DOI:
10.1039/C6RA20625E
(Paper)
RSC Adv., 2016,
6, 108662-108667
Exonuclease III-assisted substrate fragment recycling amplification strategy for ultrasensitive detection of uranyl by a multipurpose DNAzyme†
Received
16th August 2016
, Accepted 3rd November 2016
First published on 4th November 2016
Abstract
We report on a novel and highly sensitive strategy for UO22+ detection. The designed single-labeled hairpin signal probe (SSP) was employed as a reporter, and a multipurpose RNA-cleaving DNAzyme as a target recognition element, catalytic DNAzyme and the primer of substrate fragment recycling amplification. The presence of UO22+ results in the substrate strand in DNAzyme being cleaved at the RNA site, releasing short substrate fragments. The fragment that is complementary partially with SSP could open the hairpin of SSP to form a double-stranded DNA with recessed 3′-termini, which could be selectively digested by exonuclease III. After the double-stranded DNA is fully consumed, the substrate fragment is released. This one could hybridize with another SSP to initiate the next round of digestion by exonuclease III. The recycling of substrate fragments can be repeated multiple times, resulting in the accumulation of the more 6-carboxyfluorescein in the solution along with an amplified fluorescence signal. By this strategy, we achieved hitherto an unreported UO22+ detection limit of 2.4 pM. Therefore, this novel exonuclease III-amplified strategy has great potential for highly sensitive and selective quantification of UO22+ in biomedical research and environmental science fields.
Introduction
Uranium is a radioactive and toxic heavy metal that exists ubiquitously in the environment.1–3 The uranyl ion (UO22+) is the most stable form of uranium in water, which is highly bioavailable and poses the greatest risk to human health.3,4 Therefore, the development of an ultrasensitive and selective method for UO22+ assay is highly desirable in environmental protection, as well as disease prevention and treatment. To meet this goal, Lu and co-workers reported a catalytic beacon sensor for detecting uranyl with a detection limit of 45 pM,5 which is much lower than the toxic UO22+ level in drinking water defined by the U.S. Environmental Protection Agency. Furthermore, a number of biosensors based on an uranyl-specific DNAzyme have also been reported for UO22+ assay.6–11 Although these methods have made great advances toward the UO22+ assay, the further improvement of the sensitivity for detecting UO22+ with extremely low amount is still highly necessary. This is due to the concentration of UO22+ often being at a very low level in the early stage of disease. In recent years, nuclease-assisted signal amplification strategy has been proposed to boost detection sensitivity of biosensors.12–16 Among these enzymes, exonuclease III (Exo III) has been widely used for amplified detection of DNA or other targets.16–22 Unlike nicking endonucleases, Exo III does not require a specific recognition site and can catalyze stepwise removal of mononucleotides from the blunt or recessed 3′-OH termini of double-stranded DNA (dsDNA).23–28 It is not active on 3′-overhang ends of dsDNA or single-stranded DNA (ssDNA). This feature of selective nucleotide digestion has been utilized to “recycle” target molecules, which can enhance significantly the detectable signal level for target molecules. Thus, Exo III provides a more versatile platform for amplification detection of analytes. However, so far little method has been reported for the detection of UO22+ based on both RNA-cleaving DNAzyme and Exo III as a signal amplification strategy. Therefore, to design a new system for sensing of uranyl based on DNAzyme and Exo III is highly necessary to satisfy the requirements in biological research and medical diagnosis. Fluorescence technology is widely employed in the detection of metal ions owing to its advantages of having high sensitivity, selectivity and reproducibility.29 The selection of the fluorescent probes is a critical element for achieving this goal. Molecular beacon (MB) has become a mainstay for DNA detection because it can rapidly and specifically bind given nucleic acid sequence in homogeneous solution.30 Up until now, most of the reported MB biosensors need to be labeled with a donor of fluorophore and an acceptor of quencher.31 This double labeling would result in some problems such as high cost and low yield. Therefore, the fabrication of a single-labeled MB (signal probe) for the sensitive detection of UO22+ has become highly focused.
