DOI:
10.1039/C6SC00659K
(Edge Article)
Chem. Sci., 2016,
7, 4341-4346
Reversible gel–sol photoswitching with an overcrowded alkene-based bis-urea supergelator†
Received
12th February 2016
, Accepted 22nd March 2016
First published on 22nd March 2016
Abstract
A new type of low-molecular-weight gelator (LMWG), i.e. overcrowded alkene-based bis-ureas, can be switched effectively between cis and trans isomers using light as demonstrated by 1H NMR and UV-Vis spectroscopy. Gelation studies reveal that one of the synthesized trans compounds forms stable gels in aromatic hydrocarbon solvents down to a critical concentration of 0.4 mg mL−1. Transmission electron microscopy (TEM) shows that this gel consists of an entangled fibrous network. For the trans isomer of this LMWG intermolecular urea hydrogen bonding is observed in the solid state, whereas density functional theory (DFT) geometry optimization of the cis isomer indicates the possible formation of an intramolecular hydrogen bond. Irradiation of the gel triggers trans-to-cis isomerization and consequently, a gel–sol phase transition. This process can be fully reversed by altering the irradiation wavelength.
Introduction
The interest in self-assembled supramolecular materials that can respond or adapt to external stimuli is rapidly expanding.1 This is not surprising when one considers the potential applications of these materials in important areas, for example, drug delivery,2 self-healing,3 and chemosensing.4 In particular, physical gels formed by low-molecular-weight gelators (LMWG) are highly versatile and effective.5 The ease of disrupting the weak non-covalent interactions between LMWGs is what makes these gels responsive to a wide variety of chemical and physical stimuli.6
Bis-urea compounds are known to be excellent LWMGs that can gelate a wide range of solvents at low concentrations.7,8 Dissolution of bis-urea gels has been successfully triggered by anion coordination,9 and mechanical force,10 among others. The use of light as a non-invasive stimulus to control gelation properties has attracted much attention because it can be delivered with high spatiotemporal precision and does not produce waste.11 For this reason, van Esch and Feringa developed photoresponsive bis-urea LMWGs having azobenzene and dithienylethene core structures.12 However, for the azobenzene bis-urea derivatives the photochemical isomerization was blocked in the gel state and for the dithienylethenes, photoswitching had to be performed at elevated temperature (105–110 °C) to be able to induce a gel–sol transition. These studies accentuate that it is still a major challenge to develop light-responsive LMWGs of which (i) photoswitching is not constrained in the gel state and (ii) of which the gelation behaviour is sufficiently altered in the photogenerated state for undergoing a gel–sol transition.
Our group has developed various overcrowded alkene-based molecular switches,13 which have been applied to control, for example, magnetic and fluorophore interactions,14 anion binding,15 and aggregation16 by light. Yang and co-workers have recently functionalized a structurally related stiff stilbene switch with quadruple hydrogen bonding ureidopyrimidinone units.11e Using light, they were able to alternate between supramolecular polymer and dimer formation. Irradiation of a concentrated polymer gel, or alternatively the addition of an acid, resulted in gel dissolution. Herein, we present the first overcrowded-alkene based bis-urea LMWGs 1a–c (Scheme 1) that can undergo a trans-to-cis stilbene-type isomerization in response to UV irradiation.
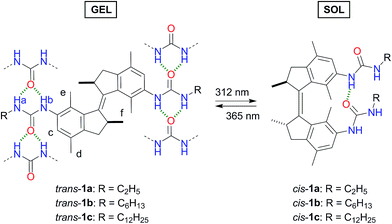 |
| Scheme 1 Photoisomerization behaviour and predicted urea hydrogen bonding pattern of trans and cis bis-urea LMWGs 1a–c. | |
We found that the hexyl derivative trans-1b forms stable gels at very low concentrations (down to 0.4 mg mL−1) in aromatic hydrocarbons. Gel dissolution can be triggered by irradiation and is most likely due to the obstruction of intermolecular hydrogen bonding, which is necessary to form fibres, in the photogenerated cis form. This process is fully reversible and hence, light can be used to switch between gel and solution (sol) phase on demand. The results presented here pave the way for further development of robust photo-switchable bis-urea gels to be used in diverse nanotechnology applications.
