P450, tlmD1, which we have shown to be essential for TLM biosynthesis through in-frame genetic mutation;14tlmA, encoding a PKS-protein housing a KSQ domain,16 and an acyl carrier protein (ACP) domain, therefore likely acting as the initiation module; and tlmB, encoding a novel multienzyme containing a single PKS extension module juxtaposed at its C-terminus with several NRPS domains. Bioinformatic analysis of this peculiar biosynthetic cluster led to the prediction that the TLM tetraketide backbone would be assembled by the ORFs tlmA and tlmB. TlmA was proposed to catalyse the attachment of a malonyl group to the ACP and its subsequent decarboxylation providing the acetate starter unit for TLM, likely borrowing its required acyltransferase (AT) domain from the malonyl-CoA:ACP acyl-transferase of fatty acid biosynthesis (MCAT), as presented earlier in actinorhodin17 and FK228 biosyntheses.18 The predicted domain order within TlmB from the N-terminus is ketosynthase (KS), acyltransferase (AT), dehydratase (DH), ketoreductase (KR), acyl-carrier protein (ACP) domains, followed by the NRPS domains: cyclisation (Cy), adenylation (A) and peptidyl carrier protein (PCP). Therefore TlmB was envisaged to act as an iterative type I PKS by recruiting three successive propionate units and catalyzing three cycles of chain elongation. In-frame gene mutation and complementation studies on tlmA within the ATCC 31319 tlm cluster have revealed that tlmA is essential for thiotetronate biosynthesis, strengthening our proposed mechanism for thiotetronate polyketide assembly unexpectedly involving an iterative, rather than modular, system.14 An encouraging precedent for such an iterative assembly-line is the recently-uncovered PKS for the 14-macrocyclic polyketide galbonolide.19 Validation of this mechanism requires isolation and identification of the intermediate species. While this has historically been a significant obstacle due to the covalent attachment of all intermediates to polyketide synthase multienzymes throughout chain assembly, a recently developed chemical strategy has provided a useful way to sample and identify PKS intermediates from both modular20 and iterative systems.21 This strategy employs nonhydrolysable synthetic mimics of the natural PKS extender units recruited for polyketide formation. These small molecules act as competitive substrates for the natural ACP-bound extender units, carrying out decarboxylative condensation with the KS-tethered polyketide: by decoying and removing the growing chain from the PKS, the product from each round of chain extension can be isolated and analysed. As reported by Havemann et al. in the accompanying paper,1in vivo feeding of nonhydrolysable synthetic mimics to the TLM-producing strain Lentzea sp. resulted in the capture and identification of putative thiolactomycin polyketide intermediates1 including di-, tri- and tetraketide species with the expected states of β-keto-reduction.1 However, this work alone cannot conclusively demonstrate that only TlmA and TlmB are sufficient for polyketide assembly. To do this, the minimal set of genes required for TLM production was first determined through heterologous expression of the gene cluster in several heterologous Streptomyces hosts, and then the mechanism for TLM polyketide assembly was enzymatically reconstituted in vitro. The tlm cluster was cloned and transplanted into two genetically well-defined Streptomyces heterologous host strains. The 13.7 kbp tlm cluster was PCR amplified as a fragment containing terminal regions identical to the NdeI- and EcoRI-digested ends of pIB139. This fragment was then inserted into digested pIB139 through Gibson assembly, transformed into Escherichia coli DH10B, and apramycin-resistant pTLM containing colonies were confirmed through PCR analysis and Sanger sequencing. pTLM (Fig. 1S, ESI†) was introduced into the Streptomyces coelicolor M1154 and Streptomyces lividans TK24 genomes by conjugation through the triply methylation-deficient E. coli strain ET12567/pUZ8002. The resulting transformed strains M1154::pTlm and TK24::pTlm were cultured and the extracts analysed by LCMS. As shown in Fig. 1, the recombinant strains gained the ability to produce TLM at comparable/higher levels than those found in Lentzea sp. Having independently identified a tlm cluster within Salinispora pacifica CNS863, Tang et al. have also been successful in demonstrating heterologous production of TLM in the recombinant host M1152/pMXT13.15 TLM production in heterologous Streptomyces hosts has confirmed that only TlmA and TlmB are required for assembly of the thiolactomycin polyketide chain, and that the sulfur insertion machinery is borrowed from primary metabolism.
