Robust liquid-repellent coatings based on polymer nanoparticles with excellent self-cleaning and antibacterial performances†
Received
29th July 2016
, Accepted 17th November 2016
First published on 17th November 2016
Abstract
In this work, quaternary ammonium salt (QAS) functionalized fluorinated copolymer tethered hydroxyl groups were synthesized by free radical polymerization. And then novel liquid-repellent and antibacterial nanocomposite coatings were prepared via cross-linking the fluorinated copolymer and poly(urea-formaldehyde) nanoparticles (PUF NPs) containing active methylol groups with hexamethylene diisocyanate. The surface physical and chemical properties of the nanocomposite coating were systematically characterized by a series of measurements, demonstrating that the liquid-repellent surface with a dual hierarchical structure was obtained by the introduction of PUF NPs. The nanocomposite coating displayed superb self-cleaning and liquid-repellent properties, and could also maintain its superhydrophobicity even after 16 abrasion cycles and 20 cycles of cross-cut tape test. Moreover, the nanocomposite coating with over 0.11% surface concentration of N+ exhibited excellent antibacterial activity against Gram-negative Escherichia coli and Gram-positive Staphylococcus aureus. These results conclusively indicated that nanocomposite coatings had great promise for potential application in a wide range of practical fields.
1. Introduction
Inspired by some natural species, such as lotus leaves,1 rice leaves, butterfly wings,2 geckos,3 seaweed,4etc., superhydrophobic surfaces (SHS) with a high water contact angle (>150°) and low sliding angle (<10°)5 have received tremendous interest from both the scientific and industrial fields.6,7 Surfaces with such special superwetting behaviors promise an extensive range of practical applications including anti-icing,8 anti-frost,9 anti-corrosion,10 self-cleaning,11,12 drug release,13,14 marine antifouling coatings,15 water/oil separation,11,16,17 and oil purification.18 The preparation of artificial superhydrophobic surfaces usually involves surface modification via surface etching, sol–gel processes, photolithography, layer-by-layer deposition, electrodeposition, or filling particles, resulting in multiscale roughness of surfaces, sometimes combined with additional chemical modifications to reduce surface energy.19–26 Lots of efforts have been devoted to fabricating SHS by spraying the dispersions of various inorganic nanoparticles,8,27–32 carbon nanomaterials,9,33 pigments,34 and clay,35 in a polymer matrix. However, the big drawback of these artificial surfaces via filling inorganic particles is their low robustness caused by the incompatibility between the particles and the organic polymer matrix.30
Microbial contamination has always been detrimental to human health in daily life and medical procedures.36 Many kinds of bacteria may bring serious illness even death to people.37,38 Thus, it is of great importance and necessity to develop multifunctional antibacterial materials. Since the adhesion of bacteria to the substrate is the first stage of bacterial colonization and subsequent biofilm formation,39 the preparation of surfaces preventing the initial adhesion of bacteria to the substrate becomes one alternative stratagem to solve the problem. Superwetting surfaces with antibacterial properties have attracted much attention of researchers recently. M. Zhang et al. prepared a superhydrophobic surface containing a bimetallic composition (Cu/Ag) that exhibited certain antibacterial activity using a galvanic replacement approach followed by a thermal oxidation process.40 M. Wu et al. fabricated colored cotton fabrics with antibacterial and self-healing superhydrophobic properties by the solution-dipping method involving sequential deposition of branched poly(ethylenimine) (PEI), silver nanoparticles (AgNPs), and fluorinated decyl polyhedral oligomeric silsesquioxane (F-POSS) on cotton fabrics.41 These studies are useful in combining the antibacterial performance with superhydrophobicity by blending the antibacterial agent in the substrate. However, the ‘Achilles' heel’ of these materials is that the antibacterial performance relying on the release of antibacterials is time limited.42 Therefore, long-term, effective and environmentally friendly antibacterial materials are highly required.43,44 Quaternary ammonium salts (QAS) with an alkyl chain substituent longer than 8 carbon atoms have been widely used as antibacterials to kill microorganisms including bacteria, yeasts and molds,45,46 owing to their strong durable antimicrobial activity and nontoxicity without releasing heavy-metal ions.47–51 Nevertheless, to the best of our knowledge, few previous papers appear to have been reported for the preparation of SHS with antibacterial properties by incorporation of QAS groups.
The motivation of this work is to develop robust multifunctional materials displaying excellent self-cleaning, liquid-repellent and unique antimicrobial performances. Herein, reactive polymer nanoparticles, crosslinked poly(urea-formaldehyde) (PUF), were used to construct a surface with high roughness and provide coupling points to achieve good compatibility between particles and the polymer matrix. PUF is a widely used adhesive for bonding wood products, automobile tires and electrical devices because of its fast curing, thermotolerance, abrasion resistance and low price.52–54 A transmission electron microscopy (TEM) image (JEM-2100, Japan, operating at 200 kV) of pristine PUF NPs is provided in Fig. S1.† In this study, novel durable surfaces with special self-cleaning, liquid-repellent and antibacterial activities were fabricated by crosslinking of the synthesized QAS-functionalized fluorinated copolymer bearing hydroxyl groups, reactive PUF nanoparticles (PUF NPs) and hexamethylene diisocyanate (HMDI). The microstructure and morphology of the nanocomposite surface were characterized by Fourier transform infrared spectroscopy (FT-IR), scanning electron microscopy (SEM), and atomic force microscopy (AFM). The surface composition and superwetting properties of the coating were investigated in detail. Furthermore, the self-cleaning, liquid-repellent, abrasion resistant and antibacterial performances were assessed by a series of tests.