Results and discussion
Synthesis and isomerisation behaviour
The urea end groups are known to have a large influence on the gelation properties.7 Therefore, the alkyl chain length was varied (i.e. ethyl, hexyl, dodecyl) in bis-urea LMWGs trans-1a–c. Reaction of the previously reported bis-amine precursor trans-S1 (ref. 17) with the respective isocyanate in CH2Cl2 at room temperature (see Scheme S1 in the ESI for details†) afforded the desired products trans-1a, b in good yield (80–90%) after filtration. The isolated yield of 1c was lower (55%) due to its sticky and glassy nature. The resulting bis-ureas, obtained and used as racemates, were fairly soluble in DMSO. Hence, their photoresponsive behaviour was studied in this solvent by 1H NMR and UV-Vis spectroscopy.
Irradiation with 312 nm light of solutions of trans-1a–c, in DMSO-d6 induced 1H NMR spectral shifts that are indicative for formation of the cis isomer (Fig. 1 and S6–S8 in the ESI†).13,15 The cis
:
trans ratio at the photostationary state (PSS) was found to be 85
:
15 for each compound. Subsequent irradiation of the samples with 365 nm light led to full recovery of the 1H NMR spectrum of the starting trans isomer.
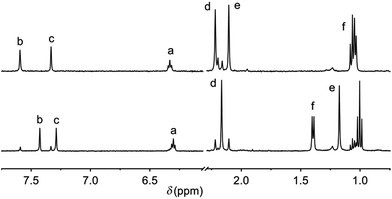 |
| Fig. 1 Aliphatic and aromatic region in the 1H NMR spectrum of 1a (1 mM in DMSO-d6) before (top) and after (bottom) 312 nm irradiation at 20 °C for 1 h. The top spectrum was recovered upon subsequent 365 nm irradiation for 1.5 h (see Fig. S6 in the ESI†). For the proton assignment, see Scheme 1. | |
By analogy with the trans-to-cis isomerisation of structurally related overcrowded alkenes,13,15 irradiation with 312 nm light of trans-1a–c resulted in a bathochromic shift of the UV-Vis absorption maxima (see Fig. 2 and S9–S11 in the ESI†). For bis-urea 1a for example, the absorption maxima at λ = 290 nm and 320 nm decreased and a new band appeared at λ = 360 nm. For all compounds, clear isosbestic points were observed which confirms that the photochemically induced trans-to-cis isomerization is a unimolecular process.18
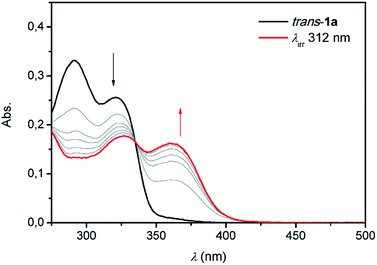 |
| Fig. 2 UV-Vis spectral changes upon irradiation with 312 nm light starting with trans-1a (black) at 1 × 10−5 M in DMSO and ending at the PSS (red). | |
Gelation solvent screening
The solubility and gelation properties of trans-1a–c were tested in a wide range of organic solvents (Table 1). A 10 mg mL−1 dispersion was repeatedly heated and sonicated to solubilise the material and the resulting solution was left to cool down to room temperature. In the case of poor solubility, more solvent was added to the sample. As can be observed in Table 1, the ethyl-appended trans-1a was insoluble in most of the selected solvents or it precipitated upon cooling of the solution. Nevertheless, an opaque gel was obtained within a few min of cooling from a CHCl3 solution as evidenced by the test tube inverting method (Fig. 3).
Table 1 Solubility and gelation properties of bis-ureas 1a–ca
Solvent |
1a
|
1b
|
1c
|
Abbreviations g: gel (between brackets the critical gelation concentration), i: insoluble, p: precipitate, g/p: gel-like precipitate, s: soluble at 20 °C at 10 mg mL−1.
Solubility in hot EtOH ≤ 2 mg mL−1.
Solubility in hot THF ≤ 5 mg mL−1.
