DOI:
10.1039/C6RA21347B
(Paper)
RSC Adv., 2016,
6, 94531-94538
Correlations between fluorescence emission and base stacks of nucleic acid G-quadruplexes†
Received
25th August 2016
, Accepted 22nd September 2016
First published on 23rd September 2016
Abstract
The aromatic 5/6 ring overlaps at the interface of 5′-5′ stacked dimers formed by two parallel G-quadruplexes have been demonstrated to produce a characteristic 385 nm florescence emission band excited at 260 nm. To further investigate the effect of base stacks within nucleic acid G-quadruplexes on the generation of the particular fluorescence emission bands, a series of G-rich DNA sequences are selected. They have been previously studied by NMR forming G-quadruplexes in which bases have defined glycosidic bond angles (GBA) and stack patterns; therefore, the G-quadruplexes give characteristic circular dichroism spectra termed as parallel or antiparallel structure. Herein, we measure their fluorescence emissions excited at 260 nm. Our results show that parallel G-quadruplexes can produce characteristic fluorescence emissions particularly when they stack at the interface in 5′-5′ manner regardless of aromatic 5/6 ring overlaps. Stacked guanines inside the G-quadruplex cores that present in anti GBA can also have a subtle influence on fluorescence emissions, which result in tailing peaks over 390 nm. Furthermore, the simultaneous presence of two remarkable emission bands around 330 nm and 385 nm is found to be related to the overall anti guanines stacking. These findings show potential use for providing topological information of G-quadruplexes based on characteristic fluorescence emissions.
Introduction
Guanine-rich (G-rich) nucleic acid sequences are widely present in functional domains of human genes, for example telomeres and oncogene promoters. They can form non-canonical four-stranded structures, namely, G-quadruplexes.1–4 G-quadruplex formations are not only biologically relevant but also potential targets for cancer treatments and inhibitors of HIV integrase.5–9 In addition, the G-quadruplexes have been applied as building blocks for the construction of nanoscale materials.10,11
In the structure of G-quadruplex (Fig. 1), four guanines arrange in a rotational way to form a G-quartet square. At the edge of G-quartet guanines are connected by Hoogsteen hydrogen bonds. Aligned guanines adopt either syn or anti conformation according to the definition state of the glycosidic bond angle (GBA, Fig. 1b). The G-quartet has a large π-surface that can stack upon each other to construct a tetrad core. The linkages between G-repeats hanging outside the G-quadruplex core form different types of loops (propeller, diagnostic, and lateral). In addition, monovalent cations between G-quartets reduce the repulsion of oxygen atoms and stabilize G-quadruplexes.
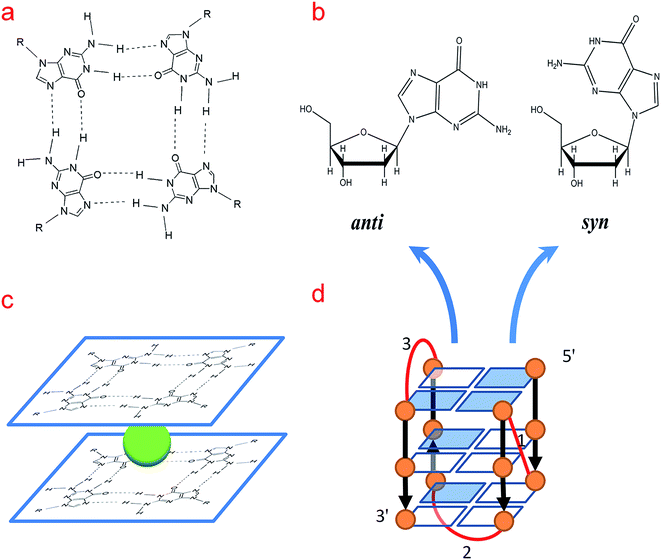 |
| Fig. 1 (a) A G-quartet formed by four guanines linked by Hoogsteen hydrogen bonds. (b) Chemical structures of guanines with syn and anti glycosidic bond angles. (c) Stacked G-quartets with a monovalent cation between them. (d) Schematic of a representative G-quadruplex formed by Hb-G3 with sequence listed in Table 1 wherein the red line 1 represents a propeller loop. Red line 2 represents a diagnostic loop and red line 3 represents a lateral loop. The nattier blue quadrangles stand for syn GBA guanines and white quadrangles stand for anti GBA guanines. | |
Both the loop types and the various glycosidic bond angles are important structural factors contributing to the diversity of topological G-quadruplexes.12 Recently, Webba da Silva et al. systematically investigated stacking types of bases. They propose four possibilities of base stacking interactions in a quadruplex stem: anti–anti, anti–syn, syn–anti, syn–syn.13,14 Phan et al. studied stacking geometries of guanines in the G-quadruplex core and stacking at the interfaces in higher-ordered quadruplexes by energy calculations.15 Their results demonstrated that 5′-5′ and 3′-3′ stacking for parallel G-quadruplexes have a different stacking pattern and 5′-5′ stacking is more energetically favorable. Furthermore, it has been revealed that an intrinsic property of base stacking is aromatic overlaps, which should feature in spectroscopic analysis and provide important structural information related to base stacking.16
