DOI:
10.1039/C6RA20903C
(Paper)
RSC Adv., 2016,
6, 105661-105675
Transferrin-conjugated drug/dye-co-encapsulated magnetic nanocarriers for active-targeting fluorescent/magnetic resonance imaging and anti-tumor effects in human brain tumor cells†
Received
19th August 2016
, Accepted 27th October 2016
First published on 27th October 2016
Abstract
A combinatorial nanosystem with the advantages of superparamagnetic iron oxide nanoparticles (SPIO NPs) and targeting polymer carriers is expected to improve the therapeutic effects in developing multifunctional delivery systems. Here we developed an innovative tumor-specific multi-functional SPIO NPs nanoplatform containing the antitumor drug doxorubicin (DOX) and fluorescent dye rhodamine B isothiocyanate (RBITC) for theranostic analysis and anti-tumor therapy in human brain tumor U251 MG cells. The core nanocarrier SPIO NPs (γ-Fe2O3) were synthesized and decorated with chitosan (CS), subsequently followed with conjugation with tumor-specific ligand transferrin (Tf) to fabricate tumor-targeted Tf–CS/SPIO NPs. The anti-tumor drug DOX was then loaded onto Tf–CS/SPIO NPs, and transferred into U251 MG cells for assaying their biological effects. Besides, the produced Tf–CS/SPIO NPs were fluorescently labeled with RBITC for simultaneously intracellular fluorescent/magnetic resonance imaging in targeted U251 MG cells. The results showed that the fabricated Tf–CS/SPIO NPs nanocarriers demonstrated some favorable properties, including immediate responses under magnetic fields, stable behavior in different media, efficient encapsulation for drug loading, undetectable cytotoxicity, and effective intracellular visualization. Moreover, the fluorescent Tf–CS/SPIO NPs could be successfully applied for concurrent fluorescent/magnetic resonance imaging, and the finalized drug-loading Tf–CS/SPIO NPs displayed an improved cellular uptake, and thus effectively killed tumor cells through inducing a concurrence of cell apoptosis and autophagy in the treated tumor U251 cells. Therefore, the fabricated Tf–CS/SPIO NPs should be of great significance in developing multi-purpose nanocarriers for anti-tumor drug delivery and fluorescent/magnetic resonance imaging in human brain tumor treatments.
1 Introduction
Glioblastoma multiforme (GBM, grade IV), also known as glioblastoma, is the most common and aggressive malignant primary brain tumor among adults, accounting for approximately half of all brain tissue tumors.1 GBM patients show a poor prognosis and usually survive for less than 15 months.2 The 2 year survival rate is less than 3%.3 Therefore, tremendous efforts have been made to develop effective therapeutic strategies for anti-tumor therapy in glioma treatments. Chemotherapy is one of commonly applied treatment strategy for malignant glioma.4,5 However, traditional chemotherapy is usually limited mainly by the low selectivity of anticancer drugs toward tumor cells (they kill tumor cells as well as affect the growth of normal tissue cells), causing severe side effects.6,7 Improvement of drug delivery strategies has become an important consideration in the effort to increase local effective therapeutic concentration of drugs, to further facilitate the efficiency of drug delivery to specific tissues and improve therapeutic efficacy, and to minimize toxicity and side effects in patients.8,9
Recent advances in tumor drug delivery strategies have suggested that nanocarriers can improve the prolonged circulation time and can significantly reduce toxicity compared with carrier-free drug delivery systems to tumors.10–12 Among these nanocarriers, the suitably modified superparamagnetic iron oxide nanoparticles (SPIO NPs, especially Fe3O4 and γ-Fe2O3), which have been extensively employed for biomedical applications, have drawn interest as drug delivery vehicles to improve therapeutic efficacy owing to their non-toxicity, attractive physicochemical characteristics, flexible design and surface functional modification, and outstanding superparamagnetic properties under an external magnetic field.13–15 Despite the improvements in pharmacokinetics, one great challenge that currently confronts SPIO NPs is specific targeting and enhancement of the therapeutic efficacy of formulation.16,17 Recent studies have proposed that the clinical efficiency of SPIO NPs in anti-tumor agent delivery can be further improved by employing the active targeting strategy, which involves increased specific targeting of drug delivery to tumor cells.18,19 Active targeting, i.e., nanoparticles (NPs), can specifically deliver anti-tumor drugs into target cells via receptor-mediated endocytosis and can efficiently deliver drug into malignant cells, resulting in high local concentration of chemotherapeutics and reduction of severe side effects.20–22 One of the most widely used tumor targeting ligand is transferrin (Tf), a human serum glycoprotein involved in transport of ferric ions in vivo with low immunogenicity; Tf can specifically bind to the carrier protein transferrin receptor (TfR),23 which is a dimeric transmembrane glycoprotein that is overexpressed in many types of tumor tissues.24–26 Thus, Tf-conjugated NPs could selectively deliver therapeutic agents into TfR-overexpressing tumor cells through TfR-mediated endocytosis.27
To meet the demand for potential clinical application of targeted anti-tumor therapies, the use of multifunctional nanocarriers for simultaneous targeted imaging, drug delivery, and noninvasive detection and therapeutic capabilities is desirable.28–30 Therefore, we proposed a novel nanoplatform consisting of four key components: (i) the carrier core SPIO NPs for MRI; (ii) Tf ligand target modification for tumor cell targeting; (iii) fluorescent dye (RBITC) for imaging; and (iv) anti-tumor drug doxorubicin (DOX) encapsulated to induce tumor cell apoptosis.31,32 Keeping these in minds, we thus constructed a multi-functional SPIO NPs nanoplatform for combined fluorescent imaging, magnetic resonance imaging (MRI), and targeted tumor therapy, and their biological effects were evaluated in human brain tumor U251 MG cells.
2 Experimental section
2.1 Materials
The SPIO NPs (i.e., γ-Fe2O3) used in this study were synthesized from magnetite (Fe3O4) according to previously proposed methods.33,34 The human brain tumor U251 MG cell line was obtained from Shanghai Cell Bank of the Chinese Academy of Sciences (Shanghai, China). Cell culture medium and fetal bovine serum (FBS) were purchased from Gibco Invitrogen Corporation (CA, USA). Doxorubicin (DOX) was purchased from Aladdin Industrial Co., Ltd. (Shanghai, China). CS, Tf, sodium tripolyphosphate (TPP), dimethyl sulfoxide (DMSO), RBITC, fluorescein diacetate (FDA), propidium iodide (PI), acridine orange (AO), 3-(4,5-dimethylthiazlo-2-diphenyl-tetrazolium)bromide (MTT), Hoechst 33258, sulfo-SMCC, and Traut's reagent were purchased from Sigma-Aldrich (St. Louis, MO, USA). Rhodamine phalloidin was obtained from Cytoskeleton Inc. (Denver, CO, USA). Reactive oxygen species (ROS) assay and terminal deoxynucleotidyl transferase-mediated dUTP nick end labeling (TUNEL) cell apoptosis detection kits were obtained from Keygen Biotech Co., Ltd. (Nanjing, China). Monoclonal mouse anti-LC3A/B antibody and Alexa Fluor 488 goat anti-mouse IgG were obtained from Proteintech Group, Inc. (Chicago, IL, USA). The fluorescent dye 4,6-diamidino-2-phenylindole (DAPI) was purchased from Molecular Probes Inc. (Eugene, OR, USA). Other reagents and chemicals with analytical grade were purchased from local commercial suppliers. Deionized (DI) water (Milli-Q, Millipore, Bedford, MA) was used to prepare aqueous solutions.
2.2 Assay principle of Tf–CS/SPIO NP preparation and DOX loading
In principle, the fabrication of DOX loading magnetic Tf–CS/SPIO NPs (Scheme 1) starts with synthesis of the magnetic core SPIO NPs, i.e., γ-Fe2O3, then followed with CS modification, Tf conjugation, RBITC labeling and DOX loading, and the constructed multi-purpose NPs were subsequently delivered into U251 MG cells for assaying of their biological effects.
