DOI:
10.1039/C6RA21386C
(Paper)
RSC Adv., 2016,
6, 93349-93355
A new water-soluble heteronuclear PdII–AuI pincer complex as two-photon luminescent probe for biological Co2+ detection†
Received
25th August 2016
, Accepted 25th September 2016
First published on 26th September 2016
Abstract
The heterodinuclear NCN pincer complex K[(L)PdII(DPS)AuICl], 1, (HL = 5-methoxy-1,3-bis(1-methyl-1H-benzo[d]imidazol-2-yl)benzene, DPSH2 = 4,4′-dicyanamidodiphenyl sulfone) with two-photon induced luminescent properties was synthesized and investigated for biological Co2+ detection. Importantly, 1 can selectively recognize Co2+ in aqueous media in the presence of other abundant cellular cations (such as Na+, K+, Mg2+, and Ca2+), trace metal ions in organisms (such as Zn2+, Ag+, Fe3+, Fe2+, Ni2+, Mn2+, and Cu2+), prevalent toxic metal ions in the environment (such as Cd2+, Hg2+, and Cr3+), and amino acids, with high sensitivity. The fluorescence response of 1-Co2+ with respect to pH change was studied and the resulting demonstrated fluorescence enhancement was observed in the pH range of 4–10. The water-soluble probe 1 was successfully applied in the visualizing of the site of Co2+ accumulation as well as estimating of trace amounts of Co2+ ions in live HeLa cells by two-photon microscopy.
1. Introduction
In recent years, the expansion of highly sensitive and selective luminescent sensors for heavy- and transition-metal ions has involved remarkable interest due to their importance in chemistry, biology, and environmental science.1–5
Among the essential heavy metal ions in the human body, Co2+ is an important element for all multicellular organisms present in minute amounts as part of cobalamin and a few metalloproteins, and it plays important roles in several biological processes.6 Although cobalt is mildly toxic, unregulated exposure may cause detrimental effects including decreased cardiac output, cardiac and thyroid enlargements, heart disease, and elevated red blood cells accompanied by increased cells in bone marrow, increased blood volume and vasodilation, and flushing.7,8 Therefore, it is necessary to develop a novel method for the detection of these transition metals in biological and environmental samples in bioavailable forms.
Some organic molecules, silver and gold oxide nanoparticles were applied to detect Co2+,9–13 and a lanthanide show sensing effect to Zn2+, Cd2+ and Co2+ at the same time, but it is not a selective Co2+ sensor.10 That is, though lanthanide complex had been reported to detect many kinds of ions and molecules14–23 but designing fluorescent probes for Co is challenging due to the fluorescence quenching nature of paramagnetic character associated with Co2+ as well as microsecond to millisecond life times, characteristic and narrow emission bands, large stokes shifts. Therefore, selective and sensitive sensing Co2+ by luminescent metal complex is urgent and interesting. Moreover, in contrast to traditional one-photon microscopy (OPM) imaging techniques, two-photon microscopy (TPM) utilizes two photons of lower energy to obtain the excited state of a fluorophore and thus has advantages over OPM, including lower phototoxicity, better three-dimensional spatial localisation, deeper penetration depth, and lower self-absorption.24–29 Thus, the development of two-photon fluorescent probes for Co2+, probes that would have more practical applications than one-photon probes, would be of great value.
Recently, platinum(II) complexes system received great attention in the property of nonlinear absorption and two-photon induced luminescence.30–33 Although some cyctometalated platinum(II) complex showing two-photon (TP) imaging in live cells have been reported for the potential advantages including the long resident lifetime in biology system, the high two-photon induced luminescent intensity and the low cytotoxicity,34–36 the use of organic–metal palladium(II) complex in the field of TP probes studies has been mainly ignored.
Recently, we synthesized Au(I, III), trimethyltin(IV), mercury(II), nickel(II), and palladium(II) complexes of phenylcyanamide derivatives37–41 and our focus was on the coordination, biological chemistry and selective gas sensors. In continuation of our work, we now report, a new water-soluble heterodinuclear NCN pincer complex K[(L)PdII(DPS)AuICl], 1, (HL = 5-methoxy-1,3-bis(1-methyl-1H-benzo[d]imidazol-2-yl)benzene, DPSH2 = 4,4′-dicyanamidodiphenyl sulfone) has been reported for Co2+ detection. This complex takes strong two-photon-excitation luminescence and exhibits high sensitivity and selectivity toward Co2+ in live cells. As far as we are aware of, 1 is the first Co2+ sensor based on NCN pincer-Pd(II) complex containing phenylcyanamide derivative that can detect Co2+ in live cells.
2. Experimental
2.1. General procedures, materials and physical measurements
All materials were obtained from Sigma-Aldrich and used as received. 4,4′-Dicyanamidodiphenyl sulfone (DPSH2)42 and 5-hydroxy-1,3-bis(benzimidazol-2′-yl)benzene, HL′,43 were prepared according to the literature methods. Solutions of CuII, NaI, KI, MgII, CaII, MnII, and HgII were prepared from the chloride salts; solutions of CoII, NiII, ZnII, CdII, and CrIII were prepared from the acetate salts; solutions of AgI and FeIII were prepared from the nitrate salts; the FeII solution was prepared from ferrous ammonium sulphate and used immediately.