The original fluorescence quantum yield of DNA sequence is fairly low.17,18 The detection of target DNA usually relies on the help from attached fluorescent dyes.19 Furthermore, by probing the fluorescence quenching or fluorescence resonance energy transfer (FRET) in the ensemble of fluorescent beacons, it is possible to approach the G-quadruplex formation at single-molecule level.20–22 However, recent reports show that DNA G-quadruplexes have relatively higher fluorescence quantum yields than the dissociative single-stranded oligonucleotides.23 The excimer dynamics of oligonucleotides are sensitive to the structure adopted, particularly when it contains π–π base stacking and base pairing.24 Phan et al. show an intensively characteristic 385 nm emission band excited at 260 nm produced by the stacked interface of two parallel quadruplexes.24 They proposed that aromatic 5/6-ring overlap is a factor resulting in the production of 385 nm emissions. This band differs from the 330 nm emission produced by interlocked G-quadruplex dimers or other aromatic stacking modes. These results attract our attention to further investigate correlations between fluorescence emission and base stacking of G-quadruplexes.
In the present study, several well-known DNA sequences were selected (Table 1), which had well-defined base stacking patterns and GBAs. The sequences are classified into four categories according to their potential conformation: (1) 5′-5′ stacked dimers formed by two parallel subunits in which all bases adopt an anti conformation (the sequences include T39695, T30177 and NG16);25,26 (2) two parallel monomers and an interlocked dimer (c-kit87up, c-myc and 93del). The c-kit87up and c-myc from c-kit oncogene and c-myc promoters form parallel monomers, respectively.27 The last guanine in the structure of c-myc presents a syn conformation. 93del is a parallel interlocked dimer in which G1 of each subunit adopts a syn conformation, inserting into another subunit to form a G-quartet at the interface;28 (3) 3 + 1 type of monomolecular G-quadruplexes, in which one strand aligns differently from the other three strands in orientation and bases adopt mixed anti and syn GBAs (the sequences include Ht-1 & Ht-2 and Hb-G3);29 (4) antiparallel bimolecular G-quadruplexes in which bases adopt mixed anti and syn GBAs (the sequence includes G3G3 and G4G4).30,31 By monitoring the fluorescence emissions of G-quadruplexes formed by these sequences in vitro, significant information on correlation between fluorescent signals and base stacking was obtained. Based on our FL results and combined with mass spectrometry (MS) and circular dichroism (CD) methods, the stacking status of a higher-ordered G-quadruplexes was proposed.
Table 1 Sequences used in this work
Name |
Sequence (5′-3′) |
Topology |
PDB no./ref |
Names marked in italic are the nomenclature used in corresponding references. P and A in the topology column are abbreviations of parallel and antiparallel respectively. G4G3 will change conformation when incubated at different strand concentrations and ionic conditions. |
T30177a |
GTGGTGGGTGGGTGGGT |
Pb (stack) |
2M4P/ref. 25 |
NG16 |
GGGTGGGTTGGGTGGG |
P (stack) |
2LXV/ref. 26 |
c-kit87up |
AGGGAGGGCGCTGGGAGGAGGG |
P |
2O3M/ref. 27 |
c-myc |
TGAGGGTGGIGAGGGTGGGGAAGG |
P |
2A5P/ref. 27 |
93del |
GGGGTGGGAGGAGGG |
P (interlock) |
1Y8D/ref. 28 |
Ht-1 |
TTGGGTTAGGGTTAGGGTTAGGGA |
3 + 1 |
2GKU/ref. 28 |
Ht-2 |
TAGGGTTAGGGTTAGGGTTAGGG |
3 + 1 |
3CDM/ref. 28 |
Hb-G3 |
GGGTGGGTTTTGGGTTTGGG |
3 + 1 |
—/ref. 13 |
G3G3 |
GGGTTTTGGG |
Ab |
—/ref. 30 |
G4G4 |
GGGGTTTTGGGG |
A |
—/ref. 31 |
3T-(G3T)3G3 |
TTTGGGTGGGTGGGTGGG |
P |
— |
(G3T)3G3 |
GGGTGGGTGGGTGGG |
P (stack) |
— |
(G3T)3G3-3T |
GGGTGGGTGGGTGGGTTT |
P |
— |
N-myc |
TAGGGCGGGAGGGAGGGAA |
P |
2LED/ref. 37 |
G4G3c |
GGGGTTTTGGG |
A/P |
—/ref. 39 |
Experimental
Sample preparation
All DNA oligonucleotides listed in Table 1 (OPC grade) were purchased from TaKaRa Biotechnology Co., Ltd. (Dalian, China) and used without further purification. Synthesized DNA samples were dissolved in deionized water at a concentration of approximate 2 mM as a stock solution and stored under −20 °C. The exact strand concentration was obtained based on their UV absorbance measured at 260 nm at an elevated temperature of 90 °C and with the absorption coefficient acquired from the website http://www.scitools.idtdna.com/country.aspx.