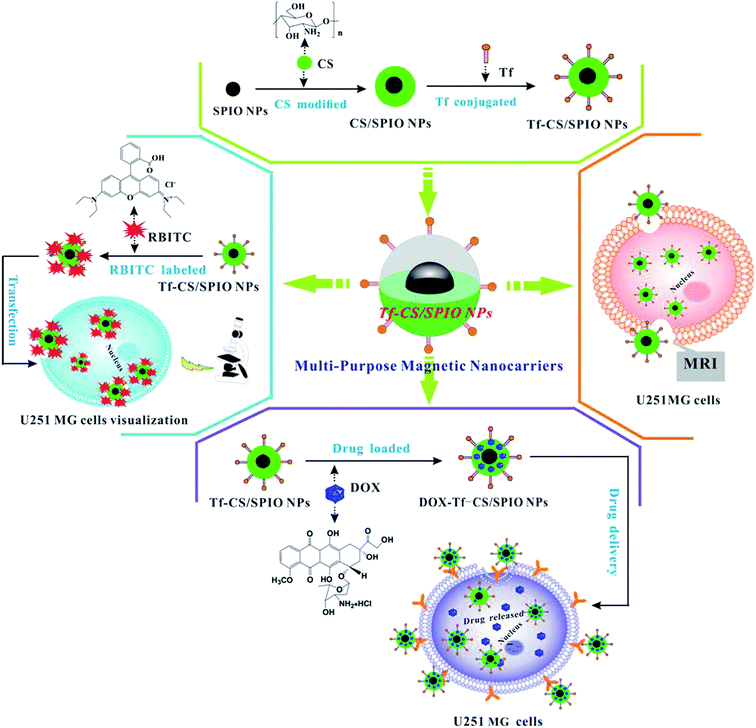 |
| Scheme 1 Schematic representation of fabrication of magnetic nanocarriers (Tf–CS/SPIO NPs) for targeted intracellular visualization, drug delivery and fluorescent/MRI analysis in U251 MG cells. | |
2.3 Synthesis of Tf–CS/SPIO NPs
In this study, the magnetic SPIO NPs (γ-Fe2O3) were firstly synthesized as superparamagnetic core nanocarriers through chemical co-precipitation method as previously described.33,34 To surface modify SPIO NPs with CS, 25 mL of TPP (1 mg mL−1) was mixed with SPIO NPs (10 mg mL−1) and then vigorously stirred for 30 min at 60 °C. The mixture was incubated for 12 h at room temperature (RT), and then washed thrice with DI water to obtain TPP–SPIO NPs. 5.5 mL of CS solution (1% w/v, dissolved in acetic acid) was mixed with 30 mL of TPP–SPIO NPs (2 mg mL−1) and allowed to react for 30 min in ultrasonic emulsifier. The formed CS/SPIO NPs were washed thrice with DI water.
The CS/SPIO NPs were modified with Tf by using the heterobifunctional crosslinker sulfo-SMCC and the Traut's reagent method as outlined in Scheme 2. The Tf was first thiolated by mixing 1 mL of Tf (5 mg mL−1) in borate buffer (pH 8, containing 2 mM EDTA) followed by addition of 86 μL of Traut's reagent (2 mg mL−1), and then the mixture was reacted under stirring for 60 min at RT to obtain Tf–SH. The CS/SPIO NPs were crosslinked with sulfo-SMCC by adding sulfo-SMCC solution (545 μL, 2 mg mL−1) into 5 mL CS/SPIO NPs suspension (10 mg mL−1), to produce sulfo-SMCC–CS/SPIO NPs. Finally, the prepared Tf–SH was added into the sulfo-SMCC–CS/SPIO NP solution and kept for 30 min to obtain Tf–CS/SPIO NPs. The obtained Tf–CS/SPIO NPs were washed thrice with PBS (0.1 M, pH 7.4), re-dispersed in PBS (pH 7.4), and stored at 4 °C.
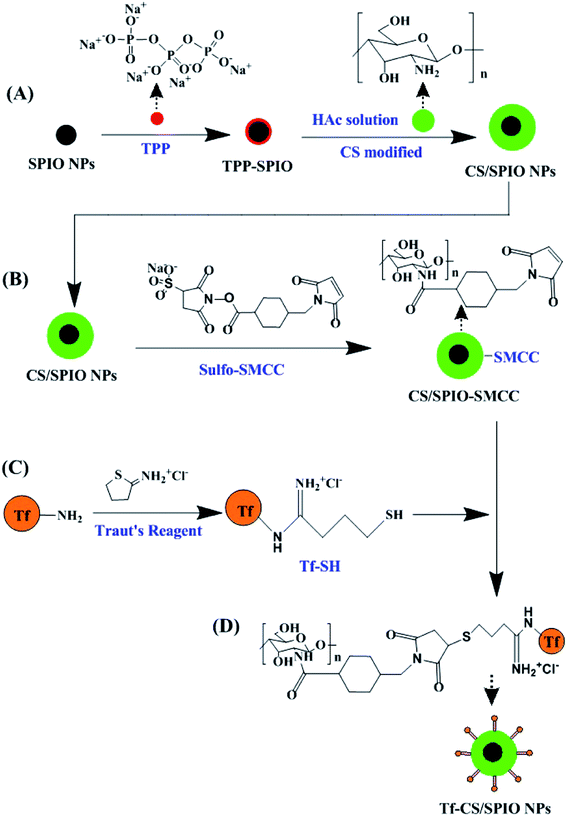 |
| Scheme 2 Schematic illustration for chemical formation of Tf–CS/SPIO NPs. (A) SPIO NPs were synthesized and modified with CS through TPP to prepare CS/SPIO NPs, (B) CS/SPIO NPs was crosslinked with sulfo-SMCC, (C) Tf was thiolated through Traut's reagent in borate buffer to form Tf–SH, and Tf–CS/SPIO NPs were synthesized (D). | |
2.4 Magnetic responsiveness and stability of Tf–CS/SPIO NPs
The magnetic response and stability of the prepared Tf–CS/SPIO NPs in different media were analyzed according to a previously described protocol.35 The Tf–CS/SPIO NPs (100 μg mL−1) were ultrasonically dispersed in DI water, RPMI-1640 culture medium, and PBS (pH 7.4) at RT under magnetic field. The magnetic responsiveness and stability of Tf–CS/SPIO NPs were then examined by measuring the optical absorbance of the dispersions at predetermined time points by using a UV-Vis spectrophotometer at 480 nm.
2.5 DOX loading and encapsulation efficiency
DOX solution (500 μL, 2 mg mL−1) was mixed with 1 mL of CS/SPIO NPs or Tf–CS/SPIO NP solution (10 mg mL−1), and stirred for 1 h, and then washed thrice with DI water. The DOX loading was assessed by determining the absorbance at 480 nm by using a UV-visible spectrophotometer. Drug loading was defined as the amount of drug (mg) loaded for every 100 mg of polymer, whereas encapsulation efficiency was defined by the ratio of encapsulated drug to the initial amount of drug.
Wt is the weight of DOX in NPs, Ws is the weight of NPs, and W0 is the initial weight of DOX in the system.
2.6 Fluorescent RBITC-labeled Tf–CS/SPIO NPs
Tf–CS/SPIO NP solution (5 mL, 2 mg mL−1, dissolved in borate buffer) was added into 50 μL of RBITC solution (2 mg mL−1, dissolved in borate buffer), and the mixture was slowly stirred for 2 h at RT in the dark. The mixture was transferred into an ultrafiltration tube, centrifuged at 2000 rpm for 15 min to remove residual RBITC. The obtained RBITC–Tf–CS/SPIO NPs were stored at 4 °C until use.
2.7 Cell culture, cytotoxicity, and cell viability assays
The U251 MG cells were grown in RPMI 1640 medium supplemented with 8% (v/v) inactivated FBS (Gibco), 1% L-glutamine, 1% penicillin (100 U mL−1), and 1% streptomycin (100 μg mL−1) in a humidified incubator at 37 °C under 5% CO2. The cultured U251 MG cells were regularly monitored under an inverted light microscope, and then subcultured at 1
:
3 ratio every 72 h to maintain the exponential growth phase.
The cytotoxicity of the prepared NPs was evaluated. MTT assay was performed to evaluate cell proliferation of the treated U251 MG cells. Briefly, the U251 MG cells were seeded in 96-microwell plates at a density of 1 × 104 cells per well and cultured for 24 h. Various concentrations of CS/SPIO NPs, Tf–CS/SPIO NPs, DOX–CS/SPIO NPs, and Tf–CS/SPIO NPs (0, 25, 50, 100, 200, and 400 μg mL−1) were separately added into the culture wells and then further incubated for 48 h. The culture medium was removed and washed thrice with PBS (pH 7.4). MTT solution (200 μL, 0.5 mg mL−1) was then added into each well and incubated for another 4 h. Finally, 150 μL of DMSO was added followed by incubation for another for 15 min in a shaking incubator to thoroughly dissolve the formazan into the solvent. The absorbance of the sample was measured at 570 nm by using a microplate spectrophotometer (Bio Tek Instrument Inc., USA).
The cellular viability of the treated U251 MG cells was assessed using the FDA and PI double staining method.36,37 The U251 MG cells were seeded at a density of 2 × 104 cells per well and then cultured for 24 h. Various concentrations of Tf–CS/SPIO NPs, DOX–CS/SPIO NPs, and Tf–CS/SPIO NPs (0, 25, 50, 100, 200, and 400 μg mL−1) were subsequently added into the culture wells and incubated for another 48 h. FDA solution (1 μg mL−1) and PI solution (20 μg mL−1) were successively introduced into the culture plates, and then incubated for 10 min at RT. The living cells were stained green by FDA, whereas the dead cells were stained red by the fluorescent dye PI. Cell viability was then estimated by counting the live and the dead cells under an inverted fluorescence microscope (Eclipse TE 2000-U, Nikon, Kyoto, Japan) equipped with a high-resolution CCD camera (CV-S3200, JAI Co., Japan).
