DOI:
10.1039/C7RA04614F
(Paper)
RSC Adv., 2017,
7, 25964-25968
Realizing superior white LEDs with both high R9 and luminous efficacy by using dual red phosphors
Received
25th April 2017
, Accepted 9th May 2017
First published on 16th May 2017
Abstract
The luminous efficiency of radiation (LER) and colour rendering indices (CRIs) are two key technological parameters for white light-emission diodes (WLEDs), but there is always a trade-off between them, especially between LER and R9 (the 9th CRI for red colour). It is therefore necessary to find ways to solve this problem by choosing appropriate red phosphors as well as the phosphor blend. In this work, we attempted to apply both a narrow-band (K2SiF6:Mn4+,KSF) and a broad-band ((Sr,Ca)AlSiN3:Eu2+, SCASN) red phosphors together with a green phosphor (Lu3Al5O12:Ce3+, LuAG) to minimize the trade-off. For a warm white LED with the correlated colour temperature of 3000 K, the combination of a single red phosphor (either KSF or SCASN) and LuAG led to high LERs (92.4 lm W−1 for KSF and 95 lm W−1 for SCASN) but low Ra (average of the first eight CRIs) and R9 (Ra = 60.9 and R9 = −28.2 for KSF; Ra = 83.7 and R9 = 16.6 for SCASN). On the other hand, by using dual red phosphors instead of one, CRIs and even LERs are significantly increased, which is dominantly ascribed to the narrow emission band and the absence of re-absorption of green light of KSF. A superior WLED with LER = 115.4 lm W−1, Ra = 96.9 and R9 = 95.8 was attained when pumping the phosphor blend of (KSF:SCASN:LuAG = 6
:
0.75
:
5.1 in weight ratio) by a blue InGaN LED, which enables it to be used broadly for high quality solid state lighting.
A. Introduction
Currently, WLEDs are attracting great interest in both academic and industrial communities because they promise energy savings, high efficiency, robustness, environmentally friendliness and long lifetimes compared to traditional incandescent bulbs and fluorescent tubes.1,2 They are now rapidly penetrating into the lighting and display markets and contributing greatly to the greenhouse reduction and environment protection. For WLEDs, two of the most important parameters are LER and CRI, which are used to characterize the luminous efficacy and colour rendering properties, respectively.3 However, there are trade-offs between LER and CRI as they have different requirements for spectral configurations, i.e., increasing the one will compromise on another.4 Therefore, to reduce the trade-off between them is a big challenging and highly pursued topic for scientists and engineers. As to CRIs, Ra is usually used to evaluate colour rendering of a light source compared with colour reproduction under natural daylight, which is obtained by averaging the values of the first eight special colour rendering indices of unsaturated colour samples (R1–R8).5 WLEDs having Ra greater than 80 are acceptable for most lighting applications. However, only Ra is not enough to represent the colour quality, typically in the case where deep-red colour is prevalent in the hues such as lighting meat, fish, vegetables and fruits in supermarket, artwork in gallery, clothes in display windows, human skin and surgical procedures.5,6 In these lighting applications, besides Ra the R9 index, representing a saturated red colour, needs to be given to accurately render colour red.7 To bring out the richness red and provide a reasonable rating system with respect to light quality, WLEDs with both high Ra and R9 are required and getting much more attentions nowadays.8 Therefore, to greatly enhance R9 and Ra without sacrificing the luminous efficacy of WLEDs is greatly demanded for superior high light quality.