Fourier transform infrared spectra were recorded on a PerkinElmer Spectrum 400 (FT-IR/FT-NIR spectrometer). 1H and 13C {1H} nuclear magnetic resonance (NMR) spectra were recorded on a Bruker-400 MHz spectrometer at ambient temperature in DMSO-d6. Elemental analyses were carried out with an EA 3000 CHNS. Mass spectra were obtained on solutions in CH3OH-DMSO with an AB/Sciex QStar mass spectrometer with an ESI source in both positive or negative ion mode and time of flight detection. Fluorescence spectra were obtained using a Perkin-Elmer LS55 fluorescence spectrofluorometer at room temperature (25 °C). UV-visible (UV-vis) spectra were recorded on a JASCO 7580 UV-vis-NIR double-beam spectrophotometer using a quartz cell with a path length of 10 mm. Quantum yields of luminescence at room temperature (25 °C) were calculated according to literature procedures.44
2.2. Synthesis of ligands and complexes
2.2.1. Synthesis of ligand, (HL). A solution of 5-hydroxy-1,3-bis(benzimidazol-2′-yl)benzene (4.56 g, 14 mmol) in 25 mL of dry THF was treated with 27 mL of n-butyllithium (1.6 M in hexane, 43 mmol) at 0 °C under a nitrogen atmosphere. After 50 min, 6.67 g (47 mmol) of methyl iodide was added and the reaction mixture was allowed to reach ambient temperature. Stirring was continued for 34 h at room temperature. The solvent was evaporated under vacuum. After removal of the solvent, the residue was purified by column chromatography on silica gel with petroleum ether/EtOAc (4
:
1) as the eluent to give the pure product. Further recrystallisation from diethyl ether yielded 2 as white microcrystals (3.69 g, 71.6%). Mp: 92–96 °C. Anal. calc. (%) for C23H20N4O: C, 74.98; H, 5.47; N, 15.21; found (%): C, 74.91; H, 5.42; N, 15.16. IR: ν 3069 (w), 3033 (w), 2946 (m), 1581 (m), 1512 (w), 1436 (w), 1409 (s), 1371 (m), 1311 (s), 1275 (s), 1221 (w), 1165 (w), 1057 (m), 1018 (m), 995 (m), 870 (m), 848 (s), 735 (w), 679 (w) cm−1. 1H NMR (DMSO-d6): δ 7.84–7.79 (m, 2H, H-4′), 7.54 (t, 1H, H-1, 4J 1.4), 7.47–7.38 (m, 4H, H-5′ and H-6′), 7.35–7.29 (m, 4H, H-3,5 and H-7′), 3.83 (s, 3H, H-7), 3.39 (s, 6H, H-10′). 13C NMR (DMSO-d6): δ 161.14 (Ar), 153.78 (Ar), 142.76 (Ar), 136.36 (Ar), 132.05 (Ar), 122.82 (Ar), 122.33 (Ar), 121.87 (Ar), 120.08 (Ar), 117.45 (Ar), 110.25 (Ar), 56.13 (C-7), 32.11 (C-10′).
2.2.2. Synthesis of ligand 4,4′-dicyanamidodiphenyl sulfone (DPSH2). 4,4′-Dicyanamidodiphenyl sulfone (DPSH2) was prepared according to literature procedures (ESI†).42 Yield: 254 mg, 85%. Anal. calc. (%) for C14H10N4O2S: C, 56.37; H, 3.38; N, 18.78; found (%): C, 56.31; H, 3.32; N, 18.70. IR: ν 2945 (w), 2879 (m), 2229 (vs., NCN), 1615 (m), 1594 (s), 1415 (w), 1359 (m), 1316 (s), 1266 (m), 1245 (m), 1175 (s), 1145 (s), 1087 (m), 985 (m), 743 (w) cm−1. 1H NMR (DMSO-d6): δ 8.11 (s, 2H, H–NHCN), 6.99 (d, 4H, H-Ar (DPSH2), 3J 8.0), 6.79 (d, 4H, H-Ar (DPSH2), 3J 8.0). 13C NMR (DMSO-d6): δ 140.99 (Ar), 134.82 (Ar), 132.02 (Ar), 131.10 (Ar), 113.01 (NHCN).