2. Experimental section
2.1 Materials
Butyl methacrylate (BMA) was purchased from Sinopharm Chemical Regent CO., Ltd. 3,3,4,4,5,5,6,6,7,7,8,8,8-Tridecafluorooctyl acrylate (TFOA), 2-hydroxyethyl methacrylate (HEMA), hexamethylene diisocyanate (HMDI), dibutyltin dilaurate (DBTDL) and 1-docosanethiol were purchased from Aldrich. The QAS monomer QDEMA was synthesized as described in a previous study.55 2,2′-Azobisisobutyronitrile (AIBN, Aldrich) was recrystallized from ethanol. All the monomers except QDEMA were filtered through a basic alumina column to remove the radical inhibitor. Poly(urea-formaldehyde) (PUF) nanoparticles, PERGROPAK™ M7 (size diameter 110 nm, denoted as PUF NPs), were provided by Beijing THK Sci. CO., Ltd. Butyl acetate and acetonitrile were obtained from Sinopharm Chemical Reagent and used as received. The human blood we employed in the experiments was heparinized and kindly provided by Zhejiang University Hospital. All the experiments involving the use of human subjects were conducted in compliance with the relevant laws and institutional instructions, such as the Declaration of Helsinki and Approaches to the Ethical Review of Biomedical Research Involving Human Subjects (China, 2007). The committee of Zhejiang University has approved the experiments. Escherichia coli (E. coli) and Staphylococcus aureus (S. aureus) were obtained from the Microorganism Institute of Zhejiang University. The culture medium was commercial.
2.2 Synthesis of the quaternary ammonium salt (QAS) functionalized fluorinated copolymers
The QAS-functionalized fluorinated copolymer was synthesized by free radical solution polymerization. The synthesis procedure is described in Scheme S1.† Typically, 3.5 g of BMA, 4.0 g of TFOA, 2.0 g of QDEMA and 0.5 g of HEMA were dissolved in 10 g mixture of butyl acetate and acetonitrile with nitrogen protection. Then 0.15 g of AIBN as the initiator was added into the reactor at 60 °C, with subsequent addition of 1-docosanethiol to control the reaction. After 12 h of polymerization, the crude reaction solution was purified by rotary evaporation to remove most of the solvent. Then, the copolymer was precipitated in n-hexane three times, and finally dried in a vacuum oven at 50 °C. The synthesized functionalized copolymers were designated as FC. The recipes of polymerization are summarized in Table 1.
Table 1 Compositions of FC copolymers
Samples |
BMA/HEMA/TFOA/QDEMAa |
TFOA content (wt%) |
QDEMA content (wt%) |
Feed weight ratio.
|
FC1 |
7/1/12/0 |
60 |
0 |
FC2 |
7/1/8/4 |
40 |
20 |
FC3 |
7/1/8/6 |
40 |
30 |
FC4 |
7/1/6/6 |
30 |
30 |
2.3 Preparation of PUF–FC nanocomposite coatings
In this step, as shown in Scheme 1, the FC copolymer dissolved in the mixture of butyl acetate and acetonitrile was mixed with HMDI by ultrasonication for 10 min, and then PUF nanoparticles were added and ultrasonicated for another 10 min. The obtained mixture was sprayed onto glass slides or silicon wafers or other substrates using a spray gun (nozzle diameter, 0.2 mm; HD® 180, No. 302, Taiwan). The spraying pressure of the spray gun was 22 psi; the amount of mixture sprayed was about 0.08 mL cm−2; the spraying distance between the spray gun and substrate surface was 7 cm. PUF–FC nanocomposite films were allowed to cure for 2 h at room temperature, followed by an additional 12 h drying in an oven at 120 °C. Four film samples were prepared in this work. The thickness of PUF–FC nanocomposite films was measured by Bruker's Stylus Profiler (DEKTAK-XT®, Boyue Instruments (Shanghai) Co., Ltd), as listed in Table S1.† The detailed compositions of PUF–FC nanocomposite films are listed in Table 2. As controls, pure copolymer films were also prepared by spraying the mixture of the FC copolymer and solvents onto substrates, and then curing in a similar way.
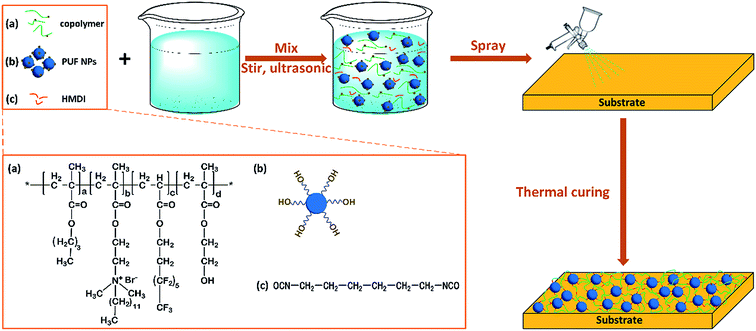 |
| Scheme 1 Synthesis route to the PUF–FC coating. | |
Table 2 Compositions of PUF–FC nanocomposite films
Samples |
Polymer/PUF/HMDIa |
Mass ratiob |
Feed weight ratio.
Mass ratio represents the m(QDEMA) : m(TFOA) of the copolymer.
|
PUF–FC1 |
7/3/0.28 |
0 : 6 |
PUF–FC2 |
7/3/0.28 |
2 : 4 |
PUF–FC3 |
7/3/0.28 |
3 : 4 |
PUF–FC4 |
7/3/0.28 |
3 : 3 |
2.4 Characterization
FT-IR spectra were measured on a Nicolet 5700 FT-IR spectrometer to confirm the structure of the samples by using the KBr pellet method.
1H-nuclear magnetic resonance (1H-NMR) spectra were conducted on the obtained copolymers by using a Bruker Avance 500 MHz (25 °C). The test copolymers were dissolved in deuterated chloroform (CDCl3), and all chemical shifts were recorded in ppm (d).
SEM analysis (SIRISON, FEI Co., Ltd) and AFM were applied to examine the surface morphologies of the sample surfaces. AFM was operated in tapping mode using Multi Mode (Veeco, USA). The scanning range of the samples was 5 μm × 5 μm. The roughness value, root-mean-square (RMS), was calculated from the AFM image.
The surface composition of the coating was analyzed by X-ray photoelectron spectroscopy (XPS; Thermo Scientific, USA) with an Al Kα X-ray source. The X-ray gun was operated at 14 kV and 350 mW, and the analyzer chamber pressure was 10−9 to 10−10 Pa.
The surface wettability was investigated by water contact angle (WCA) measurements performed on a CAM 200 optical contact-angle goniometer (KSV Co., Ltd, Helsinki, Finland) at room temperature. The volume of the tested water droplet is 5 μL. The sliding angles (SAs) of the drops were measured by tilting the sample plate from zero to a higher angle until the droplet rolled off the surface.