Herein, we report a highly sensitive strategy for biosensing of UO22+. This strategy integrates two processes, namely, the catalytic cleavage of the substrate strand in RNA-cleaving DNAzyme by UO22+, and Exo III-assisted substrate fragment recycling amplification. A single-labeled hairpin signal probe (SSP) was rationally designed as a reporter (Scheme 1). The presence of uranyl results in SSP being opened by hybridizing with the cleaved substrate fragment from RNA-cleaving DNAzyme, accompanied by the formation of a DNA duplex (dsDNA) with a recessed 3′-terminus. In this case, Exo III can preferentially bind to the duplex region and hydrolyze the mononucleotides from the recessed 3′-termini in the direction of 3′- to 5′-ends. This can trigger the cleaved substrate fragment recycling and release more 6-carboxyfluorescein (6-FAM) into the solution, which can notably amplify the fluorescence signal in UO22+ detection system. Thereby, a novel fluorescence amplification strategy for UO22+ assay was developed. As a proof-of principle experiment, we demonstrated that the developed method can sensitively and selectively detect UO22+ in picomolar range. Our attempt will not only extend the application of DNAzyme and Exo III in biosensing areas but also pave the way to adaptation of the protocols to other related analytes.
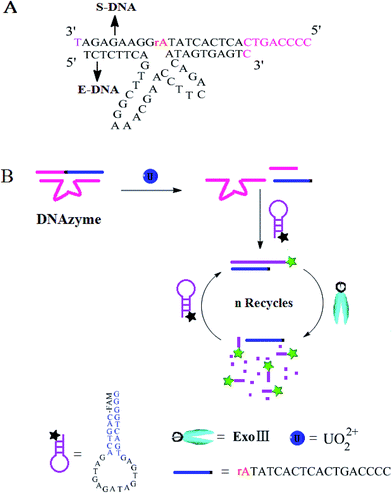 |
| Scheme 1 Molecular structure of RNA-cleaving DNAzyme (A) and schematic diagram for the analytical principle of Exo III-assisted signal amplification for UO22+ assay (B). | |
Experimental
Materials and chemicals
HPLC-purified oligonucleotides, Exo III and NEB buffer (pH 8.0, 500.0 mM Tris–HCl, 50.0 mM MgCl2, and 10.0 mM DTT) were purchased from TaKaRa Biotechnology Co., Ltd. (Dalian, China). The oligonucleotides sequences are shown as follows: enzyme strand (E-DNA): 5′-TCT CTT CAG TTC GGA AAC GAA CCT TCA GAC ATA GTG AGT C-3′. Substrate strand (S-DNA): 5′-CCC CAG TCA CTC ACT AT rA GGA AGA GAT-3′. SSP6: 5′-FAM-CAG TCA GAT GAG ATA GTG AGT GAC TGG GG-3′. Exo III (200 U μL−1) was diluted to 0.2 U μL−1 by 1‰ diethyl pyrocarbonate (DEPC) solution. MES (pH 5.5, NaNO3, 3.0 M) was purchased from Aladdin Chemistry Co., Ltd. (Shanghai, China). UO2(CH3CO2)2·2H2O was obtained from the 404 company limited, China National Nuclear Corporation (Lanzhou, China), and dissolved in water to make a 1.0 mM stock solution. All reagents were of analytical grade, and ultra-pure water (18.2 MΩ cm) was used throughout this work.
Apparatus
All fluorescence spectra were obtained with a Hitachi F-4500 fluorospectrophotometer (Tokyo, Japan). The absorption spectra were measured by a Shimadzu UV-2450 spectrophotometer (Kyoto, Japan). A pH meter (Sartorius AG, Germany) was used for pH adjustment.