2.2.3. Synthesis of K[(L)PdII(DPS)AuICl], 1. A mixture of the ligand HL (390 mg, 1 mmol) and Pd(OAc)2 (230 mg, 1 mmol) in glacial acetic acid (25 mL) was refluxed for 14 h. Then 4,4′-dicyanamidodiphenyl sulfone (DPSH2) (299 mg, 1 mmol) and KOH (112 mg, 2 mmol) in ethanol (10 mL) and HAuCl4 (340 mg, 1 mmol) in water (10 mL) was added and the reaction mixture was stirred at reflux temperature for 10 h to give a yellow precipitate. The precipitate was collected by filtration and washed with 10 mL of water, ethanol, and diethyl ether, respectively. The yellow powder was dried and dissolved in a minimum of acetonitrile (ca. 5 mL), flash precipitated in diethyl ether, collected by vacuum filtration and dried under vacuum (788.75 mg, 75.72% yield). Anal. calc. (%) for C37H27AuClKN8O3PdS: C, 42.66; H, 2.61; N, 10.76; found (%): C, 42.59; H, 2.56; N, 10.70. IR: ν 2964 (w), 2887 (m), 2118 (vs., NCN), 1605 (m), 1584 (s), 1435 (w), 1379 (m), 1316 (s), 1275 (m), 1259 (m), 1203 (w), 1173 (s), 1143 (s), 1061 (m), 983 (m), 730 (w) cm−1. TOF-MS: 1003 (M − K)−. 1H NMR (DMSO-d6): δ 7.93 (d, 2H, H-4′, 3J 8.0), 7.30 (t, 2H, H-6′, 3J 8.0), 7.03 (t, 2H, H5′, 3J 8.0), 6.97 (d, 2H, H-7′, 3J 8.0), 6.72 (s, 2H, H-3,5), 6.52 (t, 2H, Ar (DPSH2), 3J 8.0), 6.41 (t, 2H, Ar (DPSH2), 3J 8.0), 6.27 (t, 2H, Ar (DPSH2), 3J 8.0), 6.19 (t, 2H, Ar (DPSH2), 3J 8.0), 3.85 (s, 3H, H-7), 3.41 (s, 6H, H-10′). 13C NMR (DMSO-d6): δ 167.31 (Ar), 160.48 (Ar), 155.93 (Ar), 154.78 (Ar), 141.89 (Ar), 133.23 (Ar), 133.02 (Ar), 133.00 (Ar), 123.12 (Ar), 123.00 (Ar), 121.83 (Ar), 121.11 (Ar), 112.81 (Ar), 112.53 (Ar), 110.47 (Ar), 104.02 (NCN), 56.24 (C-7), 32.19 (C-10′).
2.3. Measurement of two-photon cross section
The two-photon cross section (δ) was determined by using femto second (fs) fluorescence measurement technique as described.45 Upon addition of Co2+ to 1 dissolved in 20 mM HEPES buffer (pH 7.0) at concentrations of 5.0 × 10−6 M and then the two-photon induced fluorescence intensity was measured at 700–1000 nm by using fluorescein (8.0 × 10−5 M, pH 11) as the reference, whose two-photon property has been well characterized in the literature.46 The intensities of the two-photon induced fluorescence spectra of the reference and sample emitted at the same excitation wavelength were determined. The two photon absorption (TPA) cross section was calculated by using δ2 = δr(ΦrCrIsns/ΦsCsIrnr): where I is the integrated fluorescence intensity, C is the concentration, n is the refractive index, and Φ is the quantum yield. Subscript “r” stands for reference samples, and “s” stands for samples.
2.4. Cell culture and imaging
HeLa human cervical carcinoma cells line were purchased from Pasture Institute, Tehran, Iran. The cells were maintained in Dulbecco's Modified Eagle Medium (DMEM) supplemented with 10% FBS, 100 IU mL−1 of penicillin, 100 μg mL−1 of streptomycin and 2 mM of Glutamax at 37 °C in a humidified incubator at 5% CO2. The adherent cultures were grown as monolayers and were passaged once in 4–5 days by trypsinizing them with 0.25% trypsin–EDTA.
2.5. Two-photon fluorescence microscopy
Two-photon fluorescence microscopy images of probe-labeled cells were obtained with spectral confocal and multiphoton microscopes (Leica TCS SP2) with a 100 (NA = 1.30 OIL) objective lens. The two-photon fluorescence microscopy images were obtained with a DMIRE2 Microscope (Leica) by exciting the probes with a mode-locked titanium-sapphire laser source (Coherent Chameleon, 90 MHz, 200 fs) set at wavelength 780 nm and output power 1230 mW, which corresponded to approximately 10 mW average power in the focal plane. To obtain images at 350–550 nm range, internal PMTs were used to collect the signals in an 8 bit unsigned 1024 × 1024 pixels at 400 Hz scan speed.
3. Results and discussion
3.1. Synthesis and characterization
The DPSH2 ligand was synthesized from 4,4′-diaminodiphenyl sulfone as previously reported.42 The synthesis of the ligand HL is shown in Scheme S1 (ESI†). 5-Hydroxy-1,3-bis-(benzimidazol-2′-yl)benzene (HL′) which was synthesized as previously reported43 was reacted with n-butyllithium and methyl iodide to give 5-methoxy-1,3-bis(N-methylbenzimidazol-2′-yl)benzene (HL) in ca., 60% yield. The purity and structure of the ligand was confirmed by elemental analysis, IR, 1H, and 13C NMR spectroscopy. Reaction of HL with Pd(OAC)2 in refluxing HOAc, followed by the addition of a solution of 4,4′-dicyanamidodiphenyl sulfone (DPSH2) and potassium hydroxide in ethanol afforded complex 1 (Fig. S1†). This step-by-step method allows for high-purity products in good yields. Complex 1 was fully characterized by IR, 1H NMR and 13C NMR spectroscopy (Fig. S2–S7, ESI†), MS spectrometry (Fig. S8, ESI†) and elemental analysis. The complex 1 is soluble in most of the organic solvents and water.