The mechanical abrasion test was carried out on a chosen coating surface. The coated glass slide weighing 180 g was placed face-down on sandpaper and moved for 10 cm along the ruler and reversely moved for another 10 cm along the ruler. This process is regarded as one abrasion cycle. The cross-cut tape test was also conducted by cycles of attachment and detachment processes using double-sided adhesive tape. A new adhesive tape was used for each new cycle.
Self-cleaning effects of the nanocomposite coating surfaces were studied by contaminating the surfaces using copper(II) chloride dihydrate (CuCl2·2H2O) particles respectively, and then removing the contaminants using water droplets.
The antibacterial performances of the nanocomposite coatings were investigated against E. coli and S. aureus with the plate count method, as described elsewhere.56,57 Basically, aliquots of the 108 cfu mL−1 log phase E. coli suspension and S. aureus suspension were prepared in Luria–Bertani media. The coating samples on coverslips (2 × 2 cm2) were immersed in 5 mL of E. coli suspension or S. aureus suspension (108 cfu mL−1) and incubated in a shaking incubator at 37 °C for 3 h. The coating samples were then gently swabbed with a sterile cotton swab; they were then placed in tubes with 10 mL of Ringer's solution, sonicated for 5 min, and vortexed for 30 s to collect the adherent bacterial cells on the coverslips. Six 10-fold dilutions were prepared, and the surviving bacteria were counted by the spread plate method (100 μL samples of the decimal dilutions were spread on a Petri dish that contained Luria–Bertani agar). The Petri dishes were incubated at 37 °C overnight. After incubation, the colonies were counted. The percentage reductions in bacterial colonies, as compared to an uncoated coverslip (as a blank control), were reported as the mean value of three replicate samples. Specifically, the bactericidal rate (Eb) was calculated by the following equation:
where
Nb and
Nc are the numbers of colonies (which means the surviving bacterial cells) corresponding to the uncoated coverslip and nanocomposite coating samples, respectively.
3. Results and discussion
3.1 Structural characterization
The copolymer FC was first synthesized by free radical solution polymerization of BMA, TFOA, QDEMA, and HEMA. The FT-IR spectra were recorded after polymerization to verify the functional groups of FC. As shown in Fig. S2,† the peak at 3300 cm−1 was assigned to the stretching vibration of the hydroxyl –OH in PHEMA. The existence of the C–N+ stretching vibration band was confirmed by the band at 1450 cm−1 in the spectrum of PQDEMA. The bands at 1140 and 1250 cm−1 corresponded to the CF2 and CF3 groups, respectively. The characteristic absorptions of C
O (1735 cm−1) were clearly visible. To further confirm the structure, 1H-NMR spectra of copolymer FC2 are provided in Fig. S3.† The chemical shift at 3.94 ppm could be assigned to –CH2OH from PHEMA. The signals at 3.75 and 3.47 ppm were assigned to CH2N+(CH3)2 and CH2N+(CH3)2 from PQDEMA, respectively. The peaks observed at 2.5 ppm were assigned to the –CH2CF2– group in PTFOA. Both of the FT-IR and 1H-NMR results showed that the QAS-functionalized fluorinated copolymers were successfully synthesized by the free radical polymerization method.
A simple crosslinking process was conducted to fabricate a PUF–FC nanocomposite coating by mixing FC, PUF NPs, and HMDI. Both the copolymer FC and PUF NPs contain hydroxyl groups, which can react with the isocyanate groups of HMDI. The mixture of these reactants in butyl acetate and acetonitrile solution was sprayed onto substrates, followed by thermal curing to obtain the PUF–FC nanocomposite coating. The chemical structures of PUF and synthesized PUF–FC were identified by FT-IR spectra shown in Fig. 1. For PUF, the broad bands around 3440 cm−1 were assigned to the N–H and –OH groups. Other broad bands at 1629–1540 cm−1 were ascribed to the –CONH stretching vibration. For PUF–FC1, PUF–FC2, PUF–FC3, and PUF–FC4 samples, the two broad bands around 3440 and 1629–1540 cm−1 were separately assigned to N–H and NH2 from PUF. The peak at 1490 cm−1 was ascribed to the C–N+ group, which could be seen from all the samples except PUF and PUF–FC1. The two new broad bands at 1745 and 1250–1140 cm−1 corresponded to the C
O and C–F groups from FC. More importantly, the peaks at 2272 cm−1 corresponding to NCO groups were not observed for these four samples, indicating the complete reaction among the QAS-functionalized fluorinated copolymer, HMDI, and PUF.
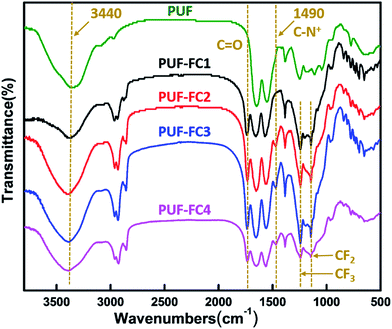 |
| Fig. 1 FT-IR spectra of PUF, PUF–FC1, PUF–FC2, PUF–FC3 and PUF–FC4 from the top to the bottom, respectively. | |
3.2 Surface topography
The surface topographies of the pure copolymer coating and the nanocomposite coating were investigated by SEM and AFM, respectively. Fig. 2a and b show the SEM images of the pure copolymer coating FC2 and the nanocomposite coating PUF–FC2. It was found that under the same magnification, FC2 had a smooth surface with no distinct apophyses (Fig. 2a), while, with the addition of PUF NPs, micro/nano-scaled protuberances and cavities could be clearly seen from the surface of PUF–FC2 (Fig. 2b). These protuberances and cavities were formed due to agglomeration of PUF NPs, and larger-sized cavities could trap more air in pockets on the liquid/solid interface. Hence the water droplet cannot penetrate into the surface, resulting in high CAs and low adhesion. Therefore, the nanocomposite coating with a hierarchical structure was obtained by the crosslinking reaction of the functionalized copolymer and HMDI with addition of PUF NPs.