The most common way to produce WLED is to combine a yellow (Y,Gd)3(Al,Ga)5O12:Ce3+ phosphor with a blue LED chip, which is also named as one-phosphor-converted (1-pc) WLEDs. This type of WLEDs usually exhibits a high luminous efficacy, but has a low Ra (<80) and a negative R9 due to the deficiency of red component in the spectral distribution.9 To obtain high CRIs, multi-phosphors-converted WLEDs have to be considered, in which red phosphors, such as (Ca,Sr,Ba)2Si5N8:Eu2+
10–12 and (Sr,Ca)AlSiN3:Eu2+,13–15 are mixed with other green and/or yellow phosphors. Lin et al. reported a WLED showing a high Ra of 93 but a low LER of 68 lm W−1 by combining YAG:Ce and CaAlSiN3:Eu2+ with a blue LED.16 According to the photonic spectral sensitivity representing how well the human eye responds throughout the visible region under daylight conditions, human eyes have the highest sensitivity at the monochromatic wavelength of 555 nm.17 It means that the maximum of luminous efficiency can be reached in the green spectra range, and the combination of a red and a green phosphor would lead to a higher LER. Li et al. fabricated WLEDs showing a high LER (101 lm W−1), Ra (97.1) and R9 (87) by combining Ca0.78Al0.78Si1.22N2.78O0.22:Eu2+ (λem = 638 nm) with a commercial green phosphor (ZYP520G2, λem = 520 nm) phosphors.18 By employing Sr0.8Ca0.2AlSiN3:0.05Eu (λem = 617 nm) and Y3Al5−xGaxO12:Ce3+ (λem = 520 nm) phosphors, Zou et al. obtained a warm LED with Ra = 88.2 and LER = 81.4 lm W−1.19
On the other hand, (Sr,Ca)AlSiN3:Eu2+ has a broad emission band with a FWHM of 90 nm and shows a large part of spectral component longer than 650 nm, which results in high CRIs but yields low LERs due to the very weak photonic vision in the deep-red spectral region.20 We reported an exceptional case where both high LER of 101 lm W−1 and high CRIs of Ra = 96 and R9 = 95 were achieved by using a green LuAG:Ce3+ and an orangish-red Ca1−xLixAl1−xSi1+xN3:Eu2+ with a large band width of 117 nm.21 Alternatively, narrow-band red phosphors containing less spectral components longer than 650 nm are proposed to make good balances between LER and Ra as well as R9, which include K2(Si,Ti)F6:Mn4+
22–24 and (Ca,Sr)LiAl3N4:Eu2+.20,25–27 Pust et al. reported that the luminous efficacy of WLEDs was increased by 14% by using SrLiAl3N4:Eu2+ instead of CaAlSiN3:Eu2+. Ra and R9 of the prepared WLEDs were 91 and 57, respectively.20 Zhang et al. fabricated a warm WLED having high CRIs of Ra = 91.1 and R9 = 68 but a low LER of only 24.9 lm W−1, by using the phosphor blend of SrLiAl3N4:Eu2+ and YAG:Ce3+. The low efficiency was due to the re-absorption of green light from YAG:Ce3+ by SrLiAl3N4:Eu2+.28 To reduce the re-absorption, the narrow-band K2TiF6:Mn4+ (KTF) phosphor (FWHM < 40 nm), whose excitation spectrum has no overlaps with the emission band of YAG:Ce3+ or LuAG:Ce3+, was used to increase the luminous efficacy. Zhu et al. prepared WLEDs by using the phosphor blend of KTF and YAG:Ce3+ or LuAG:Ce3+, and obtained LER = 116 lm W−1, Ra = 81, R9 = 32 for “KTF + YAG” and LER = 115 lm W−1, Ra = 95 and R9 = 73 for “KTF + LuAG”.23 As seen, the luminous efficacy of such WLEDs is quite high but R9 is still low, indicating that great efforts should be made to realize both high LER and CRIs.
In this work, we proposed a strategy to overcome the trade-off between luminous efficacy and color rendering indices of warm WLEDs, by combining dual red phosphors containing the broad band (Sr,Ca)AlSiN3:Eu2+ (SCASN) and the narrow-band K2SiF6:Mn4+ (KSF) with LuAG:Ce3+. SCASN was mainly used to increase the color rendering properties whereas KSF was added to enhance the luminous efficacy. We investigated the variations of LERs, Ra and R9 with increasing the KSF/SCASN ratio, and demonstrated that both high LER and CRIs could be realized by the proposed strategy.