Procedure for fluorescence measurement
Into a 2 mL EP tube, a 8 μL of 5.0 μM S-DNA was mixed with 10 μL of 5.0 μM E-DNA in 10 μL of MES buffer solution (pH 5.5) including 3.0 M NaNO3, and incubated at 95 °C for 5 min, then cooled down to room temperature for 1 h. Thereafter, the different concentrations of UO22+ were added into the DNAzyme solution prepared, diluting with water to a volume of 70 μL. The scission reaction was performed for 15 min at room temperature. Subsequently, a 10 μL of above-mentioned solution was mixed with 6 μL of 2.5 μM SSP6 in 50 μL NEB buffer solution of pH 8.0, followed by adding 20 μL of 0.2 U μL−1 Exo III into this solution. The mixtures were diluted to a final volume of 450 μL with water and incubated at 37 °C for 1 h. Then, the fluorescence spectra were obtained by scanning from 500 to 570 nm at λex = 495 nm. The spectral bandwidths of both the excitation and emission slits were set to 5 nm, respectively. The fluorescence intensity of the system was measured at λem = 517 nm, and represented as ΔF = F − F0, here F and F0 were the fluorescence intensities of the system with and without UO22+, respectively.
Result and discussion
Design strategy and sensing principle
In this work, we rationally designed a multipurpose probe of RNA-cleaving DNAzyme according to the literature,10,31 which is a hairpin structure containing the rA site and seven protruding mononucleotides at the 5′ terminus (Scheme 1A). In order to prevent the RNA-cleaving DNAzyme from digestion by Exo III, both 3′-ends of substrate strand (S-DNA) and enzyme strand (E-DNA) were protected by a phosphorothioate label. This multipurpose probe of RNA-cleaving DNAzyme was used as a target recognition element, catalytic DNAzyme and the primer of substrate fragment recycling amplification. Scheme 1B illustrates the principle of our novel biosensor for uranyl assay. We supposed that in the absence of UO22+, the DNAzyme is resistant to Exo III digestion. The introduction of UO22+ in the solution might cause the DNAzyme being activated, accompanied by the cleavage of the substrate strand in DNAzyme at the rA site.10,31 This can release two short oligonucleotide fragments (ssDNA) and a E-DNA strand. The fragment, which is complementary partially with a single-labeled hairpin signal probe (SSP), was expected to open the hairpin of SSP to form a dsDNA along with a specifically increase in fluorescence intensity. The resultant dsDNA with recessed 3′-termini can be selectively digested by Exo III,22–27 and the 6-carboxyfluorescein (6-FAM) was released into the solution. After the dsDNA was fully consumed, the substrate strand fragment was released owing to the inactivity of the Exo III toward ssDNA. Then this fragment can hybridize with a new SSP and initiate the next round of cleavage. Because one substrate strand fragment can be recycled hundreds of times to repeatedly initiate the removal of fluorescence reporter, this Exo III amplifying technique can remarkably improve sensitivity in assay system. Taking advantage of this strategy, the method can detect extremely low concentrations of UO22+ after Exo III amplification. Thereby, an ultrasensitive strategy for uranyl assay was established based on the Exo III-assisted substrate strand fragment recycling amplification.
Design and optimization of the signal probe
For this study, a SSP was purposely designed, which is constructed by an oligonucleotide strand with a 6-FAM at 5′-end and a G3 structure acting as a quencher at 3′-end (Scheme 1). The formation of a stem-loop structure results in a quenching in fluorescence due to photoinduced electron transfer from 6-FAM to G3. To achieve the best sensing performance, the sequence of the SSP was optimized. The sequences of both SSP5 and SSP6, which contain a stem of five- or six-base-pairs and a loop of 16 or 14 nucleotides respectively, were chosen as the signal probes (see Table S1 in ESI†), and the fluorescent intensities in the presence and absence of UO22+ (75 nM) were recorded. Fig. S1 (see ESI†) indicates that a maximum ΔF value was obtained by SSP6. This is attributed to the stability of SSP6, resulting in a lower blank value. So, SSP6 was chosen in this experiment. The concentration of SSP6 was also optimized. Fig. S2 (see ESI†) displays that with increasing amount of SSP6, the ΔF values went up. The addition of 6 μL of 2.5 μM SSP6 can achieve the best response. Afterwards, the ΔF declined upon addition of 2.5 μM SSP6 > 6 μL. Therefore, a 6 μL of 2.5 μM SSP6 was used to ensure a good signal-to-background ratio.