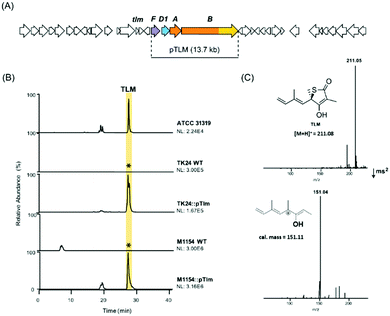 |
| Fig. 1 (A) Organisation of the proposed tlm cluster in Lentzea sp. and the limits of the fragment cloned into pTLM. (B) LC-MS analysis of the selective ion trace of thiolactomycin ([M + H]+ = 211.1 m/z) within WT and recombinant heterologous strains. The asterisk means not detected. (C) Confirmation of TLM production by MS-MS analysis.14 | |
To attempt the reconstitution of polyketide chain assembly in vitro, we first undertook recombinant expression of the giant PKS-NRPS multienzyme, TlmB. The N-terminally His6-tagged 316.8 kDa recombinant protein was expressed in E. coli BL21(DE3) and purified by selective ammonium sulfate precipitation (Fig. 2A). Matrix-assisted laser desorption/ionisation (MALDI) mass fingerprinting of recombinant TlmB, following tryptic digest, was used to confirm the identity of the expressed protein (Fig. 2S, ESI†). To ensure that the ACP domain of TlmB was in the active holo- form through addition of a 4′-phosphopantetheine (4′PP) arm, the broad-specificity 4′PP transferase Sfp from B. subtilis22 was co-expressed with recombinant TlmB. MALDI-TOF analysis to determine whether co-expression had been successful was inconclusive (Fig. 3S, ESI†), so CoASH and heterologously purified Sfp were included in TlmB enzymatic assays to ensure complete in vitro conversion of apo-ACP to holo. Based on the assumption that TlmA is the initiating enzyme providing an acetate starter unit for TlmB via malonate decarboxylation, we replaced TlmA in these assays with acetyl-CoA (2). To reconstitute thiolactomycin polyketide assembly and probe the proposed iterative mechanism of TlmB, the same chain termination probes utilised by Havemann and co-workers1in vivo were employed in vitro. Prior to incubation with recombinant TlmB, the malonate and fluoromalonate esters 4–5 were hydrolysed to the corresponding carboxylates 6–7 through incubation with pig liver esterase (PLE)20a (Fig. 2B). Purified TlmB, co-expressed with Sfp, was incubated with each probe in the presence of assay substrates acetyl-CoA (2) and (2RS)-methylmalonyl-CoA (3), as well as DTT and NADPH. The enzyme assay mixtures were quenched and extracted with ethyl acetate. High-resolution mass-spectrometry (HRMS) analyses of the organic extracts showed the presence of putative enoyl-diketide intermediates from both malonyl- and fluoromalonyl terminator assays (8–9, Fig. 2B and Fig. 4S, 10S, ESI†), indicating that acetyl-CoA could indeed replace TlmA to initiate chain extension through loading of acetyl groups onto recombinant TlmB. Additionally, for each terminator species, β-keto and β-hydroxy diketide intermediates were also detected (Table 4S and Fig. 4S, 10S, ESI†), revealing all the reductive enzyme-catalysed steps employed during the first round of chain extension. Furthermore, dienoyl triketide intermediates from the second round of TlmB chain extension (10–11) were also detected, albeit in minor amounts, from both malonyl- and fluoromalonyl terminator assays, as well as triketide β-keto and β-hydroxy species (Fig. 6S and 12S, ESI†). Finally, from the third and final round of TlmB chain extension, we were able to detect off-loaded dienoyl tetraketides (e.g.12) in organic extracts (Fig. 1B, C and Fig. 8S, ESI†). None of these putative intermediates, each characterised by HR-MSn analyses, were