The 1H NMR spectra of ligand HL and complex 1 are consistent with the C2-symmetrical skeleton (Table S1†). Coordination of Pd to the deprotonated C1 carbon of the central phenyl ring of the pincer ligand is confirmed by the disappearance of the H1 signal and the ca. 0.6 ppm (Pd) upfield shifts of the H3/H5 signal. Likewise, the signals of the H5′, H6′ and H7′ protons are shifted to higher field in 1. By contrast, the H4′ proton experience a pronounced downfield shift from 7.82 ppm to 8.64 (Pd) ppm upon complexation. The DPSH2 protons are also shifted downfield in 1. Also, the NH proton of DPSH2 disappeared due to coordination of the cyanamide group to Pd(II) in complex 1.
Interpretation of the IR spectrum requires an understanding of the effect of resonance on the cyanamide group. The protonated cyanamide group the ligand DPSH2 shows an infrared stretch with the most nitrile character and typically has ν (NCN) ≈ 2229 cm−1. Upon deprotonation, two pairs of nonbonding electrons are delocalized in the three-atom π-system, generating two resonance forms (forms A and B in Scheme S2†). Deprotonation of the cyanamide groups shift ν (NCN) to low energies (≈2120 cm−1) but the magnitude of this effect will depend on the contribution of a given resonance form to the electronic structure of the cyanamide ligand, as influenced by the nature of the metal ion. Resonance form A should have an approaches that of neutral phenylcyanamides (2250 cm−1) while resonance form B should have ν (NCN) similar to that of organic carbodiimides (for R–N
C
N–R, ν (NCN) ranges from 2100 to 2150 cm−1).47 The infrared spectrum of 1 show ν (NCN) band at 2118 cm−1 is consistent with the presence of the deprotonated cyanamide as resonance form B (Scheme S2†).
Also, the ESI-TOF MS spectra of 1 showed peaks centered at m/z = 1003 corresponding to [(K[(L)PdII(DPS)AuICl])-K]−.
3.2. Metal sensing study
The binding activities of the sensor 1 with various metal ions were investigated by UV-visible absorption and fluorescence spectroscopy. The metal ions, including cations abundant in cells (Na+, K+, Mg2+, and Ca2+), metals present in trace quantities in organisms (Zn2+, Ag+, Fe3+, Fe2+, Ni2+, Mn2+, and Cu2+), and toxic metals prevalent in the environment (Cd2+, Hg2+, and Cr3+), no significant change was observed in luminescence; however, only the addition of Co2+ to 1 caused a major decrease in luminescence intensity (Fig. 1a). In contrast, with the addition of Co2+ to the probe 1, a remarkable decrease was observed in luminescence intensity, confirming the high selectivity of probe 1 for Co2+ over the other metal ions tested. Upon the addition of increasing concentrations of Co2+ (0 to 50 μM) to the probe 1 (10 μM), the luminescence of 1 was almost completely quenched (Fig. 1b).
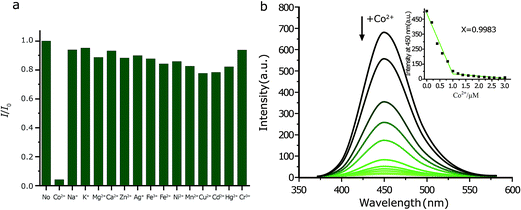 |
| Fig. 1 (a) Luminescence intensity of 1 (10 μM) with different metal ions in aqueous solution. (b) Emission spectra of 1 upon the addition of various concentrations of Co2+ (0–50 μM) in aqueous solution. Inset: plot of intensity versus Co2+ ion concentration. λex = 365 nm, λem = 450 nm. | |
From the photophysical properties it is observed that the binding of the sensor 1 to Co2+ by interactions with the oxygen atoms of –SO2 in DPSH2 ligand forms a 1– Co2+ complex. By using the mole-ratio method for determining luminescent intensity as a function of the number of equivalents of Co2+ ions added, the binding stoichiometry of 1 with Co2+ in the complex was 1
:
1 (Fig. 1b). The binding between Co2+ and the oxygen atoms of –SO2 in complex 1 would perturb the energy levels of the excited states, which results in a change of emission wavelength. The sensing ability depends on the availability of binding units which form the coordination complex with the metal ions. It also depends upon the geometry of the coordination complex formed after binding to metal (Fig. 2).