Mechanism of the Exo III-assisted substrate strand fragment recycling amplification
As stated above, we hypothesized that the conspicuous change in fluorescence signal might result from the Exo III-assisted substrate fragment recycling amplification. To confirm this presumption, the interactions between DNAzyme and SSP6 in the absence and presence of uranyl or Exo III were studied by spectrofluorimetry. Fig. 1A (curve 1) displays a weak signal in the absence of uranyl. The possible reason could be that the hairpin structure of the SSP6 brought G3 to close to 6-FAM, causing photoinduced electron transfer from 6-FAM to G3.32 Upon addition of uranyl, the fluorescence signal was enhanced obviously (Fig. 1A, curve 2). This implies the fluorophore of 6-FAM being apart from G3 quencher owing to the hybridization of cleaved substrate fragment with SSP6 to form a dsDNA. The presence of Exo III in DNAzyme–SSP6 solution caused a slight enhancement of fluorescence signal at 517 nm (Fig. 1A, curve 3), which can be attributed to the inactivity of Exo III towards dsDNA with 3′-protruding termini.33 Besides, the 3′-protruding termini designed in this work are resistant to cleavage by Exo III, and the degree of resistance might depend on the length of the extension, which could involve the background signal. Furthermore, the experimental result also showed that the activities of Exo III were not influenced by uranyl in the experimental condition (data not shown). The addition of Exo III into DNAzyme–SSP6–UO22+ solution led to a dramatic increased fluorescence signal (Fig. 1A, curve 4), which was proportional to the concentration of UO22+ (Fig. 1B, curves 1–6). This testifies that the Exo III can catalyze the stepwise removal of mononucleotides from the recessed 3′-hydroxyl termini of dsDNA,15–21 resulting in the more 6-FAM being released into the solution. Thereby, a 500% signal enhancement (ΔFExo III (F4 − F3)/ΔFwithout Exo III (F2 − F1)) was achieved compared to the system without Exo III in the presence of 7.5 nM UO22+ (Fig. 1A), and a limit of detection as low as 2.4 pM was obtained. This result is significantly lower than those of the traditional and DNAzyme-based methods for UO22+ detection.4,5,7,10,39–43
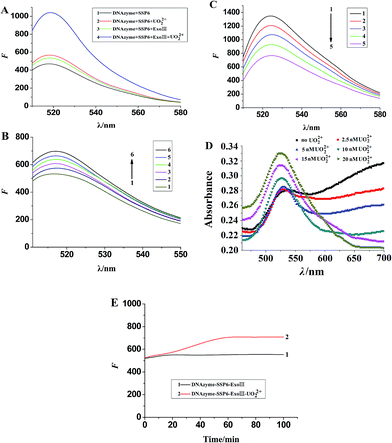 |
| Fig. 1 Fluorescence spectra of the DNAzyme–SSP6–Exo III–UO22+ system (A and B), and DNAzyme–SGI–UO22+ system (C), absorption spectra of the DNAzyme–AuNPs–UO22+ system (D) and fluorescence intensity–time curve of the system (E). (A) cUO22+ = 7.5 nM, cS-DNA = 12.5 nM, cE-DNA = 12.5 nM, cSSP6 = 30 nM, cExo III = 10 U mL−1; (B) cUO22+ (pM)/(1–6): 0, 16.0, 32.0, 48.0, 63.0, 95.0; (B and E) cS-DNA = 12.7 nM, cE-DNA = 15.9 nM, cSSP6 = 33.3 nM, cExo III = 8.9 U mL−1; (C) cUO22+ (nM)/(1–5): 0, 4.4, 11.1, 17.8, 26.7, cS-DNA = 78.0 nM, cE-DNA = 56.0 nM, cSGI = 0.11 μM; (D) cE-DNA = 0.177 μM, cS-DNA = 0.177 μM, cNaCl = 80.0 mM; (E) cUO22+ = 0.63 nM. | |