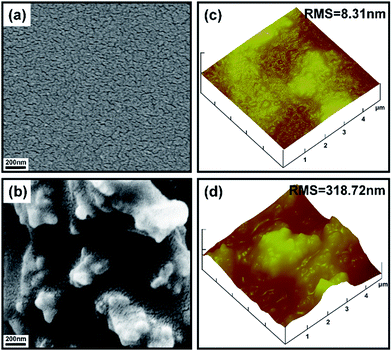 |
| Fig. 2 SEM images of FC2 (a) and PUF–FC2 (b) and the typical three-dimensional AFM height images of FC2 (c) and PUF–FC2 (d). | |
According to SEM studies, it's undoubted that the rugged surface with a dual micro–nanostructure was fabricated by adding PUF NPs. Tapping mode AFM was applied to further analyze the surface structure of the nanocomposite coating at the microscale. Three-dimensional AFM height images of FC2 and PUF–FC2 coatings are presented in Fig. 2c and d. For the pure copolymer coating, a relatively low roughness surface was observed in the scanning range (Fig. 2c). The root-mean-square (RMS) value of this sample is 8.31 nm. Fig. 2d reveals the surface morphology of the PUF–FC2 coating, the RMS roughness of which is 318.72 nm. This indicated that the surface roughness could be greatly increased by the introduction of PUF NPs. The surface topography gained by AFM images was in good agreement with the results from SEM characterization.
3.3 Surface chemical compositions
The chemical composition of the nanocomposite coating surfaces was determined by XPS analysis. Fig. 3 shows the XPS spectra of PUF–FC surfaces. In the wide scan spectra, the surface elemental atomic percentages of the coatings were calculated (inserted into the figure), and XPS characteristic signals C 1s (284.8 eV), O 1s (545.2 eV), N 1s (399.3 eV), and F 1s (685.5 eV) were all observed. The signal F 1s was naturally attributed to the fluorinated segments of the copolymer; the N 1s peak, however, was assigned to the PUF NPs together with the QAS segments of the copolymer. To further ascertain the QAS content, the high resolution XPS spectra of the N 1s for each coating were analyzed, as displayed in Fig. S4.† The presence of N 1s peaks was resolved into C–N and C–N+, which appeared at 399.3 eV and 402.0 eV, respectively, and the percentages of C–N and C–N+ were recorded. The emergence of C–N+ peaks could be obviously seen in the spectra of all the coating surfaces except the PUF–FC1 surface, which is consistent with the results obtained by FT-IR. F and N+ concentrations on the surface from XPS analysis as well as in the bulk were calculated and are shown in the inserted table of Fig. 3.
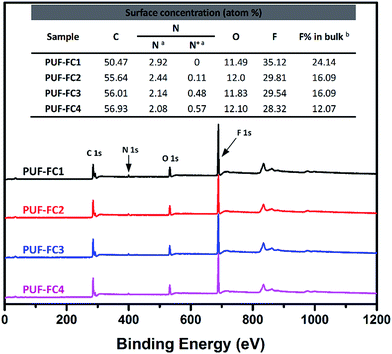 |
| Fig. 3 XPS spectra of PUF–FC1, PUF–FC2, PUF–FC3, and PUF–FC4 coatings. The inset table shows surface elemental concentrations of the samples. (a) The surface concentrations of two different N types were obtained from the high resolution of the N 1s peak. (b) The F atom% in the bulk was calculated on the basis of the known composition of the nanocomposite coating. | |
As listed in the table, for each coating sample, the concentration of F on the surface is higher than that in the bulk due to the enrichment of fluorinated segments on the surface. This will endow the coating with very low surface energy. Notably, when the same dosage of PUF NPs was added, the surface elemental atom percentages varied with the mass ratio of QDEMA and TFOA. When the mass ratio was 0
:
6, the F concentration was highest. However, when the mass ratio increased, the F atom percentage reduced, and N+ concentration accordingly got raised. These results may have an influence on the surface wettability and antibacterial activity of the nanocomposite coating.
3.4 Surface wettability and mechanical properties of the nanocomposite coating
It is widely accepted that the topographical structure and chemical compositions are two critical factors affecting the wettability of the solid material surface. The wetting behaviors of both pure copolymer coatings and nanocomposite coatings were investigated by the static CA and SA measurements. The static CA of water on FC-coated surfaces varied from 111.3 ± 1.6° to 105.2 ± 1.5°, as shown in Table S2,† which indicated that the surfaces of the FC samples were hydrophobic without the addition of PUF NPs. However, once a certain dosage of PUF NPs was added, the surface hydrophobicity of the nanocomposite coating increased significantly, even to superhydrophobicity.
As shown in Fig. 4, for the PUF–FC1 coating with the mass ratio (mQDEMA/mTFOA) 0
:
6, the CA reached as high as 162.3 ± 3.2°, and the SA was 1.4 ± 0.2°, while, when the mass ratio rose to 3
:
4, just as the PUF–FC3 coating, the CA decreased to 152.0 ± 1.7°, and the SA increased to 9.5 ± 1°. The superhydrophobicity of these surfaces would guarantee outstanding self-cleaning properties. With further increasing the mass ratio to 3
:
3, the CA of the PUF–FC4 surface dropped to 147.8 ± 2.3°, and accordingly the SA increased obviously to 17.2 ± 2°. This phenomenon may be explained by the interplay of fluorinated segments and QAS groups. It has been demonstrated that the surface of the pure copolymer coating was smooth with a low RMS, as shown in Fig. 2a and c, so the wettability was mainly determined by the chemical compositions. Nevertheless, after the introduction of PUF NPs, the surface topography of the composite coating changed from the nanostructure to a micro/nano-multiscale structure (Fig. 2). Hence, the topographical structure became the main impact factor of wetting properties as well as chemical compositions. Owing to the micro–nanostructure and fluorination, a superhydrophobic coating was obtained.