found in controls containing boiled TlmB; and the intermediates were virtually identical to those identified from in vivo feeding studies,1 supporting substrate processing by TlmB as an iterative synthase. To further confirm substrate processing by TlmB to dienoyl tetraketides, the hydrolysable N-acetylcysteamine triketide 14 was synthesised according to Scheme 1S (ESI†) and utilised to prime TlmB. Addition of chain termination probes 6–7 to the newly primed TlmB in the presence of the natural extender unit 3 led to the formation of dienoyl tetraketides 12–13 (Fig. 2D and Fig. 9S, 13S, ESI†). No further elaborated polyketide species (e.g. reduced tetraketides) were detected. These results taken together constitute unequivocal evidence of polyketide chain building and processing by an iterative enzyme in thiotetronate bio-assembly. The discovery that the thiotetronate polyketide backbone is assembled by an iterative PKS is particularly interesting given that a different level of β-keto reduction is required during the final cycle. Further, in the case of the more elaborate Tü 3010 and thiotetronomycin structures, both methylmalonyl-CoA and ethylmalonyl-CoA extension units are selected and activated by a single AT domain. The successful reconstitution of this system in vitro makes this recombinant enzyme an attractive system for further structural studies. High resolution structural investigation of TlmB could provide insights into the poorly-understood23 factors that control reduction within iterative PKSs, and pave the way to future engineering of unnatural thiotetronates.
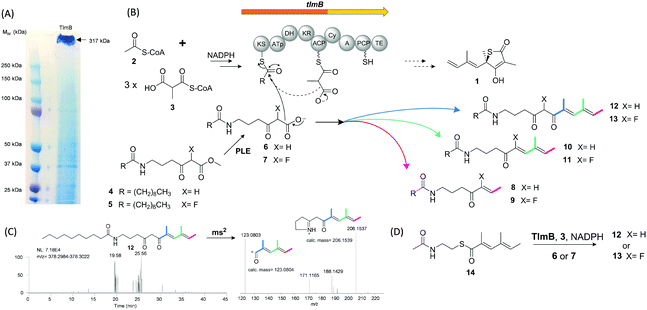 |
| Fig. 2 (A) SDS-PAGE analysis of recombinant TlmB. (B) Chain termination probes 6 and 7, generated from PLE-catalysed hydrolysis of 4 and 5, compete with ACP-bound methylmalonate in vitro to off-load recombinant TlmB-bound intermediates (representative structures shown, full overview of captured species is given in Table 4S, ESI†). (C) HR-MSn analysis of the putative dienoyl tetraketide 12 captured by probe 6 from recombinant TlmB. (D) Incubation of recombinant TlmB with the synthetic SNAc triketide 14 in the presence of probe 6 or 7 results in the formation of species 12 or 13 (ESI†). | |
Complete in vitro reconstitution of the PKS enzymology within TLM biosynthesis has confirmed an unexpectedly iterative method of assembly. This work has now paved the way for the investigation of post-PKS mechanisms leading to sulfur-insertion and thiolactone formation.
We gratefully acknowledge support from the Herchel Smith Chair of Biochemistry Fund and PGS-D3 funding from the Natural Sciences and Engineering Research Council of Canada (NSERC) (grants to M. E. Y.); support from BBSRC (project grant BB/J007250/1 to M. T.) and EPSRC (DTA PhD studentship to R. J.); and Dr Cleidiane Zampronio (School of Life Sciences, Warwick) for assistance with LC-HRMSn analyses performed on an Orbitrap Fusion instrument.
Notes and references
- J. Havemann, M. E. Yurkovich, R. Jenkins, S. Harringer, W. Tao, S. Wen, Y. Sun, P. F. Leadlay and M. Tosin, Chem. Commun., 2017 10.1039/c6cc09933e.
-
(a) J. O'Neil, Rev. Antimicrob. Res., 2016, 1, 1–76 Search PubMed;
(b) E. D. Brown and G. D. Wright, Nature, 2016, 529, 336–343 CrossRef CAS PubMed;
(c) O. Genilloud, Antonie van Leeuwenhoek, 2014, 106, 173–188 CrossRef CAS PubMed.