A series of the control experiments were also carried out for validating above conclusion. Fig. 1C (curve 1) illustrates a noticeable fluorescence signal in the DNAzyme–SYBR Green I (SGI) solution. This may be that SGI exhibits much higher binding affinity and stronger fluorescent emission toward the un-cleaved hairpin probe than the cleaved sequence.34 The addition of UO22+ into this solution resulted in a decreased fluorescence signal, which was proportional to the concentrations of UO22+ (Fig. 1C, curves 2–5). This result indicates that DNAzyme might be cleaved into the substrate strand fragments and a E-DNA strand by UO22+. The data from UV-vis spectra further confirmed this phenomenon. Fig. 1D displays that the absorbance at 526 nm enhanced gradually with increasing UO22+ concentration, accompanied by a decrease of absorbance at 650 nm. This result implies that the presence of UO22+ leads to the cleavage of DNAzyme to release the substrate strand fragments (ssDNA). The resultant ssDNA can be adsorbed onto AuNPs, protecting them from aggregation. Fig. 1E shows the real-time fluorescence intensity change at 517 nm in the DNAzyme–SSP6–Exo III–UO22+ system. As illustrated in curve 1 in Fig. 1E, there was a weak fluorescence signal in the absence of UO22+. Upon addition of UO22+ into this solution, the Exo III enzymatic reaction started right away, and the fluorescence intensity of the system increased slowly in the range of 0 to 20 min. While a rapid increase of signal intensity was observed ranging from 20 to 60 min (curve 2). This result indicated a gradual accumulation of 6-FAM in the solution, leading to a continuous increasing emission intensity–time curve. The signal reached a maximum value about 60 min, and then kept relatively stable. Based on the above-mentioned phenomenons and the clues from the literatures,22–27 we conclude that the interaction of DNAzyme with UO22+ leads to release a short oligonucleotide fragment that is complementary partially with a SSP. This fragment opens the hairpin of SSP to form a dsDNA with recessed 3′-termini. The resultant dsDNA is selectively digested by Exo III, releasing substrate fragments. The recycling of each substrate fragment can be repeated multiple times, resulting in the accumulation of 6-FAM in the solution along with a remarkably amplified detection signal.
Optimization of sensing conditions
To achieve the best sensing performance, the experimental conditions including the concentration of Exo III, the molar ratio of S-DNA to E-DNA, and pH were optimized. First, the effect of the concentration of Exo III on the signal response was investigated. Fig. S3 (see ESI†) reveals that the fluorescence response toward 0.63 nM UO22+ increased with the rise of Exo III concentration, reaching a maximum on addition of 20 μL of 0.2 U μL−1 Exo III. Further increase of the Exo III concentration caused a decrease of fluorescence intensity (ΔF). Thus, a 20 μL of 0.2 U μL−1 Exo III was chosen as the optimum assay condition.
The influence of molar ratio between S-DNA and E-DNA on the sensing system was studied. Fig. S4 (see ESI†) illustrates that a maximum ΔF value was attained at molar ratio of 8
:
10 for S-DNA
:
E-DNA. From our design, we may find the reason that the free S-DNA can open SSP6 to lead to a higher background fluorescence. While a higher concentration of E-DNA can inhibit the dissociation of DNAzyme, accordingly decreasing background signal. Whereas a lower molar ratio, such as 6
:
10, was also unfavorable. This might attribute to the formation of a small number of DNAzymes, which may not satisfy the requirement of the scission reaction. Thereby, a molar ratio of 8
:
10 for S-DNA
:
E-DNA was selected in this assay.