For the oligonucleotides that were expected to form monomolecular or bimolecular G-quadruplexes when they were incubated, 100 μM DNA strand concentrations were used. For oligonucleotides that were supposed to form tetramolecular G-quadruplexes, such as TG4T and TG5T, the incubation strand concentrations were increased to 200 μM. To construct a higher-ordered G-quadruplex, higher strand and ion concentrations will be required and the detailed concentrations are described in the related section. DNA oligonucleotides were dissolved in either 60 mM ammonium acetate solution (pH = 6.8) or 60 mM KCl solution (pH = 7.0) and they were heated at 90 °C in a water bath for 10 min. The samples were then cooled to room temperature. After the cooling process, samples were kept below 4 °C for 48 hours (all sample concentrations mentioned in the article were designated as incubated concentrations). Before injecting into the mass spectrometer samples were mixed with the same volume of methanol for preferable ionization. The co-solvents (ethanol or methanol) increase the ESI-MS signals owing to better droplet desolvation.
Fluorescence spectroscopy
Fluorescence measurements were performed on a Hitachi F-2700 FL spectrophotometer (Tokyo, Japan). Fluorescence wavelength scanning mode was used at 260 nm excitation. The emission wavelength started from 300 nm and ended at 500 nm. Excitation and emission slits were set to 5.0 nm. The operating PMT voltage was 400 V. A 1 cm quartz cuvette was used. 200 μL samples prepared as above were diluted to 1 mL for fluorescence measurements. The same samples were also subjected to mass spectrometry analysis for comparison and confirmation. FL spectra of most sequences in either K+ or NH4+ solution were highly consistent except for slight changes in abundance. Hence, the FL spectra of sequences incubated in NH4+ solution were not displayed for simplification. All spectra obtained have the original fluorescence of K+ or NH4+ in a solution removed. Since entire fluorescence intensities of G-quadruplexes were relatively low, all FL spectra were normalized to the arrangement from 0 to 1 for qualitative analysis.
Mass spectrometry
ESI-MS experiments were performed in negative ion mode on a Bruker micro-Q-TOF mass spectrometer (Bremen, Germany). Optimized ESI-MS parameters were capillary voltage: 3300 kV; the source end plate offset: −500 V; the quadrupole ion energy: 5.0 eV; the funnel 1 and funnel 2 RF: 100 Vpp and 400 Vpp, respectively; collision energy: 5.0 eV; collision RF: 600 Vpp. Mass range was set from 50 to 3000 m/z. If necessary, ion energy or collision energy was tuned higher for better mass spectra.
Circular dichroism
CD spectra were obtained on a Jasco-810 spectropolarimeter (Tokyo, Japan) using a 200 μL micro quartz cuvette at room temperature. Scans from 220 to 320 nm were performed with 200 nm min−1, 1 nm pitch and 1 nm bandwidth. Each sample was recorded three times and the data were averaged. DNA strand concentration was 100 μM and ion concentration was 60 mM.
Results and discussion
Fluorescence spectra of stacked dimeric G-quadruplexes
Sequences in which guanine repeats were connected by single-residue bases were proven to favor stable propeller-type G-quadruplexes with all guanines in anti conformations. Such subunits can further stack on each other forming dimeric G-quadruplexes (Fig. 2a).32–34 Several sequences including T30695 and its derivatives have been studied by NMR, which are proven to stack on the 5′-5′ interface and to bring out the 385 nm emission band when excited at 260 nm.24 The formation of the particular 385 nm emission band is attributed to interfacial aromatic 5/6-ring overlaps. To further investigate and confirm the effect of base stacking, both at the interface and inside the G-quadruplex, on the production of the 385 nm emission band, several sequences were selected for further studies.