B. Experimental details
LuAG:Ce3+ was purchased from Nakamura-Yuji Co. (Beijing, China). It has a particle size of 6 μm. LuAG:Ce3+ exhibits an emission peak at 542 nm and an external quantum efficiency of 83% under 460 nm excitation (Fig. 1). SCASN (Sr0.8Ca0.192AlSiN3:Eu0.008) was prepared by the solid-state method at high temperature. Raw materials of Ca3N2 (99%), Si3N4 (92%), AlN (99.9%), and EuN (99%) were weighed in stoichiometric proportions and then mixed evenly. The powder mixtures were fired at 1800 °C for 2 h under 1.0 MPa N2. The SCASN phosphor was then pulverized, sieved and washed for use. The average particle size of SCASN is 12 μm. As shown in Fig. 1, SCASN shows a broad band with an emission maximum of 630 nm and a FWHM of 82 nm. The external quantum efficiency of SCASN is about 72% under 450 nm excitation. K2Si0.94F6:Mn0.06 (KSF) was synthesized by using a two-step co-precipitation method, as described in ref. 29. The synthesized phosphor shows a particle size of about 30 μm. KSF consists of several sharp emission lines with the strongest peak at 631 nm, which is due to the spin-forbidden 2Eg → 4A2 transition (Fig. 1). As seen Fig. 2, a negligible spectral overlap occurs between the excitation spectrum of KSF and emission spectrum of LuAG, whereas a large spectral overlap is seen between SCASN and LuAG.
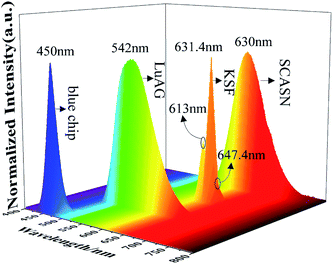 |
| Fig. 1 Normalized emission spectra of the blue LED chip, LuAG, SCASN and KSF phosphors. | |
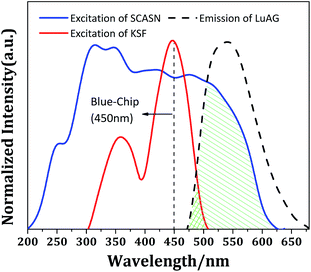 |
| Fig. 2 Excitation spectra of KSF and SCASN and emission spectrum of LuAG, showing less re-absorptions of green emission from LuAG by KSF. | |
Warm WLEDs with the correlated colour temperature of ∼3000 K were prepared by coating the blue InGaN LED chip (purchased from San'an Optoelectronics CO., LTD, China) with the phosphors blend of LuAG, SCASN and KSF. The CIE coordinates of the prepared WLEDs were x = 0.434–0.439 and y = 0.402–0.409. The epoxy resin, such as Dow Corning@OE2140 (Tokyo, Japan), was used for LED packaging. As given in Table 1, both the amounts of LuAG and SCASN were fixed while the amount of KSF was varied. The spectra of WLEDs were measured by a spectroradiometer (LHS-1000, Everfine Co., Hangzhou, China). The spatial radiation spectrum was recorded by a goniophotometer (LED626, Everfine Co., Hangzhou, China) under a DC current 60 mA and DC voltage 2.925 V at room temperature.