2.8 Evaluation of intracellular uptake
To examine the intracellular uptake of CS/SPIO NPs and Tf–CS/SPIO NPs by U251 MG cells, the CS/SPIO NPs and Tf–CS/SPIO NPs were fluorescently labeled with RBITC. Cellular uptake was subsequently analyzed by co-culturing U251 MG cells with RBITC-labeled CS/SPIO NPs and Tf–CS/SPIO NPs (RBITC–CS/SPIO NPs and RBITC–Tf–CS/SPIO NPs). The culture medium and the residual Tf–CS/SPIO NPs were then replaced and washed thrice with PBS after incubation for 4 h. The cells were fixed for 15 min with 4% paraformaldehyde (Sigma-Aldrich), washed thrice with PBS, and then counterstained with DAPI (100 nM, Sigma-Aldrich) for 10 min at RT to reveal the nuclei. The treated cells were rinsed thrice with PBS. The prepared samples were subsequently observed under an inverted fluorescence microscope equipped with a high-resolution CCD camera.
The cellular uptake of CS/SPIO NPs and Tf–CS/SPIO NPs was quantified by an atomic absorption spectrophotometer (AAS) (Hitachi Z-2000, Japan). The treated U251 MG cells were mineralized in a mixture of perchloric and nitric acids (4
:
1 ratio, v/v) by using a microwave mineralizator (Millestone ETHOS 900, Sydney, Australia) after removing the unincorporated iron particles. The samples were properly diluted with PBS (pH 7.4) solution for AAS analysis, and the iron contents were calculated based on a calibration curve of FeCl3.
2.9 Apoptotic assay
To visualize the apoptotic cells, the treated U251 MG cells were fixed with 4% paraformaldehyde for 15 min, washed thrice with PBS, and then stained with the bisbenzimide dye Hoechst H33258 solution (2 μg mL−1) for 10 min at RT. The number of apoptotic cells was measured using the TUNEL cell apoptosis detection kit (Keygen Biotech. Co., Ltd., Nanjing China) according to the manufacturer's protocol. The TRITC-labeled TUNEL-positive cells were visualized using a fluorescence microscope (Leica, Wetzlar, Hessen, Germany).
2.10 Autophagy assay
The volume of acidic vesicular organelles (AVOs), as a marker of autophagy was detected by staining with the lysosomotropic agent AO. And another autophagosome marker LC3 was also analyzed with immunocytochemistry staining. In brief, the treated U251 MG cells were fixed with 4% paraformaldehyde (w/v), and permeabilized with Triton X-100 solution (0.1%, v/v), and then incubated with 5% calf serum (v/v) for 30 min at 37 °C. Subsequently the pretreated cells were incubated with anti-LC3A/B antibody (mouse monoclonal IgG1, 1
:
100) at 4 °C overnight, and with Alexa Fluor 488 goat anti-mouse IgG (1
:
20) at 37 °C for 1 h. The cell nuclei were counterstained with DAPI (100 nM; Sigma-Aldrich) to reveal the nuclei.
2.11 Western blot analysis
The cells were lysed in a buffer containing 50 mM Tris–HCl (pH 7.4), 150 mM NaCl, 1% NP-40, 0.5% sodium deoxycholate, 0.1% SDS, 2 mM PMSF, and a protease inhibitor cocktail for 15 min on ice, and then heated at 100 °C in metal bath for 5 min. The total protein extracts (10 μg) were separated by 12% SDS-PAGE and transferred onto a PVDF membrane. The membrane was blocked with 8% (w/v) nonfat dry milk in PBS–Tween 20 (PBST; 0.05%) for 1 h and then incubated with primary antibodies (1
:
5000 in PBST) at 4 °C overnight, an then incubated with the appropriate HRP-conjugated secondary antibodies (1
:
10
000) for 1 h at RT. The immunoreactive bands were developed with the Pierce ECL (Thermo Fisher Scientific, Waltham, MA, USA) western blotting system. The relative quantity of the proteins was analyzed using the Quantity one software.
2.12 Immunofluorescent staining of actin cytoskeleton
The morphology and F-actin cytoskeleton of the U251 MG cells were investigated after treatment. Actin cytoskeleton was stained with rhodamine phalloidin following the kit's protocol with minor modifications. Briefly, the treated U251 MG cells were then fixed with 2.5% glutaraldehyde for 10 min at RT, and 0.5% Triton X-100 solution in PBS was introduced to permeabilize the cells by culturing for 5 min at RT. The permeabilized cells were washed thrice with PBS (pH 7.4) and then refilled with 200 μL of 100 nM rhodamine phalloidin. The cells were incubated in dark for 30 min, and then counterstained with DAPI. The samples were observed under an inverted fluorescence microscope equipped with a high-resolution CCD camera.
2.13 Measurement of intracellular ROS
The non-fluorescent probe DCFH–DA can permeate cell membranes and is cleaved by esterase to yield non-fluorescent DCFH. DCFH is oxidized in the presence of ROS to generate the highly fluorescent 2,7-dichlorofluorescein (DCF). The treated U251 MG cells were incubated with 10 μmol L−1 DCFH–DA dye (Keygen Biotech. Co., Ltd., Nanjing China) for 30 min at 37 °C, and then measured using an inverted fluorescence microscope equipped with a high-resolution CCD camera.
2.14 Ca2+ signal analysis of the treated U251 MG cells
The intracellular Ca2+ was monitored using the Ca2+-sensitive dye Fluo-3/AM (Molecular Probes Inc., Eugene, Oregon, USA). The treated U251 MG cells were loaded with 10 μM Fluo-3/AM and 0.02% pluronic acid F-127 (Molecular Probes Inc., Eugene, Oregon, USA) and then incubated for 30 min at 37 °C in a Hank's balanced salt solution. The cells were subsequently suspended in Hank's buffer, and the calcium transient of the treated cells was recorded using an inverted fluorescence microscope after rinsing with a calcium-free Hank's buffer.
2.15 Cell cycle analysis
The cell cycle of the treated U251 MG cells was determined by PI staining and flow cytometry as previously described.38 The U251 MG cells were first treated with NPs, and trypsinized and dissociated into a single-cell suspension, and then fixed by adding 1 mL of 70% ice-cold ethanol, and then incubated at 4 °C overnight. The prepared suspension was centrifuged and resuspended in 1 mL PBS with 50 μg mL−1 PI staining solution containing 20 μg mL−1 RNase, and then incubated for 1 h at 4 °C in dark. The stained cells were then analyzed using a BD FACS Calibur Cytomics Altra flow cytometer (BD Biosciences, San Jose, CA) and Cell Quest software (BD Biosciences).
2.16 In vitro MRI analysis
The U251 MG cells at a density of 1 × 106 cells per well were seeded, and then treated with different concentrations of CS/SPIO NPs and Tf–CS/SPIO NPs ranging from 0 μg mL−1 to 400 μg mL−1 at 37 °C for 6 h. The cells were rinsed thrice with PBS, trypsinized, centrifuged, and resuspended in 1.5 mL PBS (containing 0.5% agarose) in 5 mL Eppendorf tubes for MRI. T2-Weighed images were carried out using a MesoMR60 scanner (Niumag, Shanghai, China) at RT. T2 mapping sequence (TR: 3000 ms, TE: 180 ms, slice width: 5.0 mm, K matrix 192 × 256) was used to measure the transverse relaxation time.
2.17 Characterizations
The prepared NPs, including naked SPIO NPs, CS/SPIO NPs, Tf–CS/SPIO NPs, DOX–CS/SPIO NPs, and Tf–CS/SPIO NPs were morphologically characterized using high-resolution transmission electron microscopy (JEM-2100, Japan). The crystal structure of the SPIO NPs was analyzed through X-ray diffraction spectroscopy (XRD, Philips D/Max-2500, Holland) using a monochromatic X-ray beam with nickel-filtered Cu-Kα radiation. The magnetic properties of the SPIO NPs, CS/SPIO NPs, and Tf–CS/SPIO NPs was measured using a vibrating sample magnetometer (LAKESHORE-7304, USA) by varying H from +1375 Oe to −1375 Oe. Fourier transform infrared spectroscopy (FT-IR, Nicolet NEXUS 670, USA) was performed to record the FT-IR spectra of SPIO NPs, CS/SPIO NPs, and Tf–CS/SPIO NPs. Dried samples were pressed with KBr powder into pellets. Sixty-four scans were signal-averaged in the range of 4000–400 cm−1. The magnetic responsiveness to an external magnetic field of the fabricated CS/SPIO NPs, Tf–CS/SPIO NPs, DOX–CS/SPIO NPs, and Tf–CS/SPIO NPs in DI water was analyzed using a UV-Vis spectrophotometer (UV-1000, Shanghai, China).