According to previous reports,4,10 the scission processes took place at pH about 5.5. Fig. S5 (ESI†) depicts that the ΔF increased with the rise of pH and reached a maximum in MES buffer of pH 5.5, which was consistent with those reported by Lu and co-worker.4,10 While the activity of DNAzyme dramatically declined at pH > 5.5. These observations reveal that the scission reaction of DNAzyme by UO22+ as a cofactor is highly pH dependent. The reason could be that pH influences the uranyl ion speciation in solution. The uranyl species produced at pH 5.5 is the most effective one for carrying out the reaction, and the hydrolyzed species such as UO2(OH)+ start to dominate at pH > 5.5. So, MES buffer of pH 5.5 was selected for controlling the acidity of the scission reaction. Through consulting literatures,35 a NEB buffer (pH 8.0, 500.0 mM Tris–HCl, 50.0 mM MgCl2, 10.0 mM DTT) was chosen for Exo III enzymatic reaction. As shown in Fig. S6 (see ESI†), a maximum was accomplished on addition of 50 μL NEB buffer of pH 8.0. So, the NEB buffer of pH 8.0 was employed for Exo III enzymatic reaction.
Sensitivity and selectivity of the sensing system
To evaluate the feasibility of UO22+ detection by DNAzyme–Exo III–SSP6 system, various concentrations of UO22+ were examined under optimized conditions. Fig. 2 shows a good linear correlation (r = 0.990) between the ΔF value and UO22+ concentrations ranging from 8.1 to 95 pM, and the equation of linear regression was ΔF = 1.62c (pM) + 22.0. The detection limit of 2.4 pM was estimated according to the equation LOD = 3Sb/slope (Sb represents the standard deviation of the 11 blank measurements), which was much lower than those of previously reported biosensors.4,5,7,10,36–43 The relative standard deviations for the 11 determinations of 16.0 pM, 48.0 pM, and 63.0 pM UO22+ were 4.07%, 2.09% and 4.25%, respectively. Therefore, these results indicated the effectiveness of the assay.
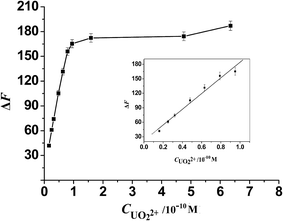 |
| Fig. 2 Calibration curve for the biosensing system. The error bars represent the standard deviations of three repetitive measurements. | |
The selectivity of this strategy was also investigated by testing the change of fluorescence intensity in the presence of potentially interfering ions. Fig. 3 demonstrates that 100 times of Ca2+, Sr2+, Zn2+, Cd2+, Ba2+, Co2+, Cr3+, Tb3+, PO43−, HPO42−, Cl−, CO22−, and SO42−, 50 times of H2PO4−, Fe2+, Fe3+, Al3+, Pb2+, Cu2+, Mn2+; 20 times of Hg2+, and 15 times of Ag+ do not interfere with determination. It is reported that some metals such as Cu2+, Fe2+, Fe3+ and Hg2+ induced strong quenching to FAM.5 However, our results confirmed that the UO22+ sensor has high selectivity against metal ions. This might be due to Exo III enzymatic reaction being performed in a NEB buffer of pH 8.0, which was not consistent with that reported by the literature.5 Hence, the method exhibits very good selectivity.