Table 1 Optical properties of WLEDs by using varying ratios of KSF, SCASN and LuAG phosphors
Samples |
KSF:SCASN:LuAG |
Ra |
R9 |
LER (lm W−1) |
A |
1 : 0 : 5.1 |
60.9 |
−28.2 |
92.4 |
B |
0 : 0.72 : 5.1 |
83.7 |
16.6 |
95.0 |
C |
1 : 0.72 : 5.1 |
87.3 |
32.4 |
105.2 |
D |
2 : 0.72 : 5.1 |
90.2 |
46 |
112.2 |
E |
3 : 0.72 : 5.1 |
92.9 |
59.3 |
115.2 |
F |
4 : 0.72 : 5.1 |
94.4 |
67.1 |
115.4 |
G |
6 : 0.72 : 5.1 |
96.9 |
95.8 |
115.4 |
H |
8 : 0.72 : 5.1 |
92.7 |
80.1 |
118.0 |
M |
10 : 0.72 : 5.1 |
92.4 |
78.8 |
116.8 |
C. Results and discussion
Fig. 3 shows the emission spectra of WLEDs using the phosphor combination of either LuAG and SCASN or LuAG and KSF. The LER of SCASN-loaded WLED is a little bit higher than that of KSF-loaded WLED (95.0 vs. 92.4 lm W−1). This enhancement is mainly attributed to the sequential emission spectra of SCASN and a larger portion of spectral region close to the eye sensitivity curve. Moreover, the SCASN-loaded WLED yields much higher CRIs (Ra = 83.7 and R9 = 16.6) than the KSF-loaded WLED (Ra = 60.9 and R9 = −28.2). However, these CRIs, typically R9, are too low to be acceptable for high quality lighting applications, indicating that using a single red phosphor cannot realize superior CRIs with both high Ra and R9 (except for using an extremely broad red phosphor in ref. 22). To further increase the CRIs, adding KSF into the phosphor blend of LuAG and SCASN to greatly enhance the red spectral part longer than 630 nm is attempted.
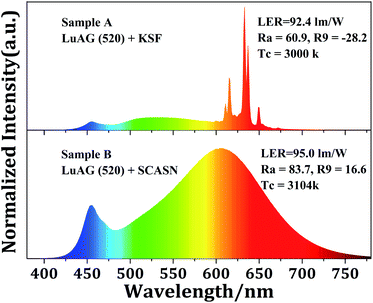 |
| Fig. 3 Emission spectra of the WLEDs based on the combination of a InGaN blue-LED chip with LuAG and (a) SCASN or (b) KSF. | |
As seen in Fig. 4, the addition of KSF makes a minute variation in the green and red spectral region (500–600 nm), but yields obvious changes in the red spectra part of 600–680 nm in the spectral power distribution (SPD) of WLEDs. With increasing the amount of KSF, the luminous intensity of several narrow peaks enhances dramatically, owing to the strong absorption and high conversion efficiency of blue light of KSF. Oppositely, the luminous intensity of SCASN decreases correspondingly, leading to a small reduction in the intensity of the red continuous spectrum. These variations definitely change the luminous efficacy and colour rendering properties of WLEDs.
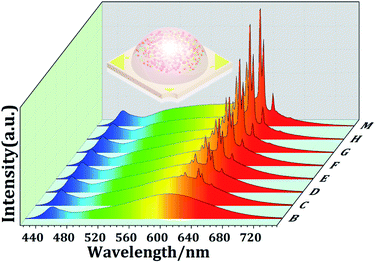 |
| Fig. 4 Spectral power distributions of WLEDs prepared by using different phosphor blends. | |
Calculations were carried out to explore the factors that affect CRIs, as given in the Chart 1. ΔEi is the color difference of 14 selected Munsell samples illuminated by test light and the standard light. Ra and Ri are the average of the first 8 color rendering indices and the special color rendering index of each sample, respectively. ΔEi is closely related to the inner product of the test spectral power distribution and the spectral reflexivity of red samples. As shown in Fig. 5a, one can find that the calculated Ra shows a similar tendency and approximately coincides with the experimental one. The WLED with uniformly distributed luminous intensity would have a great Ra in the visible region. Owing to the characteristic spectral power distribution with enhanced intensity in the red part spectral, Ra increases continuously with increasing KSF, and reaches a maximum (calculated: 93.8, experimental: 96.9) for sample G. Fig. 5b presents the measured R9 and the spectral luminance factor of R9. Similar to Ra, R9 shows the same trend as the amount of KSF increases, and reaches a maximum of 95.8 for sample G. As seen, the spectral luminance factor of R9 is close to zero when the wavelength is below 600 nm. With the adding of KSF, several strong sharp emission peaks appear at 613, 631 and 647 nm, which dramatically supplement the red spectral part. As a result, R9 advances with the degradation of ΔE9. It thus validates the feasibility of using additional KSF to improve R9 of WLEDs. However, the decline of R9 with further increasing KSF is due to the increased optical loss such as scattering loss, total internal reflection loss and Fresnel loss that exceeds the spectral improvement.