2.18 Image acquisition and statistical analyses
Bright-field and fluorescence images were captured by an inverted fluorescence microscope (Eclipse TE 2000-U) equipped with a CCD camera (CV-S3200). The Image-Pro Plus® 6.0 (Media Cyternetics) and SPSS 17.0 (SPSS Inc., Chicago) software were used for image analysis and statistical data analysis, respectively. Quantitative data are expressed as means ± standard deviation for each experiment. All experiments were performed at least for three times, and the representative results are presented.
3 Results and discussion
3.1 Synthesis and characterization of Tf–CS/SPIO NPs
In the present study, the tumor-specific targeting Tf–CS/SPIO NPs was prepared in a four-step process (Scheme 2), including (i) synthesis of core nanocarriers (SPIO NPs, i.e., γ-Fe2O3) followed with CS modification to prepare CS/SPIO NPs; (ii) formation of CS/SPIO–SMCC by crosslinking CS/SPIO NPs with sulfo-SMCC; (iii) activation of Tf with SH by thiolation through Traut's reagent; (iv) conjugation of CS/SPIO–SMCC with Tf–SH to obtain Tf–CS/SPIO NPs.
The prepared SPIO NPs and Tf–CS/SPIO NPs were then characterized (Fig. 1). The TEM images showed that the nano-sized SPIO NPs were morphologically uniform with an averaged diameter ranging from 10 nm to 15 nm (Fig. 1A). The prepared Tf–CS/SPIO NPs also displayed uniformly round microspheres with a diameter of approximately 60 nm, and demonstrated a good dispersion (Fig. 1B). Fig. 1C showed that Tf–CS/SPIO NPs were successfully modified with RBITC. The XRD pattern displayed six characteristic peaks (220), (311), (400), (422), (511), and (440), which were observed in SPIO NPs, CS-MNPs, and Tf–CS/SPIO NPs. These results indicated that incorporation of Tf and CS into SPIO NPs did not influence γ-Fe2O3 crystallization (Fig. S1A–C†).
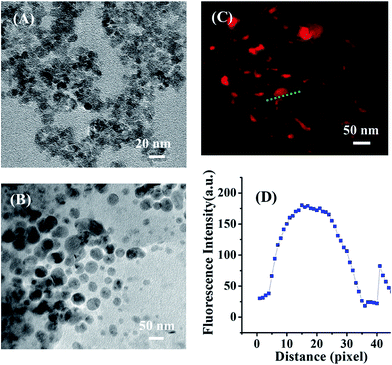 |
| Fig. 1 TEM image of SPIO NPs (A) and Tf–CS/SPIO NPs (B), the fluorescence image of RBITC modified Tf–CS/SPIO NPs (C), and (d) their fluorescence intensities corresponding to the line profile graphs analyzed using Image-Pro Plus® 6.0 software (D). | |
The composition of DOX–Tf–CS/SPIO NPs was analyzed by FT-IR (Fig. 2A), which showed that the C–N stretching vibration of amide groups was located at 1385 cm−1, the C–O bond vibration of ether groups was located at 1097 cm−1, and the characteristic band (Fe–O) of SPIO NPs was located at 591 cm−1. The peaks at 2366 and 2929 cm−1 were attributed to the stretching vibrations of methylene. The characteristic band of –COOH was also observed at 3411 cm−1. These data indicated that the SPIO NPs were modified with CS and Tf, and the anti-tumor drug DOX was successfully loaded within Tf–CS/SPIO NPs.
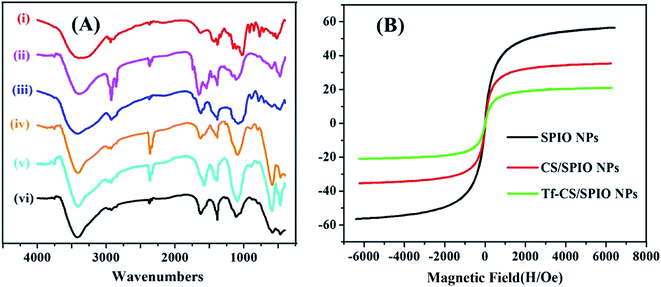 |
| Fig. 2 (A) FT-IR spectra of CS (i), Tf (ii), DOX (iii), DOX–Tf–CS/SPIO NPs (iv), CS/SPIO NPs (v), SPIO NPs (vi). (B) The magnetic hysteresis loop of SPIO NPs, CS/SPIO NPs, and Tf–CS/SPIO NPs at 300 K. | |
The magnetization curves of the prepared SPIO NPs, CS/SPIO NPs, and Tf–CS/SPIO NPs were shown in Fig. 2B, and demonstrated as a symmetrical hysteresis loop, indicating that these NPs would be magnetized in the presence of a magnetic field and the removal of magnetic field results in the presence of a minimal residual magnetization within the particles.39 Moreover, the saturation magnetization of SPIO NPs, CS/SPIO NPs, and Tf–CS/SPIO NPs were estimated to be 56.06, 35.34, and 20.94 emu g−1, respectively. The coercivity of these NPs is nearly zero.
3.2 Magnetic responsiveness, stability and drug loading
Magnetic-responsive aggregation and redispersion of Tf–CS/SPIO NPs in different media, including DI water, PBS (pH 7.4), and RPMI-1640 culture medium, were determined under an external magnetic field and gravity conditions at different time points (ESI, Fig. S2A–D†). The results indicated that the Tf–CS/SPIO NPs exhibited excellent magnetic responsive properties and easily recycled under an external magnetic field. In addition, the Tf–CS/SPIO NPs were completely redispersed by gentle shaking once the external magnet was removed. These performances indicated that the fabricated Tf–CS/SPIO NPs were probably very suitable for drug delivery. The loading capacity was measured the optical density of the supernatant. The standard curve equation of DOX was y = 0.007 + 0.012x (ESI, Fig. S3†). Therefore, DOX drug loading in DOX–Tf–CS/SPIO NPs was 1.22 mg/100 mg (1.22%) and the encapsulation efficiency was 99.08%.
3.3 Cellular uptake
Cellular uptake of Tf–CS/SPIO NPs was analyzed by incubating the fluorescent RBITC–Tf–CS/SPIO NPs with U251 MG cells for 4 h, and the results showed that Tf–CS/SPIO NPs have entered into the U251 MG cells, as the red fluorescence was located around the DAPI-stained nuclei of the U251 MG cells (Fig. 3A). Compared with the cells treated with RBITC–CS/SPIO NPs, a much stronger fluorescence was detected in RBITC–Tf–CS/SPIO NPs treated U251 MG cells, indicating conjugation of Tf could improve internalization efficiency in the targeted cells. Besides, the cellular uptake of Tf–CS/SPIO NPs was also quantified by AAS analysis, and the data showed that the iron in U251 MG cells were 6.4 pg Fe per cell for CS/SPIO NPs and 49.3 pg Fe per cell for Tf–CS/SPIO NPs (Fig. 3B). These results demonstrated that the Tf–CS/SPIO NPs could be efficiently uptaken by U251 MG cells.
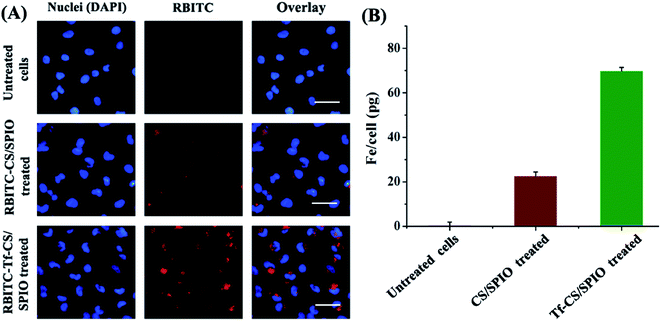 |
| Fig. 3 (A) Visualization of RBITC–CS/SPIO NPs and RBITC–Tf–CS/SPIO NPs treated U251 MG cells after 4 hours' incubation (concentration: 100 μg mL−1). Nuclei were located by counterstaining with DAPI (blue). Scale bar = 100 μm. (B) The iron content in U251 MG cells indicated an uptake of RBITC–CS/SPIO NPs and RBITC–Tf–CS/SPIO NPs. | |
3.4 In vitro cytotoxicity
MTT assay was a useful approach to estimate the proliferative ability of cells.40,41 The cytotoxicity was determined by incubation U251 MG cells with different NPs including CS/SPIO NPs, Tf–CS/SPIO NPs, DOX–CS/SPIO NPs, and DOX Tf–CS/SPIO NPs. The results showed that the viability of the treated U251 MG cells was not obviously affected by the CS/SPIO NPs and Tf–CS/SPIO NPs, but cell viability was obviously reduced when anti-tumor drug DOX was loaded onto CS/SPIO NPs and Tf–CS/SPIO NPs (Fig. 4). Moreover, the DOX–Tf–CS/SPIO NPs could most effectively decrease proliferative capacity of treated U251 MG cells.