- J. Campbell and J. E. Cronan, Annu. Rev. Microbiol., 2001, 55, 305–332 CrossRef CAS PubMed.
-
(a) H. Oishi, T. Noto, H. Sasaki, K. Suzuki, T. Hyashi, O. Hiroshi, K. Ando and M. J. Sawada, J. Antibiot., 1982, 35, 771–777 CrossRef;
(b) T. Noto, S. Miyakawa, H. Oishi, H. Endo and H. Okazaki, J. Antibiot., 1982, 35, 401–410 CrossRef CAS PubMed.
-
(a) S. Jackowski, C. M. Murphy, J. E. Cronan and C. O. Rock, J. Biol. Chem., 1989, 264, 7624–7629 CAS;
(b) A. C. Price, K. H. Choi, R. J. Heath, Z. Li, S. W. White and C. O. Rock, J. Biol. Chem., 2001, 276, 6551–6559 CrossRef CAS PubMed;
(c) S. Kodali, A. Galgoci, K. Young, R. Painter, L. L. Silver, K. B. Herath, S. B. Singh, D. Cully, J. F. Barrett, D. Schmatz and J. Wang, J. Biol. Chem., 2005, 280, 1669–1677 CrossRef CAS PubMed.
- S. R. Luckner, C. A. Machutta, P. J. Tonge and C. Kisker, Structure, 2009, 17, 1004–1013 CrossRef CAS PubMed.
- C. Rapp, G. Jung, C. Isselhorst-Scharr and H. Zähner, Liebigs Ann. Chem., 1988, 1988, 1043–1047 CrossRef.
-
(a) S. Miyakawa, K. Suzuki, T. Noto, Y. Harada and H. Okazaki, J. Antibiot., 1982, 35, 411–419 CrossRef CAS PubMed;
(b) G. R. Bommineni, K. Kapilashrami, J. E. Cummings, Y. Lu, S. E. Knudson, C. Gu, S. G. Walker, R. A. Slayden and P. J. Tonge, J. Med. Chem., 2016, 59, 5377–5399 CrossRef CAS PubMed.
-
(a) M. L. Schaeffer, G. Agnihotri, C. Volker, H. Kallender, P. J. Brennan and J. T. Lonsdale, J. Biol. Chem., 2001, 276, 47029–47037 CrossRef CAS PubMed;
(b) J. Schiebel, K. Kapilashrami, A. Fekete, G. Bommineni, C. Schaefer, M. Mueller, P. Tonge and C. Kisker, J. Biol. Chem., 2013, 288, 33738–33744 CrossRef PubMed;
(c) A. Bhatt, C. B. W. Stark, B. M. Harvey, A. R. Gallimore, Y. A. Demydchuk, J. B. Spencer, J. Staunton and P. F. Leadlay, Angew. Chem., Int. Ed., 2005, 44, 7075–7078 CrossRef CAS PubMed;
(d) S. Sridharan, L. Wang, A. K. Brown, L. G. Dover, L. Kremer, G. S. Besra and J. C. Sacchettini, J. Mol. Biol., 2007, 366, 469–480 CrossRef CAS PubMed.
- R. F. Waller, P. J. Keeling, R. G. Donald, B. Striepen, E. Handman, N. Lang-Unnasch, A. F. Cowman, G. S. Besra, D. S. Roos and G. I. McFadden, Proc. Natl. Acad. Sci. U. S. A., 1998, 95, 12352–12357 CrossRef CAS.
- S. Jones, J. Urch, R. Brun, J. Harwood, C. Berry and I. Gilbert, Bioorg. Med. Chem., 2004, 12, 683–692 CrossRef CAS PubMed.
-
(a) P. Kim, Y. M. Zhang, G. Shenoy, Q. A. Nguyen, H. I. Boshoff, U. H. Manjunatha, M. B. Goodwin, J. Lonsdale, A. Price, X. D. J. Miller, K. Duncan, S. W. White, C. O. Rock, C. E. Barry and C. S. Dowd, J. Med. Chem., 2006, 49, 159–171 CrossRef CAS PubMed;
(b) J. M. Mcfadden, G. L. Frehywot and C. A. Townsend, Org. Lett., 2002, 4, 3859–3862 CrossRef CAS PubMed.