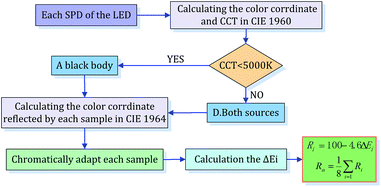 |
| Chart 1 Procedure of the calculation about CRIs CCT < 5000 K: choosing a reference source by the value of CCT. Color coordinate in CIE 1964: calculating the color coordinate of the both light sources (the test and the reference) reflected by each sample in the CIE 1964 color space. Chromatically adapt each sample: using a von Kries transform to chromatically adapt each sample. | |
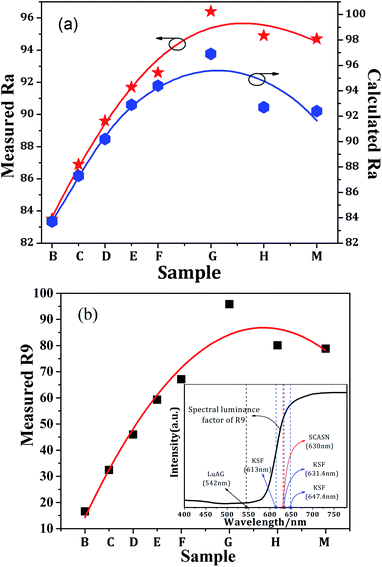 |
| Fig. 5 Measured and calculated Ra (a), measured R9 (b) of WLEDs with different phosphor blending ratios. The inset of (b) shows the spectral luminance factor of R9. | |
LER is derived via the ratio of the number of luminous flux and the input power, manifesting the capability of converting input current into white light.
|
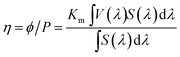 | (1) |
Where
ϕ is luminous flux,
P is the power of input,
Km is the luminous efficacy of radiation (about 683 lm W
−1), and
V(
λ) is the 1924 CIE relative luminous efficiency function of photopic vision that is defined in the visible range of 380–780 nm (shown in the inset of
Fig. 6),
S(
λ) is the spectral power distribution of WLED.
30 As seen in
Fig. 6, the luminous efficacy of WLEDs increases initially as the amount of KSF increases, and then stabilizes at 115–118 lm W
−1 even if KSF is continuously added. The increment of LER could be ascribed to the facts that (i) in the beginning the addition of the KSF does not lead to large optical loss that reduces LER; (ii) according to
eqn (1), the luminous flux is weighted by the luminous efficiency function of photopic vision. The emission intensity near the eye's response curve increases with the addition of KSF, leading to the increasing luminous flux; (iii) less re-absorption occurs for KSF, and the green light contributing dominantly to LER does not loss. With further increasing KSF, the luminous efficacy does not change nearly, ascribing to the balance between the increasing optics loss and the enhancing red spectral part of KSF. Finally, both the high luminous efficacy (115 lm W
−1) and high colour rendering indices (Ra = 96.9 and R9 = 95.8) are realized in sample G with the KSF:SCASN:LuAG ratio of 6
![[thin space (1/6-em)]](https://www.rsc.org/images/entities/char_2009.gif)
:
![[thin space (1/6-em)]](https://www.rsc.org/images/entities/char_2009.gif)
0.72
![[thin space (1/6-em)]](https://www.rsc.org/images/entities/char_2009.gif)
:
![[thin space (1/6-em)]](https://www.rsc.org/images/entities/char_2009.gif)
1.