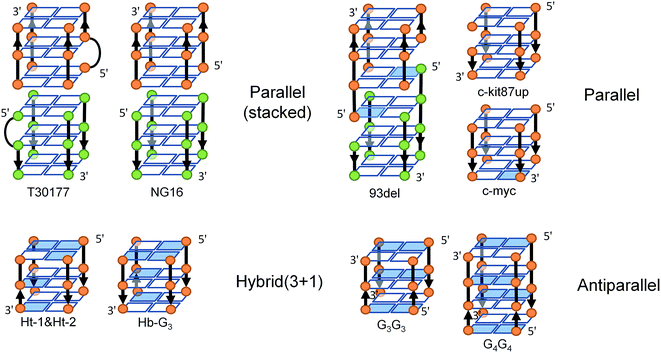 |
| Fig. 2 Schematic of well-defined G-quadruplexes formed by the sequences listed in Table 1. They are classified according to structural features in terms of parallel, antiparallel and 3 + 1 types of monomer as well as parallel stacked or parallel interlocked dimers. The nattier blue quadrangles stand for syn GBA guanines and white quadrangles stand for anti GBA guanines. For simplification, all loops and partial sugar-phosphate backbones are omitted. | |
Sequences of T30177 and NG16 (Table 1) have been demonstrated to form stacked dimeric G-quadruplexes with a structure similar to that of T30695.25,26 However, the exact base overlap of T30177 dimer in the interface is unclear. In contrast, the interfacial overlap of NG16 has been proven to be 6/6-ring stacking.33
Fluorescent spectra of these two sequences show characteristic emission bands at around 385 nm in line with prediction and previous results.24 However, it should be mentioned that the emission band of T30177 was not exactly at 385 nm. Instead, it shifted to 370 nm (Fig. S1†). This band shift might due to the bulges in the structure that affect the stacked overlaps. Mass spectrometry and CD were employed to confirm the formation of dimeric stacked G-quadruplexes generated by T30177 and NG16. Both the two sequences gave [2M + 5NH4+ − Hn+5]n− adduct peaks carrying different charges from 5 to 7 (Fig. S1†). Adduct peaks of [M + 2NH4+ − Hn+2]n− (n = 4, 5 or 6) correspond to monomeric G-quadruplexes with three G-quartets. Coexistence of monomers and dimers in the gas phase revealed equilibrium between monomolecular and stacked bimolecular G-quadruplexes in a solution. Featured CD spectra (around 240 nm negative band and 260 nm positive band) provided parallel G-quadruplex structure (Fig. S2†) consistent with the NMR structure: all guanines involved in G-quartets arrange in anti conformation.15
Thus, two additional sequences of T30177 and NG16 further confirmed that 5′-5′ stacked dimers of G-quadruplexes produced the characteristic fluorescence emission band at around 385 nm. In this case, it can be observed that all the guanines in G-quartets adopt anti conformations. However, partial 5/6-ring overlap at the stacking interface was not the only factor resulting in the formation of emissions at around 385 nm. Partial 6/6-ring overlap could also provide this featured band. To further investigate the key factors that induce the production of the characteristic emission band, a series of parallel, antiparallel and 3 + 1 types of G-quadruplexes with various GBA compositions and aromatic stacking modes were further studied.
Tracing fluorescence spectra of stacked guanines inside G-quadruplex cores
Parallel-stranded structures usually have stacked guanines with the same GBA (anti).15 However, introduction of guanine analogues to replace the original guanine bases can alter G-quartet polarity by changing the GBA of guanines. This particular base mutation will make all G-quartets in antiparallel structures to present in the same polarity and give “parallel” CD spectra.35 In contrast, antiparallel structures contain various stacking patterns, including syn–syn, syn–anti and anti–syn.
Several representative sequences (Fig. 2) that form a parallel interlocked dimer (93del), parallel monomers (c-kit87up and c-myc), 3 + 1 types of monomer (Ht-1 & Ht-2 and Hb-G3) and antiparallel dimeric G-quadruplexes (G3G3 and G4G4) were measured for fluorescence emissions. As shown in Fig. 3, all these sequences give an emission band at 330 nm. The syn–syn, syn–anti or anti–syn stacked guanines inside antiparallel G-quadruplexes show negatively characterized fluorescence signals. Nevertheless, the anti–anti stacking mode inside parallel G-quadruplexes, such as 93del, c-kit87up and c-myc, displayed a subtle red shift tendency to give inconspicuous bulges at wavelengths from 385 nm to 425 nm in the fluorescence spectra (Fig. 3). Note that according to structural information obtained from the Protein Data Bank (PDB), the aromatic stacking of these parallel structures was by partial 5/6-ring overlaps in the G-quadruplex cores. This indicated that anti base stacking in the shape of 5/6-ring overlaps packaged inside the G-quadruplex had an influence on entire FL signals though it was a slight change to some extent. The reason that the bulge in FL for 93del was not as intense as that for the two parallel monomers might due to the different base stacking at the interface, which impairs the anti base stacking. Therefore, we could utilize this particular fluorescence feature to distinguish whether a preconceived sequence forms into dimeric stacked G-quadruplexes, parallel G-quadruplex monomers or other types. However, the CD spectra were needed for further confirmation (see the ESI†).