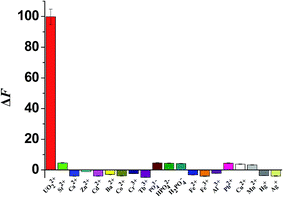 |
| Fig. 3 Selectivity of the DNAzyme–SSP6–Exo III biosensing system. cS-DNA = 12.7 nM, cE-DNA = 15.9 nM, cSSP6 = 33.3 nM, cExo III = 8.9 U mL−1, cUO22+ = 47.6 pM, cCa2+, Sr2+, Zn2+, Cd2+, Ba2+, Co2+, Cr3+, Tb3+, PO43−, HPO42− = 4.76 nM, cHg2+ = 0.952 nM, cAg+ = 0.714 nM, cFe2+, Fe3+, Al3+, Pb2+, Cu2+, Mn2+, H2PO4− = 2.38 nM. | |
Analysis of uranyl in real samples
To investigate whether this method was applicable to natural samples, seven samples were collected from the locations near an uranium mine, Xiangjiang River and a pond water in an university, respectively. All samples were filtrated, and the samples 1 and 3 were diluted for 20 times, samples 2 and 4 for 10 times and sample 7 for 5.0 × 105 times using ultra-pure water, respectively. Then these samples were tested by the developed method. Recovery test was carried out by adding a known amount of UO22+ ions to samples, and the results were shown in Table 1. It is obvious that the developed method for UO22+ detection was reliable, ultrasensitive and practical.
Table 1 Determination results of UO22+ in the samples (n = 9)a
Samples |
Found (pM) |
RSD (%) |
Added (pM) |
Total found (pM) |
Reccovery (R%) |
Sample 1: the north pond of the factory; sample 2: the west pond of the factory 1; sample 3: the west pond of the factory 2; sample 4: the outfall of the factory near Xiangjiang River; sample 5: Xiangjiang River near the Jiefang Road; sample 6: the pond water in University of South China; sample 7: the surface water of an uranium mine factory. The results obtained by diluting samples 1 and 3 for 20 times. The results obtained by diluting samples 2 and 4 for 10 times. The results obtained by diluting sample 7 for 5.0 × 105 times using ultra-pure water. |
1 |
37.5b |
1.25 |
31.7 |
70.1 |
102.8 |
2 |
53.4c |
1.04 |
31.7 |
89.0 |
104.5 |
3 |
41.3b |
2.07 |
31.7 |
74.2 |
103.7 |
4 |
47.4c |
1.21 |
31.7 |
77.2 |
94.0 |
5 |
10.7 |
1.79 |
31.7 |
43.6 |
103.7 |
6 |
18.8 |
4.53 |
63.0 |
83.4 |
102.5 |
7 |
55.2d |
2.63 |
16.0 |
72.0 |
105.0 |
Comparison of this strategy with other methods
The work described here develops an amplification strategy for ultrasensitive detection of UO22+ using a multipurpose RNA-cleaving DNAzyme and a SSP6 as signal probe. Compared to previously reported methods, the proposed strategy has several unique features and advantages. Firstly, Exo III-assisted signal amplification can significantly improve the sensitivity of the method. In addition, each UO22+ is also able to react with multiple catalytic DNAzyme to amplify the response signal through catalytic turnovers. These two factors result in an ultrahigh sensitivity for UO22+ assays. The detection limit of 2.4 pM obtained by this work is 1 to 3 orders of magnitude lower than those of the DNAzyme-based methods,4,5,7,10,39,40 and 5 orders of magnitude lower than traditional methods for UO22+ detection (Table S2, see ESI†).41–43 Secondly, the use of a single-labeled hairpin signal probe is obviously more convenient and economical than the double-labeled ones. More importantly, such a method does not require a specific recognition site due to the intrinsic property of Exo III, and can undifferentiated degrade dsDNA from the 3′-hydroxyl termini, thus it has much better flexibility in choosing molecular beacon sequences and offers wider applicability. Moreover, DNAzyme can be extended as universal probes, and their combination with Exo III could provide a general method for ultrasensitive and selective detection of a wide range of targets.
Conclusions
In summary, we proposed a novel strategy for ultrasensitive detection of uranyl based on the Exo III-assisted substrate fragment recycling signal amplification. Both a multipurpose DNAzyme and SSP6 as signal probe were designed, and Exo III was used for the signal amplification in assay system. The proposed strategy can significantly improve the sensitivity of the biosensor, giving a detection limit down to 2.4 pM, which is much lower than those of previously reported biosensors. It also showed significantly high selectivity toward target. The assay has been successfully applied for detecting UO22+ in real samples. In addition, the mechanism of the signal amplification by Exo III was demonstrated by a series of experiments. This strategy possesses the prospect of becoming a sensitive and practical platform for the analysis of UO22+ ions in environmental and biomedical fields.