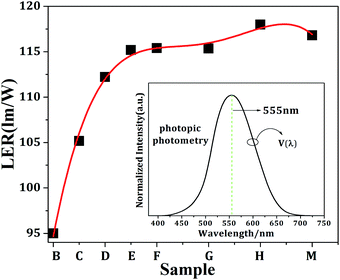 |
| Fig. 6 Measured LER of WLEDs with different amounts of KSF phosphor added. The 1924 CIE relative luminous efficiency function for photopic vision can be seen in the inset. | |
D. Conclusions
In summary, the trade-off between luminous efficacy and colour rendering indices of WLEDs is greatly alleviated by using the dual red phosphors consisting of a broad band SCASN and a narrow-band KSF. That combining a single red phosphor of either SCASN or KSF with LuAG leads to high LER but low R9. With the dual red phosphor strategy, both the luminous efficacy and colour rendering indices increase obviously, and reach the maximum of LER = 115 lm W−1, Ra = 96.9 and R9 = 95.8. The increments of LER and CRIs are attributed to the enhanced red spectral part in the range 600–680 nm and the absence of re-absorption of KSF. The WELDs with both high LER and CRIs prepared in this work are expected to be used in a broad range of lighting applications.
Funding
This work was financially supported by the National Natural Science Foundation of China (No. 61575182, 61405183, 5157223, and 51561135015), the Natural Science Foundation of Zhejiang Province (No. LY16F050004), the Research Grants Council of the HKSAR Government (No. PolyU 5228/13E) and the Applied Research Program of Commonweal Technology of Zhejiang Province (No. 2015C31102).
Acknowledgements
We thank Sunpu Opto Semiconductor Co. Ltd for the support of measurement and Dr Lu Lu at Beijing Yuji-Xinguang Optoelectronic Technology Co. Ltd for the support of LED packaging. We are grateful to the discussions with Dr Tianliang Zhou at College of Materials, Xiamen University.
Notes and references
- Y. Yuan, R. Zheng, Q. Lu, W. Zhang, J. Zheng and W. Wei, Opt. Lett., 2016, 41, 3122–3125 CrossRef PubMed.
- L. Wang, X. Wang, T. Takeda, N. Hirosaki, Y. T. Tsai, R. S. Liu and R.-J. Xie, Chem. Mater., 2015, 27, 8457–8466 CrossRef CAS.
- R. Mirhosseini, M. F. Schubert, S. Chhajed, J. Cho, J. K. Kim and E. F. Schubert, Opt. Express, 2009, 17, 10806–10813 CrossRef CAS PubMed.
- Y. Ohno, Opt. Eng, 2005, 44, 111302 CrossRef.
- Y. Ohno, Proc. SPIE-Int. Soc. Opt. Eng., 2004, 5530, 88–98 CrossRef.
- P. J. Pardo, E. Cordero, M. I. Suero and Á. L. Pérez, J. Opt. Soc. Am. A, 2016, 33, A248–A254 CrossRef PubMed.
- G. Cheng, M. Mazzeo, S. D'Agostino, S. F. Della, S. Carallo and G. Gigli, Opt. Lett., 2010, 35, 616–618 CrossRef PubMed.
- F. Viénot, E. Mahler, J. J. Ezrati, C. Boust, A. Rambaud and A. Bricoune, J. Light Visual Environ., 2008, 32, 208–213 CrossRef.
- R.-J. Xie, Nitride phosphors and solid-state lighting, CRC/Taylor & Francis, 2011 Search PubMed.
- S. E. Brinkley, N. Pfaff, K. A. Denault, Z. Zhang, H. T. Hintzen, R. Seshadri, S. Nakamura and S. P. Denbaars, Appl. Phys. Lett., 2011, 99, 241106 CrossRef.
- X. Piao, T. Horikawa, H. Hanzawa and K. Machida, Appl. Phys. Lett., 2006, 88, 161908 CrossRef.
- K. V. D. Eeckhout, P. F. Smet and D. Poelman, Materials, 2011, 4, 980–990 CrossRef.