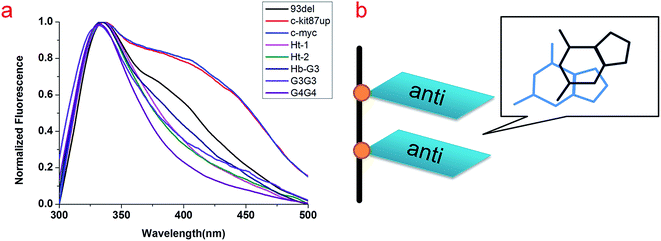 |
| Fig. 3 (a) Fluorescence spectra of sequences introduced to measure the FL effect of stacked guanines. All spectra obtained have the original fluorescence of K+ in a solution. (b) Schematic for anti–anti base stacking containing partial 5/6-ring overlap in a parallel G-quadruplex. | |
Fluorescence properties of higher-ordered stacked structures
To date, parallel 5′-5′ stacked structures have been demonstrated to show peculiar fluorescence spectra excited at 260 nm. In order to examine the effect of 5′-5′ and 3′-3′ stacks on fluorescence spectra, the sequence of T30695 was modified by removing thymine residues at both ends and adding 3T at either terminus of the sequence. To regulate stacking probability, the 3′-end thymine of T30695 was removed to obtain a new sequence herein named (G3T)3G3. 3T-(G3T)3G3 with flanking residues at the 5′-end could provide steric hindrance wherein the 5′-5′ stacking occurred. Similarly, (G3T)3G3-3T was designed to impede the probable 3′-3′ stacking. Interestingly, GGGT motifs having 5′-blocking bases indeed affect the stacking equilibrium between monomers and dimers by NMR;32 however, the mass spectrum of 3T-(G3T)3G3 presented bimolecular adduct peaks involving 5 NH4+ referring to dimers formed in a solution (Fig. 4a). Subsequently, its fluorescence spectrum gave 330 nm emission, which was not the featured fluorescence band of 5′-5′ stacking dimers (Fig. 4a′). The stacking patterns at the interface of the T30695 sequence and its analogues have been provided by NMR structures or MD simulations, in which it is primarily the 5′-5′ way. However, results from MS and fluorescence indicated that the 5′-end blocking sequence 3T-(G3T)3G3 might form into a 3′-3′ stacking dimeric G-quadruplex. For the sequence (G3T)3G3, the strand concentration as well as the ionic concentration when it was incubated was twice of what we normally use. In the MS spectrum, dimers including 5 NH4+ and trimers with 8 NH4+ adduct peaks were observed, revealing that higher-ordered stacked structures might be involved. Moreover, it was worth noting that its FL spectrum showed 385 nm emission with a bulge peak at around 335 nm. We assumed that the reason causing this particular FL spectrum was the cooperated FL effect by both monomers and 5′-5′ stacking. To confirm our hypothesis, the mixture containing incubated T30695 and 93del at the same concentration was subjected to fluorescence measurement (Fig. S3†). As we expected, the mixed solution displayed a similar 385 nm emission peak containing a bulge. The last oligonucleotide in this part was (G3T)3G3-3T. Its mass spectrum showed monomeric and dimeric G-quadruplexes. FL presented a 385 nm emission band referring to 5′-5′ stacking.