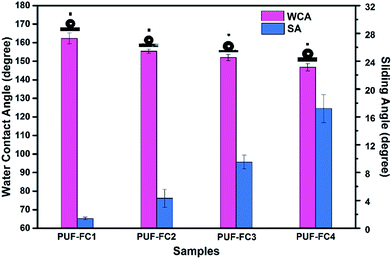 |
| Fig. 4 Water contact angles and sliding angles of the nanocomposite coatings PUF–FC1, PUF–FC2, PUF–FC3 and PUF–FC4. The red column represents WCAs, and the blue column represents SAs. Images of the contact angles appear in the insets. | |
Although a large number of artificial superhydrophobic coatings have been achieved, many of them suffer mechanical damage or are vulnerable to mechanical pressure. Besides, most superhydrophobic coatings with high roughness easily lose the superhydrophobicity once touched because the surface roughness is usually at the micro- or nanoscale and is mechanically weak and readily abraded.58 Therefore, low surface robustness is becoming the main challenge limiting the widespread applications of superhydrophobic coatings. In this section, the PUF–FC2-coated surface was chosen to evaluate the mechanical performance by the abrasion test and the cross-cut tape test. The coated glass slide weighing 180 g was placed face-down on sandpaper and moved in cycles along the ruler. Fig. 5A demonstrates the results of wettability evolution during the abrasion procedure. It was observed that the static WCA fluctuated in a small range between 162.8° and 152.4°, and that the WCA still remained above 150° after 16 abrasion cycles. The whole procedure of the abrasion test was also photographed and filmed as shown in Fig. S5 and Video S1.† After multiple abrasion processes (at least 16 cycles), the surface was intact with no scratches, indicating that the superhydrophobicity of the nanocomposite coating was not affected by mechanical abrasion due to the good compatibility between PUF NPs and the FC copolymer matrix. Fig. 5B shows the photographs of the surface before and after the 20th cross-cut tape test. The pattern on the surface is not removed after the 20th removal of the tape, indicating the strong adhesion between the coating and glass slide. Meanwhile, the droplet of blue ink could maintain its spherical shape on the pressed surface, exhibiting its outstanding mechanical properties.
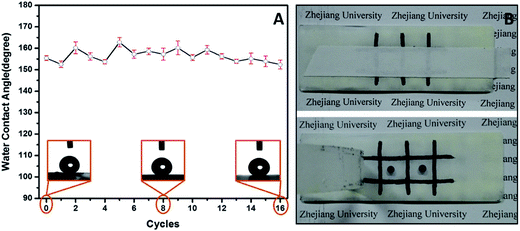 |
| Fig. 5 Mechanical durability of the coated surface during the abrasion test and the cross-cut tape test. (A) Plot of mechanical abrasion cycles and water contact angles after each abrasion test. Images of an 8 μL water drop on the PUF–FC2-coated surface before and after 8 and 16 abrasion cycles were inserted. (B) Photographs of the surface before (top) and after (bottom) the 20th cross-cut tape test. An 8 μL droplet of blue ink was placed on the pressed surface. | |
3.5 Self-cleaning properties
The self-cleaning properties of the nanocomposite coatings were demonstrated with copper(II) chloride dihydrate particles as the contaminant. As shown in Fig. 6A, a handful of contaminants were sprinkled on the surface and water was added dropwise onto the upper side of the contaminated surface afterwards with a tilt angle of around 18°. Once contact with a few droplets of water occurred, the particles on the nanocomposite coating were dissolved quickly by the droplet, and taken away by the droplet rolling off the surface. The surfaces of four nanocomposite coatings became completely clean through water droplets rolling off the surface quickly due to the high contact angle and low sliding angle. The blank glass surface, however, remained contaminated by the CuCl2 powders (Fig. 6 and Video S2†). A similar procedure was conducted on the surface of pure copolymer coatings. As seen in Fig. S6 and Video S3,† the CuCl2 powders were still stuck on all surfaces and could not be cleared off by water drops, which indicated that excellent self-cleaning properties could be achieved by the introduction of PUF NPs into copolymers.
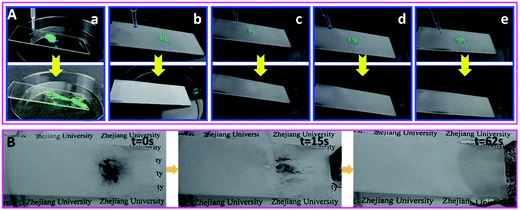 |
| Fig. 6 Self-cleaning test by (A) using copper(II) chloride dihydrate particles contaminating the surfaces, and (B) using continuous water drops to rinse the surface of PUF–FC2 contaminated by carbon particles. Images (a), (b), (c), (d), and (e) were taken before (top) and after (bottom) a few drops of water rolled off the surface of glass, PUF–FC1, PUF–FC2, PUF–FC3 and PUF–FC4, respectively. The tilt angle of the surface was about 18°. | |
To further investigate the self-cleaning properties of the nanocomposite coating to hydrophobic dusts, herein carbon particles were chosen as model contaminants. As shown in Fig. 6B and Video S4,† the carbon particle dust was easily washed away by the rolling water drops. The surface became entirely clean in 62 s, indicating an outstanding fouling-release performance of the nanocomposite coating. Due to the tendency to accumulate at the water/air interface, carbon particles could be picked by the rolling water droplet without influence on its motion; thus the composite coating surface could efficiently clear off the dust under water flow. Therefore, the nanocomposite coating surface can possess self-cleaning performance for various solid pollutants in practical applications.
3.6 Liquid repellency
As depicted above, the PUF–FC2 coatings present high self-cleaning properties for water droplets. It is of great significance to fabricate lyophobic even superlyophobic surfaces for both water and complex solutions. In this section, a series of complex liquid droplets which were commonly seen in our daily life were used to test the special liquid repellency of the nanocomposite coatings. As illustrated in Fig. 7, honey and blood droplets were chosen as test liquids. Because of their high viscosity and complicated components, honey and blood are very difficult to wipe up. The honey droplet glided down the surface in 22 s because it is too sticky to move quickly, while the blood droplet slid away fleetly in 0.7 s (Video S5†). Strikingly, these droplets left no traces along their paths, indicating that the surface was not permeated by these liquids. Other common liquids, such as tea, milk tea, coffee, albumen, soy sauce, and S. aureus bacterial suspension (1012 cell per mL), were also used to verify the ability to repel liquids. As displayed in Video S5,† all the drops of test liquids, except honey, rolled off the surface quickly in less than 2 s with no traces left, demonstrating the satisfactory liquid-repellent properties of the nanocomposite coating.