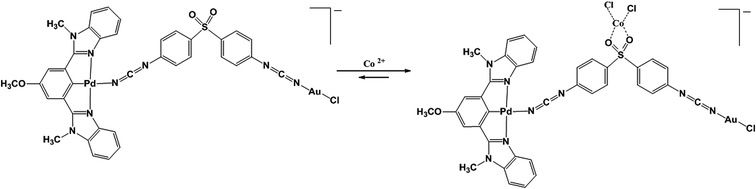 |
| Fig. 2 Proposed binding mechanism of Co2+ with sensor 1. | |
The apparent association constant (Ka) of the 1–Co2+ interaction was determined to be approximately (1.52 (±0.03) × 106) m−1 from the luminescence titration data following the modified Benesi–Hildebrand equation48,49 (Fig. S9, ESI†). Also, Ka was obtained from UV/Vis titration curve that was approximately (1.32 (±0.03) × 106). The comparison between two Ka shows the strong binding affinity of the Co2+ ion for 1 (Fig. S10, ESI†).
The sensitivity of 1 toward Co2+ was examined by using different concentrations of Co2+. There was a good linear correlation (R = 0.99763) between the relative luminescence intensity and the amount of Co2+ in the range from 2.2 × 10−7 to 4.6 × 10−6 M (Fig. 3). The regression equation was I = 561.3–121.3 × [Co2+] (mM), and the limit of detection (LOD) for the Co2+ ion with 1 was determined to be 3.42 × 10−8 M. The broad linear range and low detection limit meet the requirements for intracellular sensing and imaging of Co2+.
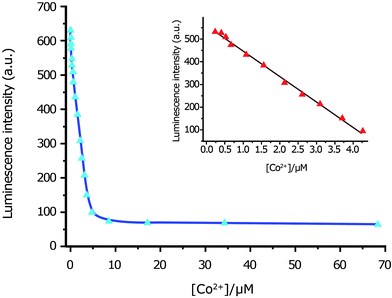 |
| Fig. 3 Luminescence response of 1 to different concentrations of Co2+. Inset: a linear correlation between emission intensity and concentrations of Co2+. λex = 365 nm, λem = 450 nm. | |
To confirm the formation of the 1 + Co2+ complex 1 was reacted with CoCl2, and the product was isolated and characterized by using ESI-MS. The signal of [1 + Co + Cl – K]− was observed in the ESI-MS spectrum, where the measured molecular weights were consistent with the expected values (Fig. S11, ESI†).
To examine the reversibility of the 1–Co2+ interaction, an aqueous solution of EDTA disodium (300 μM; EDTA = ethylene diamine tetraacetic acid) was added to the complex solution of 1 (10 μM) and Co2+ (50 μM) in a HEPES buffer solution (10 μM, pH 7.4; HEPES = 4-(2-hydroxyethyl)-1-piperazineethanesulfonic acid). The luminescence signal (λmax = 450 nm) was almost completely recovered (to 93.2% of the original; Fig. 4), demonstrating that the binding is chemically reversible.
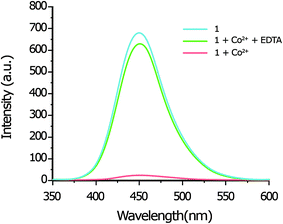 |
| Fig. 4 The emission spectra of 1 (10 μM), 1 (10 μM) + Co2+ (50 μM), and 1 (10 μM) + Co2+ (50 μM) + EDTA (300 μM) in aqueous solution (HEPES buffer solution, pH 7.4). | |
In addition, different cobalt salts including CoCl2, Co(NO3)2, Co(OAc)2, and CoSO4 gave rise to almost the same fluorescence quenching of the probe (Fig. S12, ESI†), indicating a negligible effect of the counter anions on the recognition ability and photophysical properties of 1.
The influence of other metal ions, such as Na+, K+, Mg2+, Ca2+, Zn2+, Ag+, Fe3+, Fe2+, Ni2+, Mn2+, Cu2+, Cd2+, Hg2+, and Cr3+, on the sensing of Co2+ was also determined (Fig. 5). The emission intensity of 1 in the presence of other metal ions (20 μM) only slightly decreases. Upon the addition of Co2+ (2 μM) to the incubated 1
:
20 mixture of 1 and other metal ions, the luminescence is instantly and almost completely quenched. The results indicate that the binding of Co2+ ion to 1 is much stronger than that of other metal ions and that Co2+ can replace the other metal ions if they bind to 1. As a result, the interference from those metal ions could be neglected, and 1 can be used as a highly selective luminescence sensor for Co2+ ions with a low detection limit.