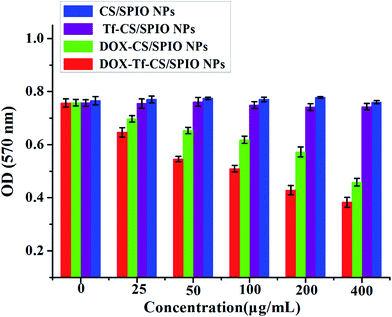 |
| Fig. 4 The growth state of the treated U251 MG cells with CS/SPIO NPs, Tf–CS/SPIO NPs, DOX–CS/SPIO NPs and DOX–Tf–CS/SPIO NPs for 48 h. | |
3.5 Cell viability
The PI and FDA double staining was performed to detect the cell viability and cell death of the treated U251 MG cells. The results showed the viability of the U251 MG cells remain almost intact and only a few dead cells were observed when treated with CS/SPIO NPs and Tf–CS/SPIO NPs compared with that of the untreated U251 MG cells (Fig. 5A and B). In contrast, a higher number of U251 MG cells showed reduced viability after DOX–CS/SPIO NP and DOX–Tf–CS/SPIO NP treatments (Fig. 5A and B). Therefore, these data indicated that DOX–Tf–CS/SPIO NPs could efficiently reduce the viability of U251 MG cells.
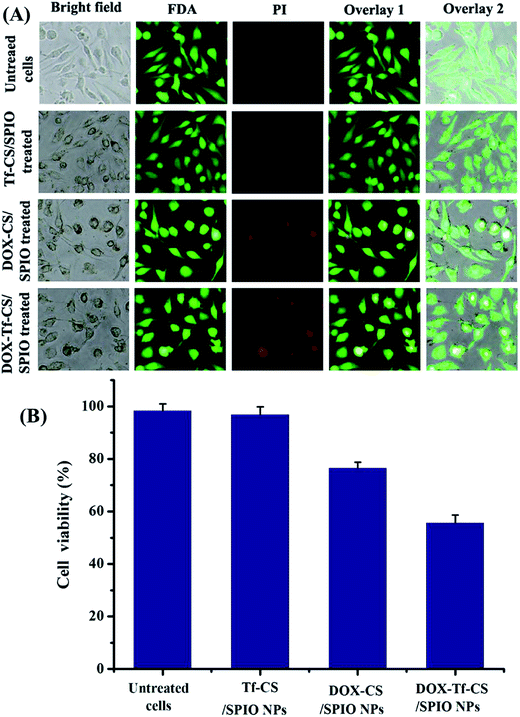 |
| Fig. 5 Viability assessment of the treated U251 MG cells. The U251 MG cells were treated with various nanocarrier complex (100 μg mL−1) for 48 h respectively, and untreated U251 MG cells served as controls. Scale bar = 100 μm. | |
3.6 Apoptosis assay
Cell apoptosis is commonly used as a behavior of the improved treatment for targeted tumor cells in therapy,42,43 which is generally characterized by distinct morphological changes, including blebbing, cell shrinkage, nuclear fragmentation, chromatin condensation, and chromosomal DNA fragmentation.44,45 Thus, to visualize nuclear fragmentation of apoptotic cells, the U251 MG cells were stained with Hoechst H33258, a fluorescent dye that binds to the AT-rich regions of DNA and allows detection and relative quantification of DNA of apoptotic cells.46 The results showed that the DOX–Tf–CS/SPIO NPs treated U251 MG cells displayed chromatin condensation, nuclear peripheral aggregation, and nuclear fragmentation (Fig. 6A and B). Additionally, the TUNEL assay showed that more apoptotic cells (TUNEL-positive) were detected as higher concentrations of DOX–Tf–CS/SPIO NPs applied in cells (Fig. 6C and D). Moreover, compared DOX–CS/SPIO NPs, the DOX–Tf–CS/SPIO NPs could more efficiently induce cell apoptosis (Fig. 6E).
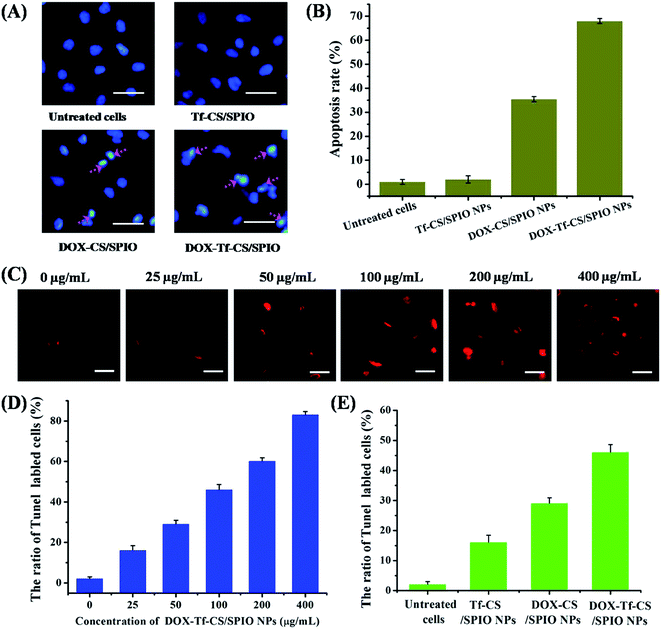 |
| Fig. 6 Apoptosis assay of the treated U251 MG cells using Hoechst H33258 staining and TUNEL assay. The fluorescence images (A) of the treated U251 MG cells with various nanocarriers (100 μg mL−1), and the statistic analysis of apoptotic rate for treated U251 MG cells (B). (C) The TUNEL assay fluorescence images of treated U251 MG cells with various concentrations of DOX–Tf–CS/SPIO NPs for 24 h; (D and E) statistic analyses of TUNEL-positive cells with different treatments of various nanocarriers. Scale bar = 50 μm. | |
To determine the expression pattern of the apoptosis-related genes in the DOX–Tf–CS/SPIO NP-treated U251 MG cells, we used Western blot to analyze the expression of the apoptosis-related proteins Caspase 3 and Bax, as well as the anti-apoptotic proteins Bcl-2 and Survivin. The results showed that expression of Caspase 3 and Bax increased with increasing DOX–Tf–CS/SPIO NP concentration used in cell treatment, whereas that of the anti-apoptotic proteins, such as Bcl-2 and survivin, decreased (Fig. 7A and B). These data indicated that DOX–Tf–CS/SPIO NPs triggered apoptosis and cell death in treated U251 MG cells by upregulating the apoptotic proteins and downregulating the anti-apoptotic proteins (Fig. 7C).
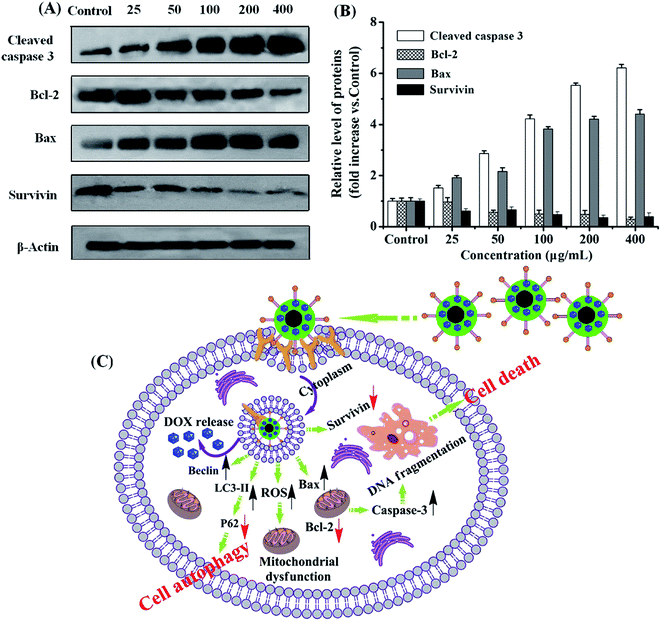 |
| Fig. 7 Western blot analysis of apoptosis-related proteins in treated U251 MG cells with DOX–Tf–CS/SPIO NPs of different concentrations (A and B, 25–400 μg mL−1). Schematic representation of DOX–Tf–CS/SPIO NPs induced apoptosis and autophagy (C). | |
3.7 Autophagy assay
Autophagy is a programmed cell death initiated by formation of autophagosomes.47,48 During autophagosome formation, the microtubule-associated protein light chain 3 I (LC3-I) is conjugated to phosphatidylamine to form LC3–phosphatidylamine (LC3-II), and the increasing ratio of LC3-II to LC3-I levels reflects the activation of autophagy.49,50 Therefore, the cytoplasmic protein LC3, being a hallmark of autophagy, can be revealed by its anti-LC3A/B antibody. In addition, formation of AVOs is a characteristic of autophagy and is usually detected by AO staining in cells.51 The results showed that, compared with untreated cells, the cells treated with DOX–CS/SPIO NPs and DOX–Tf–CS/SPIO NPs displayed stronger AO staining signal and anti-LC3 puncta, indicating accumulation of AVOs and LC3 in their cytoplasm (Fig. 8). Moreover, the DOX–Tf–CS/SPIO NP-treated U251 MG cells produced higher number of endogenous LC3 puncta than DOX–CS/SPIO NP-treated cells. Western blot analysis was performed to evaluate the conversion of LC3-I into LC3-II, and the results showed that the targeting DOX–Tf–CS/SPIO NP-treated U251 MG cells displayed enhanced expression of Beclin 1 and produced LC3-II in a dose-dependent manner (Fig. 9A and B). These data collectively suggested that DOX–Tf–CS/SPIO NPs can induce autophagy in U251 MG cells.