|
DMSO |
p |
g/p |
g/p |
DMF |
p |
p |
p |
MeCN |
i |
i |
i |
EtOH |
pb |
pb |
p |
1,4-Dioxane |
i |
p |
p |
EtOAc |
i |
i |
i |
CHCl3 |
g (3 mg mL−1) |
s |
s |
THF |
i |
pc |
pc |
Xylene |
i |
g (0.4 mg mL−1) |
g (3 mg mL−1) |
Toluene |
i |
g (0.4 mg mL−1) |
g (4 mg mL−1) |
Dodecane |
i |
i |
i |
Hexane |
i |
i |
i |
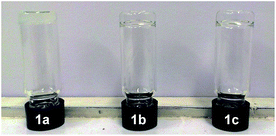 |
| Fig. 3 Gels obtained with trans-1a in CHCl3 and trans-1b, c in toluene at the critical gelation concentration (see Table 1). | |
The bis-urea LMWGs trans-1b and trans-1c with the longer alkyl substituents (i.e. hexyl and dodecyl, respectively) were generally better soluble than trans-1a (Table 1). Transparent gels formed within a couple of min of cooling a solution in the aromatic solvents xylene and toluene (Fig. 3). It is really remarkable that the hexyl derivative (trans-1b) forms toluene and xylene gels down to concentrations as low as 0.4 mg mL−1, comparable to that of the earlier classified “supergelators”.19
The morphology of the toluene gel obtained with the superior LMWG trans-1b was studied with transmission electron microscopy (TEM). Thread-like fibres were observed (Fig. 4), which fuse and intertwine to form an entangled network. The thicker fibres are bundles of thinner fibres and the smallest fibre diameter that could be measured is around 30 nm. Similar thread-like fibre formation has been reported for other bis-urea compounds.8a,b
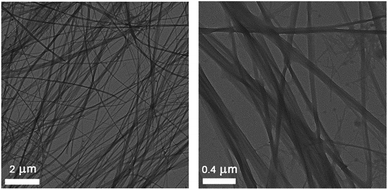 |
| Fig. 4 Electron micrographs of a toluene gel (0.5 mg mL−1) of trans-1b. | |
X-ray and DFT structural analysis
Recrystallization of trans-1b from MeOH afforded single crystals that were suitable for X-ray crystallography (Fig. 5A and B). Solid state structures of other bis-urea LMWGs most often exhibit two antiparallel hydrogen bonded urea moieties between adjacent molecules.8a,20 The resulting formation of long urea hydrogen bonded linear chains is considered to be the reason for their universal gelation ability. In the crystal structure of trans-1b, however, each urea unit is linked to a different neighbour giving rise to a two dimensional network.21 Interestingly, the neighbouring molecules alternate in chirality between (R,R) and (S,S). Most likely, this hydrogen bond arrangement in the solid state is not representative for that in the gel state since fibres with high aspect ratio are usually formed via linear self-assembly.20 Furthermore, urea hydrogen bonding N⋯O distances are in the range of 2.9–3.1 Å, which is similar to those observed before for other bis-urea gelators.20
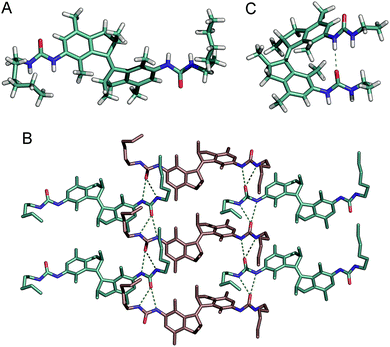 |
| Fig. 5 (A) X-ray molecular structure of trans-1b and (B) crystal packing showing the intermolecular hydrogen bond arrays. The (R,R)-enantiomer is shown in blue, the (S,S)-enantiomer in pink. Hydrogen atoms have been omitted in the packing for clarity. Selected hydrogen bond distances (Å): N⋯O: 3.053(2), 2.996(2), 2.940(2), 2.936(2). (C) DFT energy minimized structure [B3LYP 6-31G+(d,p), IEFPCM toluene] of (R,R)-cis-1a showing an intramolecular hydrogen bond. N⋯O distance: 3.216 Å. | |
The structure of the photogenerated cis isomer was optimized by DFT calculations on the B3LYP/6-31G+(d,p) level of theory using an IEFPCM, toluene solvation model (see the ESI for details†). The energy minimized structure (Fig. 5C) reveals the possible formation of an intramolecular urea hydrogen bond with an N⋯O distance of 3.2 Å. Another possibility that cannot be excluded is self-complementary dimerization as was previously observed for an ureidopyrimidinone-functionalized stiff stilbene derivate.11e Nevertheless, formation of intermolecular hydrogen bonding arrays as seen with the trans isomer, necessary to form fibres, is presumed to be unlikely for the cis isomer.
Photocontrol of gel–sol transitions
Structural analysis (vide supra) showed the formation of an intermolecular urea hydrogen bonded network for trans-1b and predicted intramolecular urea hydrogen bonding for the cis isomer. Based on these geometrical constraints, a poorer gelation ability was expected for cis-1b offering the possibility of photoinducing a gel–sol transition. To test this, a 0.5 mg mL−1 gel of trans-1b in toluene was transferred to a quartz cuvette and irradiated with 312 nm light for 15 min (Fig. 6A). Complete gel dissolution was observed and simultaneous recording of the UV-Vis spectrum (Fig. 6B), revealing a bathochromic shift, confirmed that trans-to-cis photoisomerization had taken place.22 Then, the obtained solution was irradiated for 15 min with 365 nm light followed by gentle heating.23 A transparent gel was again obtained upon cooling of the solution to room temperature proving the reversibility of this process.