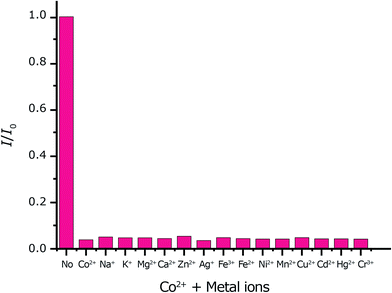 |
| Fig. 5 Luminescence intensity of 1 (10 μM) upon the addition of Co2+ (50 μM) in the presence of background metal ions (400 μM) in aqueous solution (HEPES buffer solution, pH 7.4). λex = 365 nm, λem = 450 nm. | |
Fig. 6a exhibits the two-photon-excitation spectrum of 1 from 700 to 1000 nm. With reference to rhodamine B, the largest two-photon absorption (TPA) cross section, δ, of approximately 400 Gçppert-Mayer (GM) units (1 GM = 1 × 10−50 cm4 per s per photon) was measured at 850 nm, a value that is hundreds of times larger than that of commercially available dyes for two-photon excitation (TPE) microscopy (0.16 GM for 4,6-diamino-2-phenyl indole (DAPI) and 1 GM for Cascade Bluet fluorescent dyes46) and also much higher than that of some recently reported two-photon bioavailable molecular probes.50,51 Moreover, the emission spectra of 1 and 1 + Co2+ indicated that the Co2+ ion can be detected by using the two-photon excitation window at 850 nm (Fig. 6b). A two-photon process was confirmed by a power-dependence experiment: a log–log linear relationship between the emission intensity and the incident power, with a slope of 1.9887, was recorded (Fig. S13, ESI†).
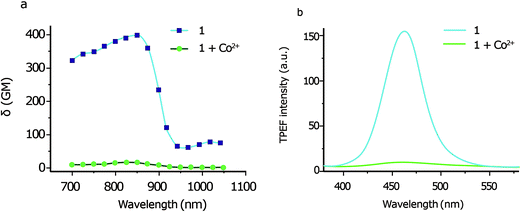 |
| Fig. 6 (a) Two-photon-excitation spectra of 1 at different excitation wavelengths from 700 to 1000 nm. (b) The two-photon fluorescence spectra with excitation by 850 nm fs laser pulses in the absence and presence of Co2+. | |
High photostability becomes very important for probes in bioimaging applications and is beneficial for long-term observations. 1 was observed to exhibit greater photostability than rhodamine B under the same excitation conditions (Fig. S14, ESI†).
For biological applications of a chemosensor, the sensor should operate in a wide pH range. To evaluate the effects of pH on the stability of 1, the emission spectra of 1 were measured at different pH conditions ranging from 4 to 10 with and without Co2+. The luminescence “on–off” switch of 1 can be operated by cobalt-ion binding in solutions with pH levels of 4 to 10 (Fig. S15, ESI†).
Because amino acids in a biological system are capable of interacting with numerous metal cations, several typical amines were also examined as potential interfering factors. In this study, no obvious signal changes were observed after the addition of amines at concentrations identical to that of the Co2+ metal ions (Fig. 7). The experiment confirmed that the presence of amino acids results in almost no interference with the sensitive detection of Co2+ by 1 in the luminescent response.
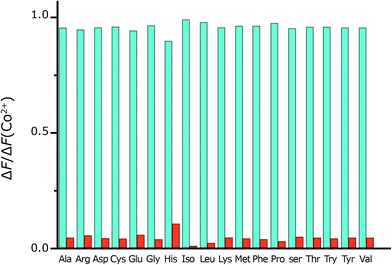 |
| Fig. 7 The ratio between the luminescence changes of 1 (10 μM) upon the addition of various amino acids (50 μM) and CoCl2 (50 μM) in aqueous solution. Left-hand bars represent the luminescent responses toward amino acids; right-hand bars represent the subsequent addition of CoCl2 (50 μM) to the aforementioned solutions. | |
To confirm the utility of this probe, the capability of 1 was tested to detect the Co2+ in live cells (Fig. 8). The TPM image of HeLa cells labeled with 1 (10 μM) for 20 min at 37 °C showed strong fluorescence (Fig. 8a). The TPEF (two-photon excited fluorescence) displayed a very weak fluorescence signal when the cells were treated with 50 μM of Co2+ for 20 min (Fig. 8b). This result indicates that 1 is capable of detecting Co2+ in live cells.
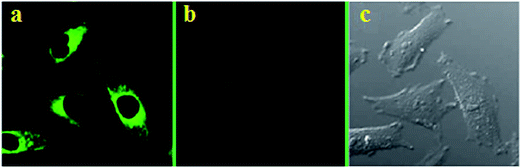 |
| Fig. 8 TPM image of HeLa cells labeled with 1 (10 μM) before (a) and after (b) addition of Co2+ (50 μM) for 20 min. (c) Bright-field image of cells. The TPEF was collected at 350–550 nm upon excitation at 365 nm with fs pulse. Scale bars, 30 μm. | |
4. Conclusions
The new heterodinuclear PdII, AuI Pincer Complex, K[(L)PdII(DPS)AuICl], 1, (HL = 5-methoxy-1,3-bis(1-methyl-1H-benzo[d]imidazol-2-yl)benzene, DPSH2 = 4,4′-dicyanamidodiphenyl sulfone) have been designed and synthesized that used as two-photon luminescent probe for Co2+ ions with high sensitivity and selectivity. The two-photon microscopy experiments indicated that 1 can be used for bioimaging of Co2+. Due to the nature of its large two-photon absorption cross-section, its good water solubility and membrane permeability, 1 used as a sensing probe for the detection of Co2+ in living cells. The design strategy and significant photophysical properties of complex 1 will be of great benefit to biomedical researchers for studying the effects of Co2+ in biological systems.