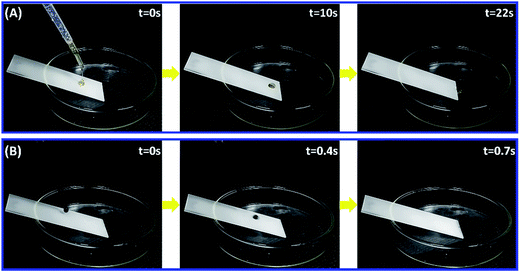 |
| Fig. 7 Snapshots of the sliding of honey (A) and human blood (B) droplets on PUF–FC2-coated glass plates. The tilt angle of plates was about 23°. | |
Due to the versatile conditions in real life, the nanocomposite coating can be applied onto various substrates using the spray method. Photos of different liquid droplets on various untreated and treated specimens with PUF–FC2 are shown in Fig. 8. Each droplet of milk tea, coffee, honey, soy sauce, bacterial suspension (1012 cell per mL), albumen, cooking oil, and human blood was placed on the surface of glass, aluminium, filter paper, and cotton fabric treated with PUF–FC2 respectively. As shown in Fig. 8, all of the untreated surfaces exhibit hydrophilic and oleophilic properties. In contrast to the uncoated surfaces, all the droplets on the coated surfaces exhibited sufficiently high contact angles and basically kept their spherical shapes, which suggested that the nanocomposite coating can endow various substrates with superlyophobic properties.
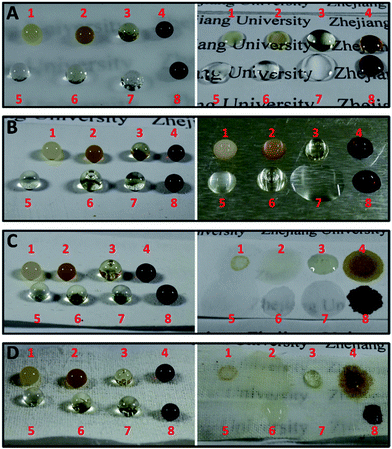 |
| Fig. 8 Photographs of the diverse liquid droplets on the PUF–FC2-coated substrates (left) and uncoated substrates (right). Substrates are (A) glass plate, (B) aluminium plate, (C) filter paper, and (D) cotton cloth. Liquids on each substrate are (1) milk tea, (2) coffee, (3) honey, (4) soy sauce, (5) bacterial suspension (1012 cell per mL), (6) albumen, (7) cooking oil, and (8) human blood, respectively. The droplet size of each liquid was 10 μL. | |
For the sake of practical use, the mechanical stability of the superhydrophobic PUF–FC2-coated cotton surface was qualitatively assessed by adhesive tape pressing. Fig. S7† reveals that the WCA of the coated cotton surface is still above 150° after 12 cycles of attachment and detachment processes using double-sided adhesive tape. These results indicate that the prepared functional nanocomposite coatings hold great potential with excellent liquid-repellent properties on various kinds of household facilities.
3.7 Antibacterial performance of the PUF–FC nanocomposite coating
QAS-functionalized surfaces exhibit biocidal activity through a contact-killing mechanism including electrostatic and lipophilic interactions with the cell wall of various microorganisms.56 More specifically, QAS groups with high positive charge density have strong electrostatic interaction with negatively charged bacteria. Thus, once adsorbed onto the bacterial cell surface, the QAS groups quickly diffuse through the cell wall to disrupt its cytoplasmic membrane and release potassium and other components to destroy the cell.
To evaluate the antibacterial properties of nanocomposite coatings, the plate count method was utilized and Gram-positive S. aureus and Gram-negative E. coli were set as model bacteria. As shown in Fig. 9, visible differences could be observed among the LB agar plates by the colony numbers. Table 3 presents the detailed data of antibacterial activities for PUF–FC calculated by the formula of bactericidal rate (Eb). As summarized in Table 3, the average Eb of PUF–FC1 with no N+ atom% on the surface was 24.1% for E. coli and 12.36% for S. aureus, respectively. The average Eb for both E. coli and S. aureus got increased with increasing N+ atom% on the surface. Surprisingly, when N+ atom% on the surface was 0.48%, the average Eb rose to nearly 100% for both bacteria. Moreover, the bactericidal rates against these two bacteria were above 82% with N+ atom% on the surface over 0.11%. The reason for the results is that the bactericidal effect of QAS segments only works on the coating surface, so the coating with a higher surface concentration of N+ (QAS) will have better antibacterial performance. Furthermore, our results also showed that the composite coatings containing QAS groups were more effective towards S. aureus than E. coli, which is in accordance with previous conclusions.59 On the basis of these data, our prepared PUF–FC nanocomposite coatings have excellent antibacterial performances, which can be applied in biomedical and related fields.
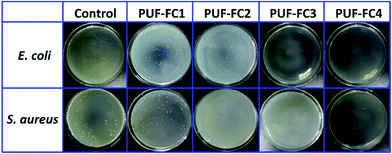 |
| Fig. 9 Antibacterial activities against the test bacteria E. coli and S. aureus after 3 h of contact time with the sample blank glass plate, PUF–FC1, PUF–FC2, PUF–FC3 and PUF–FC4. | |
Table 3 Antibacterial efficiency of the prepared nanocomposite coatings
Samples |
Average Eb for E. coli |
Average Eb for S. aureus |
Control |
0 |
0 |
PUF–FC1 |
24.1% |
12.36% |
PUF–FC2 |
82.0% |
90.2% |
PUF–FC3 |
100% |
100% |
PUF–FC4 |
99.3% |
100% |
To have a better understanding of the properties of the prepared nanocomposite coating, we have also made a comparison with some similar state-of-the-art surfaces. Since the self-cleaning property is related to superhydrophobicity, herein WCA and SA were used to evaluate self-cleaning properties. As illustrated in Table 4, there was no distinct difference in WCA among these surfaces. However, the PUF–FC surface had a SA value as low as 1.4 ± 0.2°, suggesting that the dirt could be more easily taken away by rolling water droplets. All the bacteria on the PUF–FC surface were killed with only 3 h contact, exhibiting much more efficient antibacterial activity than previous reports. Additionally, the antibacterial performance of the PUF–FC surface relied heavily on ecofriendly QAS groups, which would not cause environmental problems as the reported antibacterial SHS might do. Therefore, the nanocomposite coating having improved properties may have more applications in related areas.
Table 4 Wettability and antibacterial activity of recently reported SHS and nanocomposite surface we prepared
Surfaces |
Wettability |
Bactericidal rate (%) |
WCA (°) |
SA (°) |
Contact time (h) |
Gram-positive |
Gram-negative |
No data existed in paper.