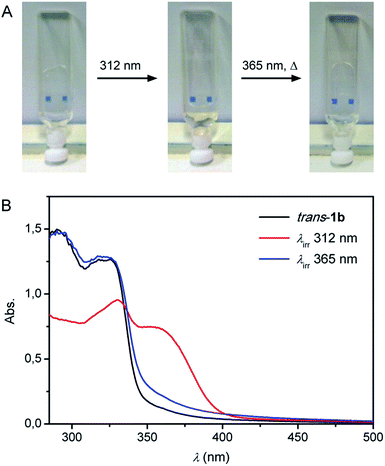 |
| Fig. 6 (A) 0.5 mg mL−1 toluene gel of trans-1b in a 1 mm quartz cuvette before and after 15 min irradiation with 312 nm light showing gel dissolution. The resulting solution was irradiated for 15 min with 365 nm light and gently heated to reform the gel upon slow cooling. (B) UV-Vis spectra measured during the gel–sol–gel switching cycle described above. | |
Whereas TEM analysis displayed fibre formation for the trans isomer (see Fig. 4), no fibres could be observed anymore by analysing the same sample that was obtained after 312 nm light irradiation (Fig. S17 in the ESI†). This result supports our hypothesis that fibre formation via intermolecular hydrogen urea bonding is blocked in the photogenerated cis state. On the other hand, when an identical sample was dried and analysed by FT-IR spectroscopy, absorptions characteristic for hydrogen bonded ureas were found (i.e. 3321 cm−1, 1644 cm−1 and 1558 cm−1 for the NH stretch, amide I and amide II vibrations, respectively, Fig. S18 in the ESI†).24 These IR absorptions do not differ significantly from those observed for trans-1b and suggest that the cis isomer is capable of urea hydrogen bonding.
Conclusions
In summary, we have described a new set of photoresponsive bis-urea LMWGs based on an overcrowded alkene switch. The hexyl-urea derivative trans-1b gelates aromatic hydrocarbons down to a critical concentration of 0.4 mg mL−1 and can therefore be categorized as “supergelator”. In the photogenerated cis state intermolecular hydrogen bonds are much less likely to form and hence, gel dissolution occurs upon the photoinduced trans-to-cis isomerization. Interestingly, this process can be reversed by light-irradiation to reobtain the gel phase. To the best of our knowledge, this work represents the most effective strategy towards reversible photoswitching of low-molecular-weight bis-urea gels. Therefore, this system is potentially suitable for functional applications such as self-healing and drug delivery. Current efforts in our lab are targeted at producing light-responsive hydrogels and exploitation of the unique chirality of these systems.
Experimental section
General methods and materials
Toluene and CH2Cl2 were dried by using an MBraun solvent purification system and solvents were degassed by purging with N2 for 30 min. The bis-amine precursor trans-S1 (see Scheme S1 in the ESI†) was prepared according to a procedure described in the literature.17 All other chemicals were commercial products and were used as received. Melting points (mp) were determined using a Büchi-B545 capillary melting point apparatus. 1H and 13C NMR spectra were recorded on Varian Mercury Plus-400 and Agilent 400-MR spectrometers at 298 K. Chemical shifts (δ) are denoted in parts per million (ppm) relative to DMSO-d6 (δH 2.50 ppm) or CDCl3 (δC 77.16 ppm). For 1H NMR spectroscopy, the splitting pattern of peaks is designated as: s (singlet), d (doublet), t (triplet), m (multiplet), or br (broad). High-resolution mass spectrometry (ESI-MS) was performed on a LTQ Orbitrap XL spectrometer with ESI ionization. UV-Vis absorption spectra were recorded on a Hewlet-Packard HP 8543 diode array with a Peltier heating/cooling element. IR spectra were measured on a Perkin Elmer Spectrum 400 FT-IR instrument and the bands are listed from 4000 to 600 cm−1 with intensities: s, m, w, br for broad and sh for shoulder. UV-Vis and 1H NMR irradiation experiments were carried out using a Spectroline model ENB-280C/FE lamp positioned at a distance of 3 cm from the sample of which the temperature was maintained at 20 °C.