- M. S. Brown, K. Akopiants, D. M. Resceck, H. A. I. McArthur, E. McCormick and K. A. Reynolds, J. Am. Chem. Soc., 2003, 125, 10166–10167 CrossRef CAS PubMed.
- W. Tao, M. E. Yurkovich, S. Wen, K. E. Lebe, M. Samborskyy, Y. Liu, A. Yang, Y. Liu, Y. Ju, Z. Deng, M. Tosin, Y. Sun and P. F. Leadlay, Chem. Sci., 2016, 7, 376–385 RSC.
- X. Tang, J. Li, N. Millan-Aguinaga, J. J. Zhang, E. C. O'Neill, J. A. Ugalde, P. R. Jensen, S. M. Mantovani and B. S. Moore, ACS Chem. Biol., 2015, 10, 2841–2849 CrossRef CAS PubMed.
- C. Bisang, P. F. Long, J. Cortes, J. Westcott, J. Crosby, A. L. Matharu, R. J. Cox, T. J. Simpson, J. Staunton and P. F. Leadlay, Nature, 1999, 401, 502–505 CrossRef CAS PubMed.
- W. P. Revill, M. J. Bibb and D. A. Hopwood, J. Bacteriol., 1995, 177, 3946–3952 CrossRef CAS PubMed.
- S. R. Wesener, V. Y. Potharla and Y. Q. Cheng, Appl. Environ. Microbiol., 2011, 77, 1501–1507 CrossRef CAS PubMed.
- C. Liu, J. Zhu, Y. Li, J. Zhang, C. Lu, H. Wang and Y. Shen, ChemBioChem, 2015, 16, 998–1007 CrossRef CAS PubMed.
-
(a) M. Tosin, L. Betancor, E. Stephens, A. W. M. Li, J. B. Spencer and P. F. Leadlay, ChemBioChem, 2010, 11, 539–546 CrossRef CAS PubMed;
(b) M. Tosin, L. Smith and P. F. Leadlay, Angew. Chem., Int. Ed., 2011, 50, 11930–11933 CrossRef CAS PubMed;
(c) M. Tosin, Y. Demydchuk, J. Parascandolo, C. Blasco Per, F. J. Leeper and P. F. Leadlay, Chem. Commun., 2011, 47, 3460–3462 RSC;
(d) E. Riva, I. Wilkening, S. Gazzola, W. M. A. Li, L. Smith, P. F. Leadlay and M. Tosin, Angew. Chem., Int. Ed., 2014, 53, 11944–11949 CrossRef CAS PubMed;
(e) I. Wilkening, S. Gazzola, E. Riva, J. S. Parascandolo, L. Song and M. Tosin, Chem. Commun., 2016, 52, 10392–10395 RSC.
-
(a) J. S. Parascandolo, J. Havemann, H. K. Potter, F. Huang, E. Riva, J. Connolly, I. Wilkening, L. Song, P. F. Leadlay and M. Tosin, Angew. Chem., Int. Ed., 2016, 55, 3463–3467 CrossRef CAS PubMed;
(b) H. Kage, E. Riva, J. S. Parascandolo, M. F. Kreutzer, M. Tosin and M. Nett, Org. Biomol. Chem., 2015, 13, 11414–11417 RSC.
- L. E. N. Quadri, P. H. Weinreb, M. Lei, M. M. Nakano, P. Zuber and C. T. Walsh, Biochemistry, 1998, 37, 1585–1595 CrossRef CAS PubMed.
- K. M. Fisch, RSC Adv., 2013, 3, 18228–18247 RSC.
Footnote |
† Electronic supplementary information (ESI) available: General methods for probe synthesis, recombinant enzyme generation and activity assays. See DOI: 10.1039/c6cc09934c |
|
This journal is © The Royal Society of Chemistry 2017 |
Click here to see how this site uses Cookies. View our privacy policy here.