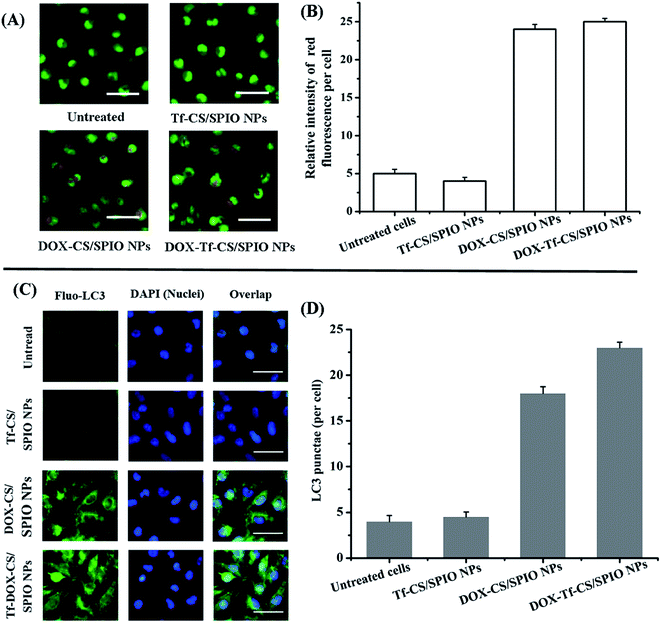 |
| Fig. 8 (A) AO staining in the treated U251 MG cells. Scale bar = 100 μm. (B) In contrast with the untreated U251 MG cells as controls, no AO stain was obviously observed in the cells treated with Tf–CS/SPIO NPs, but staining was clearly detected in the DOX–CS/SPIO NPs and DOX–Tf–CS/SPIO NPs treated U251 MG cells for 24 h. (C) Accumulated cytoplasmic LC3 signal as a hallmark of autophagy in treated U251 MG cells. Scale bar = 50 μm. (D) The untreated U251 MG cells were used as controls, and in contrast with cells treated with Tf–CS/SPIO NPs, the statistical analysis showed that the LC3 signal was obviously detected in DOX–CS/SPIO NPs and DOX–Tf–CS/SPIO NPs treated cells. | |
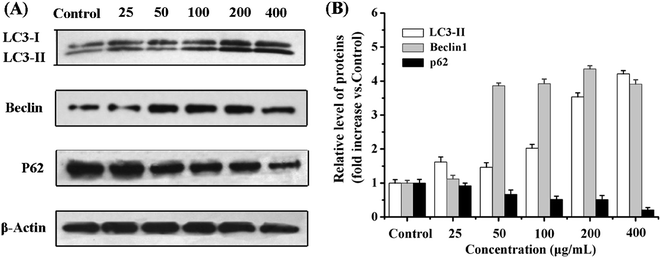 |
| Fig. 9 Western blot analysis of autophagy-associated proteins in U251 MG cells treated with DOX–Tf–CS/SPIO NPs (25–400 μg mL−1) for 24 h (A), and the histogram represents the relative level of proteins expressed in treated cells (B). | |
In addition, p62 is involved in autophagosome formation and constitutively degraded by the autophagic pathway through specific binding to LC3; a decrease in the level of p62 thus reflects an autophagy flux.52,53 Western blot analysis showed that the expression level of p62 decreased with increasing DOX–Tf–CS/SPIO NP concentrations (25–400 μg mL−1) treated with U251 MG cells (Fig. 9A and B).
3.8 Cell cycle analysis
DOX could inhibit synthesis of nucleic acid, mainly RNA synthesis, in tumor cells. DOX enters into the tumor cells, severely affecting the G2 phase. The effect of DOX–Tf–CS/SPIO NPs on cell cycle distribution in U251 MG cells was studied by flow cytometric analysis. The results showed that compared with that in untreated U251 MG cells, an increasing number of U251 MG cells were arrested in the G2 phase after treatment of increasing dosage of DOX–Tf–CS/SPIO NPs (Fig. 10A and B). In addition, compared with the CS/SPIO NPs, the targeting DOX–Tf–CS/SPIO NPs could more efficiently induce changes in cell cycle distribution and arrest cell division in the G2 phase in treated U251 MG cells (Fig. 10B).
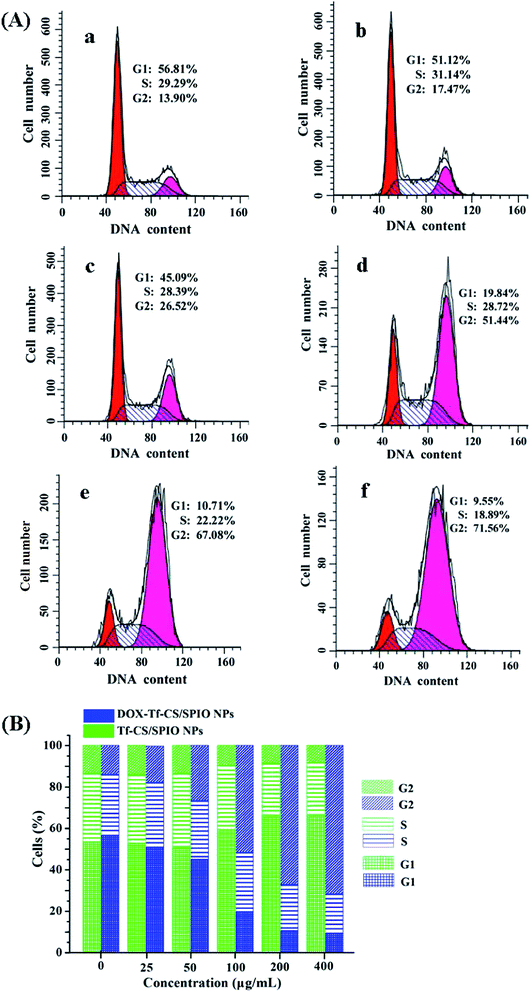 |
| Fig. 10 Cell cycle distribution of the treated cells. Various concentration (0–400 μg mL−1) DOX–Tf–CS/SPIO NPs-treated U251 MG cells were cultured for 48 h (A, b–f). Untreated U251 MG cells were used as controls (a). Effect of DOX–Tf–CS/SPIO and Tf–CS/SPIO NPs on cell cycle in U251 MG cells evaluated by calculating the percentage of cells in each phase from flow cytometric data (B). | |
3.9 MRI
The potential of Tf–CS/SPIO NPs was evaluated as a targeted MR contrast agent in glioma U251 MG cells. The results showed that the targeting Tf–CS/SPIO NP-incubated cells demonstrated a significantly negative contrast enhancement (signal darkening) compared with the cells incubated with CS/SPIO NPs (Fig. 11), which was probably because the efficient Tf-mediated endocytosis leads to a distinguishable darkening of MR images of the cells incubated with Tf–CS/SPIO NPs.