General procedure for the synthesis of bis-urea LMWGs
The respective alkyl isocyanate (0.40 mmol) was added to the bis-amine precursor trans-S1 (0.20 mmol) in CH2Cl2 (4 mL) under a N2 atmosphere. The solution was stirred for 16 h and the white precipitate was filtered off and washed with CH2Cl2 to afford bis-urea LMWGs trans-1a–c as white solids.
Bis-ethylurea trans-1a
Yield: 90%. Mp 261 °C; FT-IR (ATR) νmax/cm−1 3327 (m, br), 2968 (m), 2928 (m), 2868 (w, sh), 1643 (s), 1547 (s), 1453 (m), 1249 (s), 1211 (m, sh), 1058 (w), 871 (w, br), 772 (w), 754 (w, sh); 1H NMR (400 MHz, DMSO-d6, assignment based on COSY) δH 7.58 (s, 2H; NH), 7.33 (s, 2H; ArH), 6.32 (t, J = 5.5 Hz, 2H; NH), 3.15–3.08 (m, 6H; CH, CH2), 2.79–2.72 (m, 2H; CH), 2.22 (s, 6H; ArCH3), 2.18 (d, J = 14.4 Hz, 2H; CH), 2.11 (s, 6H; ArCH3), 1.09–1.03 (m, 12H; CH3, ArCH3); too insoluble for 13C NMR measurement; HRMS (ESI) m/z: 489.3209 ([M + H]+, calcd for C30H41N4O2+: 489.3224).
Bis-hexylurea trans-1b
Yield: 82%. Mp 242 °C; FT-IR (ATR) νmax/cm−1 3325 (m, br), 2953 (m, sh), 2925 (m), 2856 (m), 1643 (s), 1555 (s), 1453 (m), 1343 (w), 1251 (s), 1212 (m, sh), 863 (w), 771 (w), 754 (w, sh); 1H NMR (400 MHz, DMSO-d6) δH 7.59 (s, 2H; NH), 7.35 (s, 2H; ArH), 6.35 (t, J = 5.6 Hz, 2H; NH), 3.11–3.05 (m, 6H; CH, CH2), 2.77–2.72 (m, 2H; CH), 2.22 (s, 6H; ArCH3), 2.18 (d, J = 14.4 Hz, 2H; CH), 2.10 (s, 6H; ArCH3), 1.47–1.39 (m, 4H; CH2), 1.33–1.26 (br, m, 12H; CH2), 1.04 (d, J = 6.4 Hz, 6H; CH3), 0.88 (t, J = 6.8 Hz, 6H; CH3); 13C NMR (100 MHz, CDCl3) δC 157.1, 142.8, 142.0, 141.1, 134.6, 132.8, 128.0, 126.5, 42.4, 40.6, 39.0, 31.7, 30.4, 26.7, 22.7, 19.2, 18.4, 18.1, 14.2; HRMS (ESI) m/z: 601.4459 ([M + H]+, calcd for C38H57N4O2+: 601.4476), 599.4306 ([M − H2 + H]+, calcd for C38H55N4O2+: 599.4320).
Bis-dodecylurea trans-1c
Yield: 55%. Mp 249 °C; FT-IR (ATR) νmax/cm−1 3332 (m, br), 2921 (s), 2851 (m), 1640 (s), 1555 (s), 1454 (m), 1371 (w), 1252 (s), 1212 (m, sh), 1072 (w), 865 (w, br), 773 (w), 754 (w, sh); 1H NMR (400 MHz, DMSO-d6) δH 7.57 (s, 2H; NH), 7.33 (s, 2H; ArH), 6.32 (t, J = 5.6 Hz, 2H; NH), 3.11–3.05 (m, 6H; CH, CH2), 2.78–2.72 (m, 2H; CH), 2.22 (s, 6H; ArCH3), 2.18 (d, J = 14.4 Hz, 2H; CH), 2.11 (s, 6H; ArCH3), 1.47–1.40 (br, m, 4H; CH2), 1.32–1.23 (br, m, 36H; CH2), 1.04 (d, J = 6.3 Hz, 6H; CH3), 0.85 (t, J = 6.4 Hz, 6H; CH3); 13C NMR (100 MHz, CDCl3) δC 157.1, 142.8, 141.9, 141.0, 134.6, 132.7, 127.9, 126.5, 42.4, 40.6, 39.0, 32.1, 30.4, 29.8 (4×), 29.5 (2×), 27.1, 22.8, 19.2, 18.4, 18.1, 14.3; HRMS (ESI) m/z: 769.6341 ([M + H]+, calcd for C50H81N4O2+: 769.6354), 767.6191 ([M − H2 + H]+, calcd for C50H79N4O2+: 767.6198).