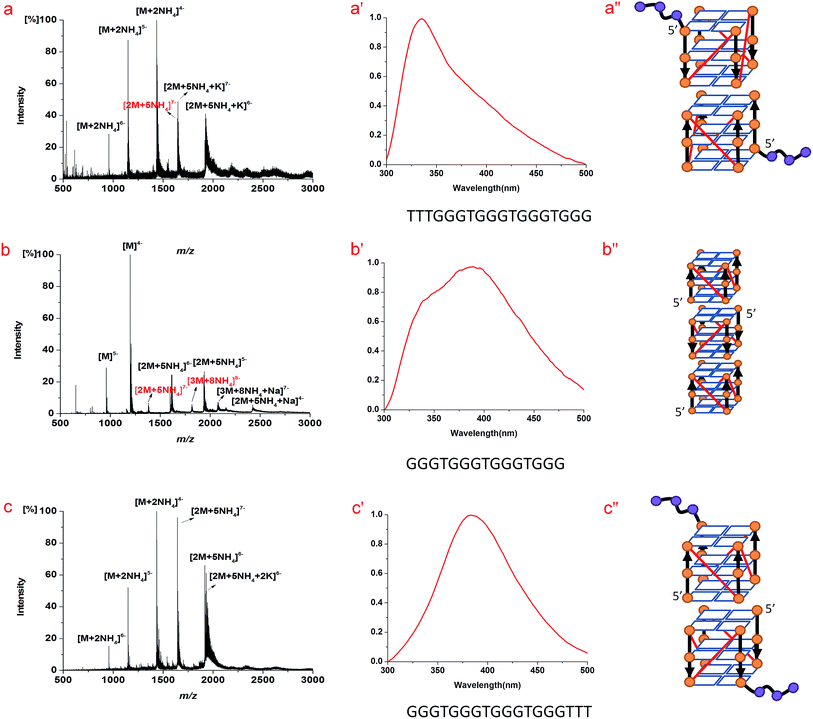 |
| Fig. 4 (a) Mass spectrum of 3T-(G3T)3G3: 100 μM strand concentration and 60 mM ammonium acetate. (a′) Fluorescence spectrum of 3T-(G3T)3G3: 100 μM strand concentration and 60 mM KCl. (a′′) Schematic structure of 3T-(G3T)3G3. (b) Mass spectrum of (G3T)3G3: 200 μM strand concentration and 150 mM ammonium acetate. (b′) Fluorescence spectrum of (G3T)3G3: 200 μM strand concentration and 150 mM KCl. (b′′) Schematic structure of (G3T)3G3. (c) Mass spectrum of (G3T)3G3-3T: 100 μM strand concentration and 60 mM ammonium acetate. (c′) Fluorescence spectrum of (G3T)3G3-3T: 100 μM strand concentration and 60 mM KCl. (c′′) Schematic structure of (G3T)3G3-3T. All stacked guanines are anti GBA configuration. Red straight lines present propeller loops. | |
To summarize this section, we have synthesized three oligonucleotides by modifying the sequence of T30695 to examine FL features of higher-ordered stacking. The most significant findings were that (i) flanking residues congested the 5′-end of GGGT motifs, which still had the stacking ability. This might be the rarely formed 3′-3′ stacking; (ii) (G3T)3G3 sequence at increased incubation concentration would adopt a higher-ordered stacking mode, which is supposed to be a combination of 5′-5′ and 3′-3′ stacking.
Describing other fluorescence specificity corresponding to G-quadruplex formations
The oligonucleotide N-myc was acquired from intron of N-myc gene.36 Confirmed by NMR, an equilibrium of monomers and dimers in K+ solution was observed.37 The unique feature of the dimers was that all six G-quartets were stacked within an entire G-quadruplex, which comprised two oligonucleotide subunits (Fig. S4†). All the stacked guanines presented anti GBA and the stacking mode was 5/6-ring overlap. Structure information from MS and CD was consistent with the NMR parallel structure (Fig. S4†). The fluorescence of N-myc provided both 330 nm and 395 nm emission bands (Fig. 5a). However, the more remarkable 395 nm emission band might due to more stacking anti guanines in G-quartets and more 5/6-ring overlaps. In order to confirm our assumption, oligonucleotides TG4T (5′-TGGGGT-3′) and TG5T (5′-TGGGGGT-3′) were investigated under the same conditions, which tend to form tetramolecular G-quadruplexes.38 For sequence TG4T and TG5T, the most abundant component in a solution should be the G-quadruplexes with four G-quartets and five G-quartets, respectively. More stacked anti guanines were involved in these sequences. However, their fluorescence spectra showed 330 nm emissions (Fig. S5†) and their CD spectra showed unique characterized peaks of parallel G-quadruplexes. This result indicated that the 395 nm emission was not supposed to be generated by more stack anti guanines. Nevertheless, there were interfaces between different G-quadruplex subunits in N-myc. According to our experimental results mentioned above, the 385 nm emissions relate to the interface base stacking. Hence, 395 nm emission should be caused by interface stacking.