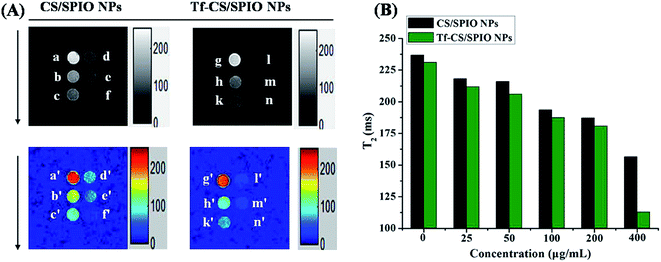 |
| Fig. 11 T2-Weighted MR images (a–f @ g–n) and their color maps (a′–f′ @ g′–n′) of U251 MG cells after incubation with the various concentrations of CS/SPIO NPs and Tf–CS/SPIO NPs (0, 25, 50, 100, 200, 400 μg mL−1) for 4 h (A). (B) T2 assay for various concentrations of CS/SPIO NPs and Tf–CS/SPIO NPs. | |
4 Conclusion
In the present study, the SPIO NPs-based multimodal theranostic nanoplatform was firstly developed with multi-purpose properties including magnetism, fluorescence, anti-tumor drug DOX loading, and MRI, and then delivered into U251 MG cells to determine their biological effects. The results showed that the DOX–CS/SPIO NPs could more efficiently inhibit cell proliferation and induce apoptosis and autophagy in the treated U251 MG cells, when conjugated with tumor-specific ligand Tf. Therefore, these fabricated Tf–CS/SPIO NPs as a core nanocarriers may offer more opportunities allowing for a dual fluorescence and MR-based imaging to real-time monitor delivery efficacy of anti-tumor drugs in human brain tumor treatments.
Acknowledgements
This work was supported by the National Natural Science Foundation of China (No. 314 008 55), the Basal Research Fund of Henan University of Technology (No. 2014 YWQ Q15), the Technology Research and Development Support Funds Project of Zhengzhou City (No. 153 PXX CY 184).
References
- F. E. Bleeker, R. J. Molenaar and S. Leenstra, Recent advances in the molecular understanding of glioblastoma, J. Neuro-Oncol., 2012, 108, 11–27 CrossRef CAS PubMed.
- D. R. Johnson and B. P. O'Neill, Glioblastoma survival in the United States before and during the temozolomide era, J. Neuro-Oncol., 2012, 107, 359–364 CrossRef CAS PubMed.
- CBTRUS, Statistical Report, 1998–2002: Central Brain Tumor Registry of the United States, 2005 Search PubMed.
- W. Chen, D. Wang, X. Du, Y. He, S. Chen, Q. Shao, C. Ma, B. Huang, A. Chen, P. Zhao, X. Qu and X. Li, Glioma cells escaped from cytotoxicity of temozolomide and vincristine by communicating with human astrocytes, Med. Oncol., 2015, 32, 43 CrossRef PubMed.
- W. Taal, J. E. Bromberg and M. J. van den Bent, Chemotherapy in glioma, CNS Oncol., 2015, 4, 179–192 CrossRef CAS PubMed.
- M. Yu, F. Guo, F. Tan and N. Li, Dual-targeting nanocarrier system based on thermosensitive liposomes and gold nanorods for cancer thermo-chemotherapy, J. Controlled Release, 2015, 215, 91–100 CrossRef CAS PubMed.
- R. J. Gibson and D. M. Keefe, Cancer chemotherapy-induced diarrhoea and constipation: mechanisms of damage and prevention strategies, Support. Care Canc., 2006, 14, 890–900 CrossRef PubMed.
- S. E. Baek, K. H. Lee, Y. S. Park, D. K. Oh, S. Oh, K. S. Kim and D. E. Kim, RNA aptamer-conjugated liposome as an efficient anticancer drug delivery vehicle targeting cancer cells in vivo, J. Controlled Release, 2014, 196, 234–242 CrossRef CAS PubMed.
- K. Zheng, R. Li, X. Zhou, P. Hu, Y. Zhang, Y. Huang, Z. Chen and M. Huang, Dual actions of albumin packaging and tumor targeting enhance the antitumor efficacy and reduce the cardiotoxicity of doxorubicin in vivo, Int. J. Nanomed., 2015, 10, 5327–5342 CAS.
- A. Jain, A. Jain, N. K. Garg, R. K. Tyagi, B. Singh, O. P. Katare, T. J. Webster and V. Soni, Surface engineered polymeric nanocarriers mediate the delivery of transferrin–methotrexate conjugates for an improved understanding of brain cancer, Acta Biomater., 2015, 24, 140–151 CrossRef CAS PubMed.
- S. Ruan, X. Cao, X. Cun, G. Hu, Y. Zhou, Y. Zhang, L. Lu, Q. He and H. Gao, Matrix metalloproteinase-sensitive size-shrinkable nanoparticles for deep tumor penetration and pH triggered doxorubicin release, Biomaterials, 2015, 60, 100–110 CrossRef CAS PubMed.
- X. Cun, J. Chen, S. Ruan, L. Zhang, J. Wan, Q. He and H. Gao, A novel strategy through combining iRGD peptide with tumor-microenvironment-responsive and multistage nanoparticles for deep tumor penetration, ACS Appl. Mater. Interfaces, 2015, 7, 27458–27466 CAS.
- A. R. Sasikala, A. R. Unnithan, Y. H. Yun, C. H. Park and C. S. Kim, An implantable smart magnetic nanofiber device for endoscopic hyperthermia treatment and tumor-triggered controlled drug release, Acta Biomater., 2016, 31, 122–133 CrossRef CAS PubMed.
- G. Unsoy, R. Khodadust, S. Yalcin, P. Mutlu and U. Gunduz, Synthesis of doxorubicin loaded magnetic chitosan nanoparticles for pH responsive targeted drug delivery, Eur. J. Pharm. Sci., 2014, 62, 243–250 CrossRef CAS PubMed.
- J. Zhang, S. Zhao, M. Zhu, Y. Zhu, Y. Zhang, Z. Liu and C. Zhang, 3D-printed magnetic Fe3O4/MBG/PCL composite scaffolds with multifunctionality of bone regeneration, local anticancer drug delivery and hyperthermia, J. Mater. Chem. B, 2014, 2, 7583–7595 RSC.
- F. M. Kievit and M. Zhang, Surface engineering of iron oxide nanoparticles for targeted cancer therapy, Acc. Chem. Res., 2011, 44, 853–862 CrossRef CAS PubMed.
- D. R. Elias, Z. Cheng and A. Tsourkas, An intein-mediated site-specific click conjugation strategy for improved tumor targeting of nanoparticle systems, Small, 2010, 6, 2460–2468 CrossRef CAS PubMed.
- N. Schleich, C. Po, D. Jacobs, B. Ucakar, B. Gallez, F. Danhier and V. Préat, Comparison of active, passive and magnetic targeting to tumors of multifunctional paclitaxel/SPIO-loaded nanoparticles for tumor imaging and therapy, J. Controlled Release, 2014, 194, 82–91 CrossRef CAS PubMed.
- Y. Wang, R. Zhao, S. Wang, Z. Liu and R. Tang, In vivo dual-targeted chemotherapy of drug resistant cancer by rationally designed nanocarrier, Biomaterials, 2016, 75, 71–81 CrossRef CAS PubMed.
- Y. Yang and C. Yu, Advances in silica based nanoparticles for targeted cancer therapy, Nanomedicine, 2016, 12, 317–332 CAS.
- Y. Mo, H. Wang, J. Liu, Y. Lan, R. Guo, Y. Zhang, W. Xue and Y. Zhang, Controlled release and targeted delivery to cancer cells of doxorubicin from polysaccharide-functionalised single-walled carbon nanotubes, J. Mater. Chem. B, 2015, 3, 1846–1855 RSC.
- N. Schleich, C. Po, D. Jacobs, B. Ucakar, B. Gallez, F. Danhier and V. Préat, Comparison of active, passive and magnetic targeting to tumors of multifunctional paclitaxel/SPIO-loaded nanoparticles for tumor imaging and therapy, J. Controlled Release., 2014, 194, 82–91 CrossRef CAS PubMed.
- G. Liu, H. Shen, J. Mao, L. Zhang, Z. Jiang, T. Sun, Q. Lan and Z. Zhang, Transferrin modified graphene oxide for glioma-targeted drug delivery: in vitro and in vivo evaluations, ACS Appl. Mater. Interfaces, 2013, 5, 6909–6914 CAS.
- W. Guo, A. Li, Z. Jia, Y. Yuan, H. Dai and H. Li, Transferrin modified PEG–PLA–resveratrol conjugates: in vitro and in vivo studies for glioma, Eur. J. Pharmacol., 2013, 718, 41–47 CrossRef CAS PubMed.
- C. Zhao, X. Liu, J. Liu, Z. Yang, X. Rong, M. Li, X. Liang and Y. Wu, Transferrin conjugated poly(γ-glutamic acid-maleimide-co-L-lactide)–1,2-dipalmitoylsn-glycero-3-phosphoethanolamine copolymer nanoparticles for targeting drug delivery, Colloids Surf., B, 2014, 123, 787–796 CrossRef CAS PubMed.
- Q. Xu, Y. Liu, S. Su, W. Li, C. Chen and Y. Wu, Anti-tumor activity of paclitaxel through dual-targeting carrier of cyclic RGD and transferrin conjugated hyperbranched copolymer nanoparticles, Biomaterials, 2012, 33, 1627–1639 CrossRef CAS PubMed.