Gelation experiments
The compound of interest (1 mg) was dispersed in 0.1 mL of solvent in a closed vial. The vial was repetitively sonicated and heated using a heating gun until the solid had dissolved. In the case of poor solubility, additional solvent was added to the mixture. The solution was allowed to cool to room temperature and gelation was considered to have occurred when there was no gravitational flow upon inverting the vial.
Gel–sol photoswitching experiments
A 0.5 mg mL−1 toluene gel of trans-1b was heated to give a solution and transferred to a 1 mm quartz cuvette. Gentle heating was followed by cooling to room temperature to reobtain the gel phase. Then the cuvette was placed upside down and irradiated with 312 nm light for 15 min using a Spectroline model ENB-280C/FE lamp positioned at a distance of 5 cm. The resulting solution was irradiated with 365 nm light for 15 min using the same lamp and slightly heated to reobtain the gel upon cooling to room temperature. After each step a UV-Vis absorption spectrum was recorded. In a separate experiment, an identically prepared 312 nm light irradiated sample was concentrated and an FT-IR (ATR) spectrum was measured.
Transmission electron microscopy
A toluene gel of trans-1b was prepared as described above and placed on a carbon coated copper grid. Images were recorded on a FEI Technai T20 microscope at 200 kV with a slow scan CCD camera.
Acknowledgements
This work was financially supported by The Netherlands Organization for Scientific Research (NWO-CW, Veni grant no. 722.014.006 to S. J. W.), the Ministry of Education, Culture and Science (Gravitation program 024.001.035), and the European Research Council (Advanced Investigator Grant, no. 227897 to B. L. F). We thank Dr Edwin Otten for X-ray crystal structure determination and Dr Tibor Kudernac for fruitful suggestions.
Notes and references
-
(a) R. Klajn, J. F. Stoddart and B. A. Grzybowski, Chem. Soc. Rev., 2010, 39, 2203–2237 RSC;
(b) M.-M. Russew and S. Hecht, Adv. Mater., 2010, 22, 3348–3360 CrossRef CAS PubMed;
(c) X. Yan, F. Wang, B. Zheng and F. Huang, Chem. Soc. Rev., 2012, 41, 6042–6065 RSC;
(d) E. A. Appel, J. del Barrio, X. Jun Loh and O. A. Scherman, Chem. Soc. Rev., 2012, 41, 6195–6214 RSC;
(e) X. Ma and H. Tian, Acc. Chem. Res., 2014, 47, 1971–1981 CrossRef CAS PubMed;
(f) Z. Qi and C. A. Schalley, Acc. Chem. Res., 2014, 47, 2222–2233 CrossRef CAS PubMed.
-
(a) S. Ganta, H. Devalapally, A. Shahiwala and M. Amiji, J. Controlled Release, 2008, 126, 187–204 CrossRef CAS PubMed;
(b) S. Mura, J. Nicolas and P. Couvreur, Nat. Mater., 2013, 12, 991–1003 CrossRef CAS PubMed.
-
(a) R. P. Wool, Soft Matter, 2008, 4, 400–418 RSC;
(b) M. D. Hager, P. Greil, C. Leyens, S. van der Zwaag and U. S. Schubert, Adv. Mater., 2010, 22, 5424–5430 CrossRef CAS PubMed;
(c) T. Aida, E. W. Meijer and S. I. Stupp, Science, 2012, 335, 813–817 CrossRef CAS PubMed.
- J. Hu and S. Liu, Macromolecules, 2010, 43, 8315–8330 CrossRef CAS.
-
(a) P. Terech and R. G. Weiss, Chem. Rev., 1997, 97, 3133–3159 CrossRef CAS PubMed;
(b) D. J. Abdallah and R. G. Weiss, Adv. Mater., 2000, 12, 1237–1247 CrossRef CAS;
(c) L. A. Estroff and A. D. Hamilton, Chem. Rev., 2004, 104, 1201–1218 CrossRef CAS PubMed;
(d) N. M. Sangeetha and U. Maitra, Chem. Soc. Rev., 2005, 34, 821–836 RSC;
(e) M. de Loos, B. L. Feringa and J. H. van Esch, Eur. J. Org. Chem., 2005, 3615–3631 CrossRef CAS;
(f) A. R. Hirst, B. Escuder, J. F. Miravet and D. K. Smith, Angew. Chem., Int. Ed., 2008, 47, 8002–8018 CrossRef CAS PubMed;
(g) X. Fu, Q. Zhang, G. Wu, W. Zhou, Q.-C. Wang and D.-H. Qu, Polym. Chem., 2014, 5, 6662–6666 RSC;
(h) R. Gu, J. Yao, X. Fu, W. Zhou and D.-H. Qu, Chem. Commun., 2015, 51, 5429–5431 RSC.