Acknowledgements
We acknowledge general support from the Department of Chemistry, Isfahan University of Technology (IUT).
References
- A. P. de Silva, H. Q. N. Gunaratne, T. Gunnlaugsson, A. J. M. Huxley, C. P. McCoy, J. T. Rademacher and T. E. Rice, Chem. Rev., 1997, 97, 1515 CrossRef CAS PubMed.
- E. L. Que, D. W. Domaille and C. J. Chang, Chem. Rev., 2008, 108, 1517 CrossRef CAS PubMed.
- Q. Zhao, F. Y. Li and C. H. Huang, Chem. Soc. Rev., 2010, 39, 3007 RSC.
- V. Fernndez-Moreira, F. L. Thorp-Greenwood and M. P. Coogan, Chem. Commun., 2010, 46, 186 RSC.
- Y. M. Yang, Q. Zhao, W. Feng and F. Y. Li, Chem. Rev., 2013, 113, 192 CrossRef CAS PubMed.
- S. Okamoto and L. D. Eltis, Metallomics, 2011, 3, 963 RSC.
- D. G. Barceloux, Clin. Toxicol., 1999, 37, 201 CAS.
- A. I. Selden, C. Norberg, C. Karlson-Stiber and E. Hellstrom-Lindberg, Environ. Toxicol. Pharmacol., 2007, 23, 129 CrossRef CAS PubMed.
- H. Y. Au-Yeung, E. J. New and C. J. Chang, Chem. Commun., 2012, 48, 5268 RSC.
- E. J. Song, J. Kang, G. R. You, G. J. Park, Y. Kim and S.-J. Kim, et al., Dalton Trans., 2013, 42, 15514 RSC.
- U. B. Patel, V. N. Mehta, M. A. Kumar and S. K. Kailasa, Res. Chem. Intermed., 2013, 39, 771 CrossRef CAS.
- K. D. Bhatt, H. S. Gupte, B. A. Makwana, D. J. Vyas, D. Maity and V. K. Jain, J. Fluoresc., 2012, 22, 1493 CrossRef CAS PubMed.
- M. R. Awual, M. Ismael and T. Yaita, Sens. Actuators, B, 2014, 191, 9 CrossRef CAS.
- Y. Kitamura, T. Ihara, Y. Tsujimura, Y. Osawa and A. Jyo, Nucleic Acids Symp. Ser., 2006, 2004, 105 CrossRef PubMed.
- K. L. Wong, G. L. Law, Y. Y. Yang and W. T. Wong, Adv. Mater., 2006, 18, 1051 CrossRef CAS.
- M. L. Cable, D. J. Levine, J. P. Kirby, H. B. Gray and A. Ponce, Luminescent lanthanidesensors, in Advances in Inorganic Chemistry, Inorganic Photochemistry, ed. R. VanEldik and G. Stochel, 2011, vol. 63, p. 1 Search PubMed.
- S. Dang, E. Ma, Z.-M. Sun and H. Zhang, J. Mater. Chem., 2012, 22, 1692 Search PubMed.
- D. Tian, Y. Li, R.-Y. Chen, Z. Chang, G.-Y. Wang and X.-H. Bu, J. Mater. Chem. A, 2014, 2, 1465 CAS.
- H. Liu, H. Wang, T. Chu, M. Yu and Y. Yang, J. Mater. Chem. C, 2014, 2, 8683 RSC.
- C. F. Chow, M. H. W. Lam and W. Y. Wong, Anal. Chem., 2013, 85, 8246 CrossRef CAS PubMed.
- Y. Zhang, Y. Tang, X. Liu, L. Zhang and Y. Lv, Sens. Actuators, B, 2013, 185, 363 CrossRef CAS.
- S. Park and S.-Y. Lee, Sens. Actuators, B, 2014, 202, 690 CrossRef CAS.
- A. Motorina, O. Tananaiko, I. Kozytska, V. Raks, R. Badia and M. E. Diaz-Garcia, et al., Sens. Actuators, B, 2014, 200, 198 CrossRef CAS.
- W. Denk, J. H. Strickler and W. W. Webb, Science, 1990, 248, 73 CAS.
- H. M. Kim and B. R. Cho, Acc. Chem. Res., 2009, 42, 863 CrossRef CAS PubMed.
- X. Wang, D. M. Nguyen, C. O. Yanez, L. Rodriguez, H. Y. Ahn, M. V. Bondar and K. D. Belfield, J. Am. Chem. Soc., 2010, 132, 12237 CrossRef CAS PubMed.