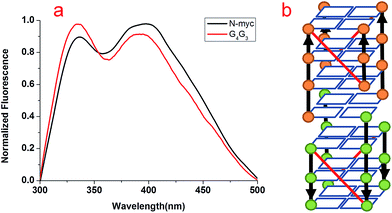 |
| Fig. 5 (a) Fluorescence spectra of N-myc (black) and G4G3 (red). (b) Schematic structure of G4G3. Spheres with different colors are only a matter of distinguishing the guanine donors. All stacked guanines are anti GBA configuration. Red straight lines represent propeller loops. | |
G4G3 was initially determined by crystal diffraction to form dimeric antiparallel G-quadruplex.39 However, our previous study revealed that it tended to transform into a polymolecular parallel structure under higher concentration.40 Therefore, we increased the strand concentration to 200 μM and enhanced the ionic condition to 150 mM. From the FL spectrum, we could observe the 330 nm and 395 nm emission, indicating the same types of guanines stacking. Due to the unequal size of G-rich motifs in the sequence, it was supposed that G1 at the 5′-end of each oligonucleotide would slip out and form a nonaligned G-quartet between two subunits (Fig. 5b). Considering the relative intensity of the 395 nm emission peak, all guanines should be arranged and stacked in anti conformation, whereas the detailed base aromatic overlaps could not be identified.
Conclusion
We built correlations between G-quadruplex structures and featured fluorescence emission bands by studying several well-defined sequences and synthesized oligonucleotides. For antiparallel structures, no obvious clues emerged based on fluorescence measurements to clarify the topology diversity. However, parallel G-quadruplexes with anti base stacks either inside or on the interface do show tendency of an emission band at around 385 nm. It follows in the order: 5′-5′ interface stacking > 5′-5′ interface pairing > inside stacking. The 5/6 ring overlap on the interface is not necessary to give the featured emission. However, the flexibility of a stacked interface may have an important effect on the generation and intensity of the featured band. More structure information can be obtained from the FL spectra. The limitation is that we can only perform qualitative analysis to some extent, owing to the low fluorescence quantum yield of G-quadruplexes. In our prospective study, increasing the G-quadruplex to an adequate extent might contribute more FL intensity in order to refine different patterns of base stacking.
Acknowledgements
This study was financially supported by the National Natural Science Foundation of China (no. 20975044, 21675060 and 51273080), the International Collaboration Project of Jilin Province (20160414010GH) and the Open Project of State Key Laboratory for Supramolecular Structure and Materials (SKLSSM 201610). We thank associate Professor Xinghua Wang at the College of Chemistry, Jilin University for his kind support in the use of the fluorescence spectrophotometer.
References
- J. T. Davis, Angew. Chem., Int. Ed., 2004, 43, 668–698 CrossRef CAS PubMed.
- J. L. Huppert, Chem. Soc. Rev., 2008, 37, 1375–1384 RSC.
- A. T. Phan, V. Kuryavyi and D. J. Patel, Curr. Opin. Struct. Biol., 2006, 16, 288–298 CrossRef CAS PubMed.
- S. Burge, G. N. Parkinson, P. Hazel, A. K. Todd and S. Neidle, Nucleic Acids Res., 2006, 34, 5402–5415 CrossRef CAS PubMed.
- H. J. Lipps and D. Rhodes, Trends Cell Biol., 2009, 19, 414–422 CrossRef CAS PubMed.
- G. W. Collie and G. N. Parkinson, Chem. Soc. Rev., 2011, 40, 5867–5892 RSC.
- J. L. Huppert, Biochimie, 2008, 90, 1140–1148 CrossRef CAS PubMed.
- A. K. Todd, M. Johnston and S. Neidle, Nucleic Acids Res., 2005, 33, 2901–2907 CrossRef CAS PubMed.
- S. Neidle, Curr. Opin. Struct. Biol., 2009, 19, 239–250 CrossRef CAS PubMed.
- C. Cao, J. Zhang, X. Wen, S. L. Dodson, D. Nguyen Thuan, L. M. Wong, S. Wang, S. Li, P. Anh Tuan and Q. Xiong, ACS Nano, 2013, 7, 7583–7591 CrossRef CAS PubMed.
- G. I. Livshits, A. Stern, D. Rotem, N. Borovok, G. Eidelshtein, A. Migliore, E. Penzo, S. J. Wind, R. Di Felice, S. S. Skourtis, J. Carlos Cuevas, L. Gurevich, A. B. Kotlyar and D. Porath, Nat. Nanotechnol., 2014, 9, 1040–1046 CrossRef CAS PubMed.
- M. Webba da Silva, Chem.–Eur. J., 2007, 13, 9738–9745 CrossRef CAS PubMed.
- M. Webba da Silva, M. Trajkovski, Y. Sannohe, N. Ma'ani Hessari, H. Sugiyama and J. Plavec, Angew. Chem., Int. Ed., 2009, 48, 9167–9170 CrossRef CAS PubMed.
- A. I. Karsisiotis, N. M. Hessari, E. Novellino, G. P. Spada, A. Randazzo and M. Webba da Silva, Angew. Chem., Int. Ed., 2011, 50, 10645–10648 CrossRef CAS PubMed.