-
(a)
J. de Jong, B. L. Feringa and J. van Esch, Molecular Switches, ed. B. L. Feringa and W. R. Browne, Wiley-VCH, Weinheim, 2nd edn, 2011, vol. 2, ch. 14, pp. 517–561 Search PubMed;
(b) J. W. Steed, Chem. Commun., 2011, 47, 1379–1383 RSC;
(c) M. D. Segarra-Maset, V. J. Nebot, J. F. Miravet and B. Escuder, Chem. Soc. Rev., 2013, 42, 7086–7098 RSC.
- For reviews see:
(a) M. Yamanaka, J. Inclusion Phenom. Macrocyclic Chem., 2013, 77, 33–48 CrossRef CAS;
(b) L. S. Shimizu, S. R. Salpage and A. A. Korous, Acc. Chem. Res., 2014, 47, 2116–2127 CrossRef CAS PubMed.
- For selected examples see:
(a) J. van Esch, F. Schoonbeek, M. de Loos, H. Kooijman, A. L. Spek, R. M. Kellogg and B. L. Feringa, Chem.–Eur. J., 1999, 5, 937–950 CrossRef CAS;
(b) J. Brinksma, B. L. Feringa, R. M. Kellogg, R. Vreeker and J. van Esch, Langmuir, 2000, 16, 9249–9255 CrossRef CAS;
(c) J. F. Miravet and B. Escuder, Org. Lett., 2005, 7, 4791–4794 CrossRef CAS PubMed;
(d) F. Rodríguez-Llansola, D. Hermida-Merino, B. Nieto-Ortega, F. J. Ramírez, J. T. López Navarrete, J. Casado, I. W. Hamley, B. Escuder, W. Hayes and J. F. Miravet, Chem.–Eur. J., 2012, 18, 14725–14731 CrossRef PubMed;
(e) Z. Qi, P. Malo de Molina, W. Jiang, Q. Wang, K. Nowosinski, A. Schulz, M. Gradzielski and C. A. Schalley, Chem. Sci., 2012, 3, 2073–2082 RSC;
(f) L. Meazza, J. A. Foster, K. Fucke, P. Metrangolo, G. Resnati and J. W. Steed, Nat. Chem., 2012, 5, 42–47 CrossRef PubMed.
-
(a) H. Maeda, Chem.–Eur. J., 2008, 14, 11274–11282 CrossRef CAS PubMed;
(b) M.-O. M. Piepenbrock, G. O. Lloyd, N. Clarke and J. W. Steed, Chem. Rev., 2010, 110, 1960–2004 CrossRef CAS PubMed;
(c) J. W. Steed, Chem. Soc. Rev., 2010, 39, 3686–3699 RSC.
- J. T. van Herpt, M. C. A. Stuart, W. R. Browne and B. L. Feringa, Langmuir, 2013, 29, 8763–8767 CrossRef CAS PubMed.
- See for example:
(a) J. J. D. de Jong, L. N. Lucas, R. M. Kellogg, J. H. van Esch and B. L. Feringa, Science, 2004, 304, 278–281 CrossRef CAS PubMed;
(b) Z. Qiu, H. Yu, J. Li, Y. Wang and Y. Zhang, Chem. Commun., 2009, 3342–3344 RSC;
(c) S. Tamesue, Y. Takashima, H. Yamaguchi, S. Shinkai and A. Harada, Angew. Chem., Int. Ed., 2010, 49, 7461–7464 CrossRef CAS PubMed;
(d) Q. Zhang, D.-H. Qu, X. Ma and H. Tian, Chem. Commun., 2013, 49, 9800–9802 RSC;
(e) J.-F. Xu, Y.-Z. Chen, D. Wu, L.-Z. Wu, C.-H. Tung and Q.-Z. Yang, Angew. Chem., Int. Ed., 2013, 52, 9738–9742 CrossRef CAS PubMed;
(f) C.-T. Chen, C.-H. Chen and T.-G. Ong, J. Am. Chem. Soc., 2013, 135, 5294–5297 CrossRef CAS PubMed;
(g) Q. Li, G. Fuks, E. Moulin, M. Maaloum, M. Rawiso, I. Kulic, J. T. Foy and N. Giuseppone, Nat. Nanotechnol., 2015, 10, 161–165 CrossRef CAS PubMed;
(h) D.-H. Qu, Q.-C. Wang, Q.-W. Zhang, X. Ma and H. Tian, Chem. Rev., 2015, 115, 7543–7588 CrossRef CAS PubMed.