- E. W. Seo, J. H. Han, C. H. Heo, J. H. Shin, H. M. Kim and B. R. Cho, Chem.–Eur. J., 2012, 18, 12388 CrossRef CAS PubMed.
- G. Wang, X. H. Zhang, J. L. Geng, K. Li, D. Ding, K. Y. Pu, L. P. Cai, Y. H. Lai and B. Liu, Chem.–Eur. J., 2012, 18, 9705 CrossRef CAS PubMed.
- F. Liu, T. Wu, J. F. Cao, S. Cui, Z. G. Yang, X. X. Qiang, S. G. Sun, F. L. Song, J. L. Fan, J. Y. Wang and X. J. Peng, Chem.–Eur. J., 2013, 19, 1548 CrossRef CAS PubMed.
- Z. D. Yang, J. K. Feng and A. M. Ren, Inorg. Chem., 2008, 47, 10841 CrossRef CAS PubMed.
- P. Shao, Y. Li, J. Yi, T. M. Pritchett and W. Sun, Inorg. Chem., 2010, 49, 4507 CrossRef CAS PubMed.
- D. C. Flynn, G. Ramakrishna, H. B. Yang, B. H. Northrop, P. J. Stang and T. Goodson, J. Am. Chem. Soc., 2010, 132, 1348 CrossRef CAS PubMed.
- C. H. Tao, H. Yang, N. Zhu, V. W. W. Yam and S. J. Xu, Organometallics, 2008, 27, 5453 CrossRef CAS.
- C. K. Koo, K. L. Wong, C. W. Y. Man, Y. W. Lam, L. K. Y. So, H. L. Tam, S. W. Tsao, K. W. Cheah, K. C. Lau, Y. Y. Yang, J. C. Chen and M. H. W. Lam, Inorg. Chem., 2009, 48, 872 CrossRef CAS PubMed.
- C. K. Koo, K. L. Wong, C. W. Y. Man, H. L. Tam, S. W. Tsao, K. W. Cheah and M. H. W. Lam, Inorg. Chem., 2009, 48, 7501 CrossRef CAS PubMed.
- C. K. Koo, L. K. Y. So, K. L. Wong, Y. M. Ho, Y. W. Lam, M. H. W. Lam, K. W. Cheah, C. C. W. Cheng and W. M. Kwok, Chem.–Eur. J., 2010, 16, 3942 CrossRef CAS PubMed.
- L. Tabrizi and H. Chiniforoshan, Dalton Trans., 2015, 44, 2488 RSC.
- H. Chiniforoshan, N. Pourrahim, L. Tabrizi, H. Tavakol, M. R. Sabzalian and B. Notash, Inorg. Chim. Acta, 2014, 416, 85 CrossRef CAS.
- L. Tabrizi, P. McArdle, A. Erxleben and H. Chiniforoshan, Inorg. Chim. Acta, 2015, 438, 94 CrossRef CAS.
- M. Jazestani, H. Chiniforoshan, L. Tabrizi, P. McArdle and B. Notash, Inorg. Chim. Acta, 2016, 450, 402 CrossRef CAS.
- L. Tabrizi and H. Chiniforoshan, Invest. New Drugs, 2016 DOI:10.1007/s10637-016-0393-0.
- M. A. S. Aquino, C. A. White, C. Bensimon, J. E. Greedan and R. J. Crutchley, Can. J. Chem., 1996, 74, 2201 CrossRef CAS.
- P. Froidevaux, J. M. Harrowfield and A. N. Sobolev, Inorg. Chem., 2000, 39, 4678 CrossRef CAS PubMed.
- J. N. Demas and G. A. Crosby, J. Phys. Chem., 1971, 75, 991 CrossRef.
- S. K. Lee, W. J. Yang, J. J. Choi, C. H. Kim, S. J. Jeon and B. R. Cho, Org. Lett., 2005, 7, 323 CrossRef CAS PubMed.
- C. Xu and W. W. Webb, J. Opt. Soc. Am. B, 1996, 13, 481 CrossRef CAS.
- R. J. Crutchley, Coord. Chem. Rev., 2001, 219–221, 125 CrossRef CAS.
- H. A. Benesi and H. H. Hildebrand, J. Am. Chem. Soc., 1949, 71, 2703 CrossRef CAS.
- A. Thakur, S. Sardar and S. Ghosh, Inorg. Chem., 2011, 50, 7066 CrossRef CAS PubMed.
- M. X. Yu, M. Shi, Z. G. Chen, F. Y. Li, X. X. Li, Y. H. Gao, J. Xu, H. Yang, Z. G. Zhou, T. Yi and C. H. Huang, Chem.–Eur. J., 2008, 14, 6892 CrossRef CAS PubMed.
- L. Liu, X. Dong, Y. Xiao, W. Lian and Z. Liu, Analyst, 2011, 136, 2139 RSC.
Footnote |
† Electronic supplementary information (ESI) available. See DOI: 10.1039/c6ra21386c |
|
This journal is © The Royal Society of Chemistry 2016 |
Click here to see how this site uses Cookies. View our privacy policy here.