- C. J. Lech, B. Heddi and A. T. Phan, Nucleic Acids Res., 2013, 41, 2034–2046 CrossRef CAS PubMed.
- P. Hobza, Phys. Chem. Chem. Phys., 2008, 10, 2581–2583 RSC.
- P. Changenet-Barret, Y. Hua, T. Gustavsson and D. Markovitsi, Photochem. Photobiol., 2015, 91, 759–765 CrossRef CAS PubMed.
- M. A. Mendez and V. A. Szalai, Biopolymers, 2009, 91, 841–850 CrossRef CAS PubMed.
- D. J. M. Blanchard, T. Z. Cservenyi and R. A. Manderville, Chem. Commun., 2015, 51, 16829–16831 RSC.
- L. Olejko, P. J. Cywinski and I. Bald, Angew. Chem., Int. Ed., 2015, 54, 673–677 CAS.
- A. Tanaka, J. Choi and T. Majima, RSC Adv., 2014, 4, 59071–59077 RSC.
- R. Kundu, Chem.–Asian J., 2016, 11, 198–201 CrossRef CAS PubMed.
- N. T. Dao, R. Haselsberger, M. E. Michel-Beyerle and A. T. Phan, FEBS Lett., 2011, 585, 3969–3977 CrossRef PubMed.
- N. T. Dao, R. Haselsberger, M. E. Michel-Beyerle and A. T. Phan, ChemPhysChem, 2013, 14, 2667–2671 CrossRef CAS PubMed.
- V. T. Mukundan, N. Q. Do and A. T. Phan, Nucleic Acids Res., 2011, 39, 8984–8991 CrossRef CAS PubMed.
- V. Kuryavyi, L. A. Cahoon, H. S. Seifert and D. J. Patel, Structure, 2012, 20, 2090–2102 CrossRef CAS PubMed.
- A. T. Phan, V. Kuryavyi, S. Burge, S. Neidle and D. J. Patel, J. Am. Chem. Soc., 2007, 129, 4386–4392 CrossRef CAS PubMed.
- A. T. Phan, V. Kuryavyi, J. B. Ma, A. Faure, M. L. Andreola and D. J. Patel, Proc. Natl. Acad. Sci. U. S. A., 2005, 102, 634–639 CrossRef CAS PubMed.
- K. N. Luu, A. T. Phan, V. Kuryavyi, L. Lacroix and D. J. Patel, J. Am. Chem. Soc., 2006, 128, 9963–9970 CrossRef CAS PubMed.
- F. W. Smith, F. W. Lau and J. Feigon, Proc. Natl. Acad. Sci. U. S. A., 1994, 91, 10546–10550 CrossRef CAS.
- P. Schultze, N. V. Hud, F. W. Smith and J. Feigon, Nucleic Acids Res., 1999, 27, 3018–3028 CrossRef CAS PubMed.
- N. Q. Do and A. T. Phan, Chem.–Eur. J., 2012, 18, 14752–14759 CrossRef CAS PubMed.
- N. Q. Do, K. W. Lim, M. H. Teo, B. Heddi and A. T. Phan, Nucleic Acids Res., 2011, 39, 9448–9457 CrossRef CAS PubMed.
- M. Adrian, D. J. Ang, C. J. Lech, B. Heddi, A. Nicolas and A. T. Phan, J. Am. Chem. Soc., 2014, 136, 6297–6305 CrossRef CAS PubMed.
- V. V. Cheong, C. J. Lech, B. Heddi and A. T. Phan, Angew. Chem., Int. Ed., 2016, 55, 160–163 CrossRef CAS PubMed.
- X. X. Tang, H. Zhao, B. Kung, D. Y. Kim, S. L. Hicks, S. L. Cohn, N. K. Cheung, R. C. Seeger, A. E. Evans and N. Ikegaki, Cancer Res., 2006, 66, 2826–2833 CrossRef CAS PubMed.
- M. Trajkovski, M. W. da Silva and J. Plavec, J. Am. Chem. Soc., 2012, 134, 4132–4141 CrossRef CAS PubMed.
- L. Joly, F. Rosu and V. Gabelica, Chem. Commun., 2012, 48, 8386–8388 RSC.
- M. Črnugelj, N. V. Hud and J. Plavec, J. Mol. Biol., 2002, 320, 911–924 CrossRef.
- X. Guo, S. Liu and Z. Yu, J. Am. Soc. Mass Spectrom., 2007, 18, 1467–1476 CrossRef CAS PubMed.
Footnote |
† Electronic supplementary information (ESI) available. See DOI: 10.1039/c6ra21347b |
|
This journal is © The Royal Society of Chemistry 2016 |
Click here to see how this site uses Cookies. View our privacy policy here.