Calculated from the data provided in paper.
|
Ag NPs coated fiber60 |
157.3 ± 1.6 |
—a |
24 |
—a |
99.99 |
Cu/Ag composition40 |
158.6 |
—a |
3 |
81.3b |
—a |
F-POSS/AgNPs/PEI41 |
169 |
3 |
3 |
87.5b |
25b |
PET@PDA@Ag2O@PFDT61 |
162.4 ± 2.5 |
3.2 ± 0.4 |
24 |
99.99 |
99.99 |
PUF–FC |
162.3 ± 3.2 |
1.4 ± 0.2 |
3 |
100 |
100 |
4. Conclusions
In summary, QAS-functionalized fluorinated coatings with micro/nano-roughness structure were efficiently fabricated by the crosslinking reaction of the functionalized copolymer and HMDI with addition of PUF NPs. The designed novel nanocomposite coating demonstrated excellent superhydrophobicity, self-cleaning and liquid-repellent properties, owing to the synergistic effect of PUF NPs and fluorinated segments. Besides, the versatility of the nanocomposite coating was confirmed by applying the coating to various substrates, suggesting much broader application areas. More importantly, the nanocomposite coating exhibited satisfactory mechanical durability even after undergoing 20 cycles of cross-cut tape test and the severe abrasion test treatments for 16 cycles under 180 g of force. The surface concentration of N+ was found to be the key factor of antibacterial performance. Thus, the nanocomposite coating had a bactericidal rate of more than 82% when N+ atom% on the surface was over 0.11%, indicating an efficient and strong antibacterial activity against E. coli and S. aureus, and feasibility of being a promising antibacterial candidate. These results show that the PUF–FC nanocomposite coatings have potential for utilization in different fields such as biomedical, building materials, household facilities, textile fabrics, and marine applications.
Acknowledgements
The authors would like to gratefully acknowledge the National Natural Science Foundation of China (NSFC) for Award No. 21476195 and 21576236 and Zhejiang Provincial Natural Science Foundation of China for Award No. Y14B060038, and Zhejiang Provincial Major Project of Science & Technology for Award No. 2014C13SAA10006 for supporting this research. We thank Prof. Yuhua Zhao (College of Life Science, Zhejiang University) for kind help with antibacterial experiments. We also thank Biyu Jin for help with shooting the movies provided in the ESI.
References
- K. Koch and W. Barthlott, Philos. Trans. R. Soc., A, 2009, 367, 1487 CrossRef CAS PubMed.
- G. D. Bixler and B. Bhushan, Crit. Rev. Solid State Mater. Sci., 2015, 40, 1 CrossRef CAS.
- G. S. Watson, D. W. Green, L. Schwarzkopf, X. Li, B. W. Cribb, S. Myhra and J. A. Watson, Acta Biomater., 2015, 21, 109 CrossRef CAS PubMed.
- Y. Cai, Q. Lu, X. Guo, S. Wang, J. Qiao and L. Jiang, Adv. Mater., 2015, 27, 4162 CrossRef CAS PubMed.
- U. Manna and D. M. Lynn, Adv. Mater., 2013, 25, 5104 CrossRef CAS PubMed.
- T. Darmanin and F. Guittard, J. Mater. Chem. A, 2014, 2, 16319 CAS.
- P. Zhang, S. Wang, S. Wang and L. Jiang, Small, 2015, 11, 1939 CrossRef CAS PubMed.
- T. Cheng, R. He, Q. Zhang, X. Zhan and F. Chen, J. Mater. Chem. A, 2015, 3, 21637 CAS.
- Y. Sohn, D. Kim, S. Lee, M. Yin, J. Y. Song, W. Hwang, S. Park, H. Kim, Y. Ko and I. Han, J. Mater. Chem. A, 2014, 2, 11465 CAS.
- X. Zhang, R. Chen, Y. Liu and J. Hu, J. Mater. Chem. A, 2016, 4, 649 CAS.
- I. Das and G. De, Sci. Rep., 2015, 5, 18503 CrossRef PubMed.
- S. Li, J. Huang, M. Ge, C. Cao, S. Deng, S. Zhang, G. Chen, K. Zhang, S. Al-Deyab and Y. Lai, Adv. Mater. Interfaces, 2015, 2, 1500220 CrossRef.
- S. T. Yohe, Y. L. Colson and M. W. Grinstaff, J. Am. Chem. Soc., 2012, 134, 2016 CrossRef CAS PubMed.
- S. T. Yohe, J. A. Kopechek, T. M. Porter, Y. L. Colson and M. W. Grinstaff, Adv. Healthcare Mater., 2013, 2, 1204 CrossRef CAS PubMed.
- A. B. Tesler, P. Kim, S. Kolle, C. Howell, O. Ahanotu and J. Aizenberg, Nat. Commun., 2015, 6, 8649 CrossRef CAS PubMed.
- C. Xiao, L. Si and Y. Liu, J. Mater. Chem. A, 2016, 4, 8080 CAS.
- J. Huang, S. Li, M. Ge, L. Wang, T. Xing, G. Chen, X. Liu, S. Al-Deyab, K. Zhang, T. Chen and Y. Lai, J. Mater. Chem. A, 2015, 3, 2825 CAS.
- N. Wang, Y. Lu, D. Xiong, C. J. Carmalt and I. P. Parkin, J. Mater. Chem. A, 2016, 4, 4107 CAS.
- C. Xue, X. Guo, M. Zhang, J. Ma and S. Jia, J. Mater. Chem. A, 2015, 3, 21797 CAS.
- C. M. Grozea, M. Rabnawaz, G. Liu and G. Zhang, Polymer, 2015, 64, 153 CrossRef CAS.
- X. Li, D. Reinhoudt and M. Crego-Calama, Chem. Soc. Rev., 2007, 36, 1350 RSC.
- Y. Zhao, Z. Xu, X. Wang and T. Lin, Appl. Surf. Sci., 2013, 286, 364 CrossRef CAS.
- H. Bellanger, T. Darmanin, E. T. de Givenchy and F. Guittard, J. Mater. Chem. A, 2013, 1, 2896 CAS.
- X. Tian, L. Yi, X. Meng, K. Xu, T. Jiang and D. Lai, Appl. Surf. Sci., 2014, 307, 566 CrossRef CAS.
- G. Wang, S. Liu, S. Wei, Y. Liu, J. Lian and Q. Jiang, Sci. Rep., 2016, 6, 20933 CrossRef CAS PubMed.