-
(a) S. van der Laan, B. L. Feringa, R. M. Kellogg and J. van Esch, Langmuir, 2002, 18, 7136–7140 CrossRef CAS;
(b) M. Akazawa, K. Uchida, J. J. D. de Jong, J. Areephong, M. Stuart, G. Caroli, W. R. Browne and B. L. Feringa, Org. Biomol. Chem., 2008, 6, 1544–1547 RSC.
- B. L. Feringa, J. Org. Chem., 2007, 72, 6635–6652 CrossRef CAS PubMed.
-
(a) J. Wang, A. Kulago, W. R. Browne and B. L. Feringa, J. Am. Chem. Soc., 2010, 132, 4191–4196 CrossRef CAS PubMed;
(b) J. Wang, L. Hou, W. R. Browne and B. L. Feringa, J. Am. Chem. Soc., 2011, 133, 8162–8164 CrossRef CAS PubMed.
-
(a) S. J. Wezenberg, M. Vlatković, J. C. M. Kistemaker and B. L. Feringa, J. Am. Chem. Soc., 2014, 136, 16784–16787 CrossRef CAS PubMed;
(b) M. Vlatković, B. L. Feringa and S. J. Wezenberg, Angew. Chem., Int. Ed., 2016, 55, 1001–1004 CrossRef PubMed.
-
(a) A. C. Coleman, J. M. Beierle, M. C. A. Stuart, B. Maciá, G. Caroli, J. T. Mika, D. J. van Dijken, J. Chen, W. R. Browne and B. L. Feringa, Nat. Nanotechnol., 2011, 6, 547–552 CrossRef CAS PubMed;
(b) D. J. van Dijken, J. Chen, M. C. A. Stuart, L. Hou and B. L. Feringa, J. Am. Chem. Soc., 2016, 138, 660–669 CrossRef CAS PubMed.
- M. Vlatković, L. Bernardi, E. Otten and B. L. Feringa, Chem. Commun., 2014, 50, 7773–7775 RSC.
- The discussed cis isomer is in a metastable state and can equilibrate to a more stable cis form. Half-lives (t1/2) at 20 °C were determined by UV-Vis spectroscopy as 70, 96 and 133 h for 1a–c, respectively (see Scheme S2 and Fig. S12–14 in the ESI for details†). The short timeframe in which the photo-switching experiments were carried out preclude significant thermal isomerization.
- O. Gronwald and S. Shinkai, Chem.–Eur. J., 2001, 7, 4328–4334 CrossRef CAS.
-
(a) M.-O. M. Piepenbrock, G. O. Lloyd, N. Clarke and J. W. Steed, Chem. Commun., 2008, 2644–2646 RSC;
(b) G. O. Lloyd, M.-O. M. Piepenbrock, J. A. Foster, N. Clarke and J. W. Steed, Soft Matter, 2012, 8, 204–216 RSC.
- A similar hydrogen bonding motif was recently reported for a mono-urea dimer: A. E. Hooper, S. R. Kennedy, C. D. Jones and J. W. Steed, Chem. Commun., 2016, 52, 198–201 RSC.
- No phase transition could be observed for a sample that was shielded with an optical filter (see Fig. S16 in the ESI†), which supports that the phase transition is induced by light.
- Gentle heating was applied to allow for restructuring of the gel network otherwise the sample remained a solution.
-
(a) Y. Mido, Spectrochim. Acta, Part A, 1972, 28, 1503–1518 CrossRef CAS;
(b) Y. Mido, Spectrochim. Acta, Part A, 1973, 29, 431–438 CrossRef CAS.
Footnote |
† Electronic supplementary information (ESI) available: UV-Vis, NMR and IR spectral data, schemes of synthesis and thermal isomerization, DFT geometry optimization and X-ray analysis. CCDC 1452254. For ESI and crystallographic data in CIF or other electronic format see DOI: 10.1039/c6sc00659k |
|
This journal is © The Royal Society of Chemistry 2016 |