- H. Watanabe, H. Wada, K. Seki, M. Itou and N. Kijima, J. Electrochem. Soc., 2008, 155, F31–F36 CrossRef CAS.
- X. Piao, K. Machida, T. Horikawa, H. Hanzawa, Y. Shimomura and N. Kijima, Chem. Mater., 2007, 19, 4592–4599 CrossRef CAS.
- K. Uheda, N. Hirosaki, Y. Yamamoto, A. Naito, T. Nakajima and H. Yamamoto, Electrochem. Solid-State Lett., 2006, 9, H22–H25 CrossRef CAS.
- C. C. Lin, Y. S. Zheng, H. Y. Chen, C. H. Ruan, G. W. Xiao and R. S. Liu, J. Electrochem. Soc., 2010, 157, H900–H903 CrossRef CAS.
- P. F. Smet, A. B. Parmentier and D. Poelman, J. Electrochem. Soc., 2011, 158, R37–R54 CrossRef CAS.
- S. X. Li, L. Wang, Q. Q. Zhu, D. M. Tang, X. J. Liu, G. Cheng, L. Lu, T. Takeda, N. Hirosaki, Z. Huang and R.-J. Xie, J. Mater. Chem. C, 2016, 4, 11219–11230 RSC.
- J. Zou, B. Yang, J. Li, S. Zhu, X. Qian and F. Wang, Ceram. Int., 2016, 42, 14956–14962 CrossRef CAS.
- P. Pust, V. Weiler, C. Hecht, A. Tücks, A. S. Wochnik, A. K. Henß, D. Wiechert, C. Scheu, P. J. Schmidt and W. Schnick, Nat. Mater., 2014, 13, 891–896 CrossRef CAS PubMed.
- L. Wang, R.-J. Xie, Y. Li, X. Wang, C. G. Ma, D. Luo, T. Takeda, Y. T. Tsai, R. S. Liu and N. Hirosaki, Light: Sci. Appl., 2016, 5, e16155 CrossRef CAS.
- J. H. Oh, H. Kang, Y. J. Eo, H. K. Park and Y. R. Do, J. Mater. Chem. C, 2015, 3, 607–0615 RSC.
- H. Zhu, C. C. Lin, W. Luo, S. Shu, Z. Liu, Y. Liu, J. Kong, E. Ma, Y. Cao and R. S. Liu, Nat. Commun., 2014, 5, 4312 CAS.
- C. X. Liao, R. P. Cao, Z. J. Ma, Y. Li, G. P. Dong, K. N. Sharafudeen and J. R. Qiu, J. Am. Ceram. Soc., 2013, 96, 3552–3556 CrossRef CAS.
- D. Cui, Q. Xiang, Z. Song, Z. Xia and Q. Liu, J. Mater. Chem. C, 2016, 4, 7332–7338 RSC.
- P. Pust, A. S. Wochnik, E. Baumann, P. J. Schmidt, D. Wiechert, C. Scheu and W. Schnick, Chem. Mater., 2014, 26, 3544–3549 CrossRef CAS.
- S. W. Kim, T. Hasegawa, S. Hasegawa, R. Yamanashi, H. Nakagawa, K. Toda, T. Ishigaki, K. Uematsu and M. Sato, RSC Adv., 2016, 6, 61906–61908 RSC.
- X. Zhang, Y. T. Tsai, S. M. Wu, Y. C. Lin, J. F. Lee, H. S. Sheu, B. M. Cheng and R. S. Liu, ACS Appl. Mater. Interfaces, 2016, 8, 19612–19617 CAS.
- L. Wang, X. Wang, T. Kohsei, K.-i. Yoshimura, M. Izumi, N. Hirosaik and R.-J. Xie, Opt. Express, 2015, 23, 28707–28717 CrossRef CAS PubMed.
- A. Zukauskas, R. Vaicekauskas, F. Ivanauskas, R. Gaska and M. S. Shur, Appl. Phys. Lett., 2002, 80, 234 CrossRef CAS.
|
This journal is © The Royal Society of Chemistry 2017 |