- H. Lei, J. Xiao, L. Zheng, M. Xiong, Y. Zhu, J. Qian, Q. Zhuang and Z. Han, Polymer, 2016, 86, 22 CrossRef CAS.
- Y. Lai, J. Huang, Z. Cui, M. Ge, K. Zhang, Z. Chen and L. Chi, Small, 2016, 12, 2203 CrossRef CAS PubMed.
- K. Sasaki, M. Tenjimbayashi, K. Manabe and S. Shiratori, ACS Appl. Mater. Interfaces, 2016, 8, 651 CAS.
- S. Peng, D. Tian, X. Yang and W. Deng, ACS Appl. Mater. Interfaces, 2014, 6, 4831 CAS.
- Y. Lu, S. Sathasivam, J. Song, C. R. Crick and C. J. Carmalt, Science, 2015, 347, 1132 CrossRef CAS PubMed.
- M. Lee, G. Kwak and K. Yong, ACS Appl. Mater. Interfaces, 2011, 3, 3350 CAS.
- Y. Sun, M. Chen, S. Zhou, J. Hu and L. Wu, ACS Nano, 2015, 9, 12513 CrossRef CAS PubMed.
- F. Wan, D. Yang and E. Sacher, J. Mater. Chem. A, 2015, 3, 16953 CAS.
- H. Ogihara, J. Okagaki and T. Saji, Langmuir, 2011, 27, 9069 CrossRef CAS PubMed.
- Y. Zhang, J. Zhang and A. Wang, J. Mater. Chem. A, 2016, 4, 901 CAS.
- S. Ma, S. Zhan, Y. Jia and Q. Zhou, ACS Appl. Mater. Interfaces, 2015, 7, 21875 CAS.
- J. W. Costerton, P. S. Stewart and E. P. Greenberg, Science, 1999, 284, 1318 CrossRef CAS PubMed.
- M. Zhang, J. Zhao and J. Zheng, Soft Matter, 2014, 10, 7425 RSC.
- A. Besinis, T. De Peralta and R. D. Handy, Nanotoxicology, 2014, 8, 745 CrossRef CAS PubMed.
- M. Zhang, P. Wang, H. Sun and Z. Wang, ACS Appl. Mater. Interfaces, 2014, 6, 22108 CAS.
- M. Wu, B. Ma, T. Pan, S. Chen and J. Sun, Adv. Funct. Mater., 2016, 26, 569 CrossRef CAS.
- H. Kong and J. Jang, Langmuir, 2008, 24, 2051 CrossRef CAS PubMed.
- Z. Wang, J. Ou, Y. Wang, M. Xue, F. Wang, B. Pan, C. Li and W. Li, Surf. Coat. Technol., 2015, 280, 378 CrossRef CAS.
- J. Podporska-Carroll, E. Panaitescu, B. Quilty, L. Wang, L. Menon and S. C. Pillai, Appl. Catal., B, 2015, 176, 70 CrossRef.
- Y. Chen, M. Niu, S. Yuan and H. Teng, Appl. Surf. Sci., 2013, 264, 171 CrossRef CAS.
- Q. Zhang, H. Liu, X. Zhan, F. Chen, J. Yan and H. Tang, RSC Adv., 2015, 5, 77508 RSC.
- A. D. Fuchs and J. C. Tiller, Angew. Chem., Int. Ed., 2006, 45, 6759 CrossRef CAS PubMed.
- X. Qin, Y. Li, F. Zhou, L. Ren, Y. Zhao and X. Yuan, Appl. Surf. Sci., 2015, 328, 183 CrossRef CAS.
- U. Mizerska, W. Fortuniak, J. Chojnowski, R. Halasa, A. Konopacka and W. Werel, Eur. Polym. J., 2009, 45, 779 CrossRef CAS.
- Y. Lin, Q. Liu, L. Cheng, Y. Lei and A. Zhang, React. Funct. Polym., 2014, 85, 36 CrossRef CAS.
- J. Zhao, L. Ma, W. Millians, T. Wu and W. Ming, ACS Appl. Mater. Interfaces, 2016, 8, 8737 CAS.
- H. Diem, G. Matthias and R. A. Wagner, Amino Resins, 2000 DOI:10.1002/14356007.a02_115.
- B. Park, S. Lee and J. Roh, Eur. J. Wood Wood Prod., 2009, 67, 121 CrossRef CAS.
- S. Samarzija-Jovanovic, V. Jovanovic, S. Konstantinovic, G. Markovic and M. Marinovic-Cincovic, J. Therm. Anal. Calorim., 2011, 104, 1159 CrossRef CAS.
- G. Lu, D. Wu and R. Fu, React. Funct. Polym., 2007, 67, 355 CrossRef CAS.
- Q. Zhang, H. Liu, X. Chen, X. Zhan and F. Chen, J. Appl. Polym. Sci., 2015, 132, 41725 Search PubMed.
- C. Zhao, X. Li, L. Li, G. Cheng, X. Gong and J. Zheng, Langmuir, 2013, 29, 1517 CrossRef CAS PubMed.
- M. Im, H. Im, J. Lee, J. Yoon and Y. Choi, Soft Matter, 2010, 6, 1401 RSC.
- C. Chen, N. C. Beck-Tan, P. Dhurjati, T. K. van Dyk, R. A. LaRossa and S. L. Cooper, Biomacromolecules, 2000, 1, 473 CrossRef CAS PubMed.
- C. Xue, J. Chen, W. Yin, S. Jia and J. Ma, Appl. Surf. Sci., 2012, 258, 2468 CrossRef CAS.
- J. Ou, Z. Wang, F. Wang, M. Xue, W. Li and A. Amirfazli, Appl. Surf. Sci., 2016, 364, 81 CrossRef CAS.
Footnote |
† Electronic supplementary information (ESI) available: Video S1 displays the abrasion test on a PUF–FC2-coated glass slide. Videos S2 and S3 show the self-cleaning properties of four nanocomposite coatings and four copolymer coatings contaminated with copper(II) chloride dihydrate particles, respectively. Video S4 shows the self-cleaning properties of the nanocomposite surface contaminated with carbon powders. Video S5 displays liquid repellency to various liquids. See DOI: 10.1039/c6ta06481g |
|
This journal is © The Royal Society of Chemistry 2017 |
Click here to see how this site uses Cookies. View our privacy policy here.