- Y. Cui, Q. Xu, P. K. Chow, D. Wang and C. H. Wang, Transferrin-conjugated magnetic silica PLGA nanoparticles loaded with doxorubicin and paclitaxel for brain glioma treatment, Biomaterials, 2013, 34, 8511–8520 CrossRef CAS PubMed.
- J. Lin, Y. Li, Y. Li, H. Wu, F. Yu, S. Zhou, L. Xie, F. Luo, C. Lin and Z. Hou, Drug/dye-loaded, multifunctional PEG–chitosan–iron oxide nanocomposites for methotraxate synergistically self-targeted cancer therapy and dual model imaging, ACS Appl. Mater. Interfaces, 2015, 7, 11908–11920 CAS.
- X. Yang, J. J. Grailer, I. J. Rowland, A. Javadi, S. A. Hurley, V. Z. Matson, D. A. Steeber and S. Gong, Multifunctional stable and pH-responsive polymer vesicles formed by heterofunctional triblock copolymer for targeted anticancer drug delivery and ultrasensitive MR imaging, ACS Nano, 2010, 4, 6805–6817 CrossRef CAS PubMed.
- S. Wang, W. Yang, H. Du, F. Guo, H. Wang, J. Chang, X. Gong and B. Zhang, Multifunctional reduction-responsive SPIO&DOX-loaded PEGylated polymeric lipid vesicles for magnetic resonance imaging-guided drug delivery, Nanotechnology, 2016, 27, 165101 CrossRef PubMed.
- B. M. Gruber, E. L. Anuszewska, I. Bubko, A. Gozdzik, W. Priebe and I. Fokt, Relationship between topoisomerase II–DNA cleavable complexes, apoptosis and cytotoxic activity of anthracyclines in human cervix carcinoma cells, Anticancer Res., 2005, 25, 2193–2198 CAS.
- L. P. Swift, A. Rephaeli, A. Nudelman, D. R. Phillips and S. M. Cutts, Doxorubicin–DNA adducts induce a non-topoisomerase II-mediated form of cell death, Cancer Res., 2006, 66, 4863–4871 CrossRef CAS PubMed.
- S. Qu, H. Yang, D. Ren, S. Kan, G. Zou, D. Li and M. Li, Magnetite nanoparticles prepared by precipitation from partially reduced ferric chloride aqueous solutions, J. Colloid Interface Sci., 1999, 215, 190–192 CrossRef CAS PubMed.
- Y. K. Sun, M. Ma, Y. Zhang and N. Gu, Synthesis of nanometer-size maghemite particles from magnetite, Colloids Surf., A, 2004, 245, 15–19 CrossRef CAS.
- X. Wang, L. Wang, X. Tan, H. Zhang and G. Sun, Construction of doxorubicin-loading magnetic nanocarriers for assaying apoptosis of glioblastoma cells, J. Colloid Interface Sci., 2014, 436, 267–275 CrossRef CAS PubMed.
- X. Wang, F. Wei, S. Yan, H. Zhang, X. Tan, L. Zhang, G. Zhou, L. Cui, C. Li, L. Wang and Y. Li, Innovative fluorescent magnetic albumin microbead-assisted cell labeling and intracellular imaging of glioblastoma cells, Biosens. Bioelectron., 2014, 54, 55–63 CrossRef CAS PubMed.
- B. W. Kristensen, H. Noer, J. B. Gramsbergen, J. Zimmer and J. Noraberg, Colchicine induces apoptosis in organotypic hippocampal slice cultures, Brain Res., 2003, 964, 264–278 CrossRef CAS PubMed.
- X. Wang, L. Zhu, X. Hou, L. Wang and S. Yin, Polyethylenimine mediated magnetic nanoparticles for combined intracellular imaging, siRNA delivery and anti-tumor therapy, RSC Adv., 2015, 5, 101569 RSC.
- J. Wang, X. Wang, L. Ren, Q. Wang, L. Li, W. Liu, Z. Wan, L. Yang, P. Sun, L. Ren, M. Li, H. Wu, J. Wang and L. Zhang, Conjugation of biomolecules with magnetic protein microspheres for the assay of early biomarkers associated with acute myocardial infarction, Anal. Chem., 2009, 81, 6210–6217 CrossRef CAS PubMed.
- M. E. Khosroshahi, H. A. Rezvani, H. Keshvari, S. Bonakdar and M. Tajabadi, Evaluation of cell viability and T2 relaxivity of fluorescein conjugated SPION-PAMAM third generation nanodendrimers for bioimaging, Mater. Sci. Eng., C, 2016, 62, 544–552 CrossRef CAS PubMed.
- J. H. Almaki, R. Nasiri, A. Idris, F. A. Majid, M. Salouti, T. S. Wong, S. Dabagh, M. Marvibaigi and N. Amini, Synthesis, characterization and in vitro evaluation of exquisite targeting SPIONs-PEG-HER in HER2+ human breast cancer cells, Nanotechnology, 2016, 27, 105601 CrossRef PubMed.
- L. Yang, Z. Wang, J. Wang, W. Jiang, X. Jiang, Z. Bai, Y. He, J. Jiang, D. Wang and L. Yang, Doxorubicin conjugated functionalizable carbon dots for nucleus targeted delivery and enhanced therapeutic efficacy, Nanoscale, 2016, 8, 6801–6809 RSC.
- X. Fu, Y. Yang, X. Li, H. Lai, Y. Huang, L. He, W. Zheng and T. Chen, RGD peptide-conjugated selenium nanoparticles: antiangiogenesis by suppressing VEGF-VEGFR2-ERK/AKT pathway, Nanomedicine, 2016, 1549–9634, 00082–00084 Search PubMed.
- E. Hollville and S. J. Martin, Measuring apoptosis by microscopy and flow cytometry, Current Protocols in Immunology, 2016, 112, 14 Search PubMed.
- D. V. Krysko, T. Vanden Berghe, E. Parthoens, K. D'Herde and P. Vandenabeele, Methods for distinguishing apoptotic from necrotic cells and measuring their clearance, Methods Enzymol., 2008, 442, 307–341 Search PubMed.
- K. Chamaon, J. Stojek, D. Kanakis, S. Braeuninger, E. Kirches, G. Krause, C. Mawrin and K. Dietzmann, Micromolar concentrations of 2-methoxyestradiol kill glioma cells by an apoptotic mechanism, without destroying their microtubule cytoskeleton, J. Neuro-Oncol., 2005, 72, 11–16 CrossRef CAS PubMed.
- L. Ouyang, Z. Shi, S. Zhao, F. T. Wang, T. T. Zhou, B. Liu and J. K. Bao, Programmed cell death pathways in cancer: a review of apoptosis, autophagy and programmed necrosis, Cell Proliferation, 2012, 45, 487–498 CrossRef CAS PubMed.
- W. Bursch, K. Hochegger, L. Torok, B. Marian, A. Ellinger and R. S. Hermann, Autophagic and apoptotic types of programmed cell death exhibit different fates of cytoskeletal filaments, J. Cell Sci., 2000, 113, 1189–1198 CAS.
- P. Giménez-Xavier, R. Francisco, A. F. Santidrián, J. Gil and S. Ambrosio, Effects of dopamine on LC3-II activation as a marker of autophagy in a neuroblastoma cell model, Neurotoxicology, 2009, 30, 658–665 CrossRef PubMed.
- H. Y. Park, J. H. Kim and C. K. Park, Activation of autophagy induces retinal ganglion cell death in a chronic hypertensive glaucoma model, Cell Death Dis., 2012, 3, e290 CrossRef PubMed.
- Y. Li, L. Zhang, J. Zhou, S. Luo, R. Huang, C. Zhao and A. Diao, Nedd4 E3 ubiquitin ligase promotes cell proliferation and autophagy, Cell Proliferation, 2015, 48, 338–347 CrossRef CAS PubMed.
- Y. Ragazzoni, M. Desideri, C. Gabellini, T. De Luca, S. Carradori, D. Secci, R. Nescatelli, A. Candiloro, M. Condello, S. Meschini, D. Del Bufalo and D. Trisciuoglio, The thiazole derivative CPTH6 impairs autophagy, Cell Death Dis., 2013, 4, e524 CrossRef CAS PubMed.
- J. M. Park, S. Huang, T. T. Wu, N. R. Foster and F. A. Sinicrope, Prognostic impact of Beclin 1, p62/sequestosome 1 and LC3 protein expression in colon carcinomas from patients receiving 5-fluorouracil as adjuvant chemotherapy, Cancer Biol. Ther., 2013, 14, 100–107 CrossRef CAS PubMed.
Footnote |
† Electronic supplementary information (ESI) available. See DOI: 10.1039/c6ra20903c |
|
This journal is © The Royal Society of Chemistry 2016 |