Acknowledgements
The authors gratefully acknowledge the support of the National Natural Science Foundation of China (No. 21177052, 81502850), the Science and Technology Program of Hunan Province in China (No. 2010SK3039) and the Natural Science Foundation of Hunan Province in China (No. 2015JJ2122).
References
- A. A. Banday, S. Priyamvada, N. Farooq, A. N. K. Yusufi and F. Khan, Food Chem. Toxicol., 2008, 46, 2080–2088 CrossRef CAS PubMed.
- F. Shaki, M.-J. Hosseini, M. Ghazi-Khansari and J. Pourahmad, Biochim. Biophys. Acta, 2012, 1820, 1940–1950 CrossRef CAS PubMed.
- J. Nriagu, D. H. Nam, T. A. Ayanwola, H. Dinh, E. Erdenechimeg, C. Ochir and T. A. Bolormaa, Sci. Total Environ., 2012, 414, 722–726 CrossRef CAS PubMed.
- J. H. Lee, Z. Wang, J. Liu and Y. Lu, J. Am. Chem. Soc., 2008, 130, 14217–14226 CrossRef CAS PubMed.
- J. Liu, A. K. Brown, X. Meng, D. M. Cropek, J. D. Istok, D. B. Watson and Y. Lu, Proc. Natl. Acad. Sci. U. S. A., 2007, 104, 2056–2061 CrossRef CAS PubMed.
- P. Wu, K. H. Wang, T. Lan and Y. Lu, J. Am. Chem. Soc., 2013, 135, 5254–5257 CrossRef CAS PubMed.
- B. Zhou, L. F. Shi, Y. S. Wang, H. X. Yang, J. H. Xue, L. Liu, J. C. Yin and J. C. Wang, Spectrochim. Acta, Part A., 2013, 110, 419–424 CrossRef CAS PubMed.
- H. Zhang, Y. Ruan, L. Lin, M. Lin, X. Zeng, Z. Xi and F. Fu, Spectrochim. Acta, Part A, 2015, 146, 1–6 CrossRef CAS PubMed.
- W. Xu, H. Xing and Y. Lu, Analyst, 2013, 138, 6266–6269 RSC.
- B. Zhou, Y. S. Wang, H. X. Yang, J. H. Xue, J. C. Wang, S. D. Liu, H. Liu and H. Zhao, Microchim. Acta, 2014, 181, 1353–1360 CrossRef CAS.
- A. K. Brown, J. Liu, Y. He and Y. Lu, ChemBioChem, 2009, 10, 486–492 CrossRef CAS PubMed.
- A. X. Zheng, J. R. Wang, J. Li, X. R. Song, G. N. Chen and H. H. Yang, Chem. Commun., 2012, 48, 374–376 RSC.
- Y. Zhao, F. Chen, Y. Wu, Y. Dong and C. Fan, Biosens. Bioelectron., 2013, 42, 56–61 CrossRef CAS PubMed.
- Y. Huang, X. Liu, L. Zhang, K. Hu, S. Zhao, B. Fang, Z. F. Chen and H. Liang, Biosens. Bioelectron., 2015, 63, 178–184 CrossRef CAS PubMed.
- X. Liu, M. Chen, T. Hou, X. Wang, S. Liu and F. Li, Biosens. Bioelectron., 2014, 54, 598–602 CrossRef CAS PubMed.
- T. Bao, H. Shu, W. Wen, X. Zhang and S. Wang, Anal. Chim. Acta, 2015, 862, 64–69 CrossRef CAS PubMed.
- X. Gan, H. Zhao, S. Chen and X. Quan, Analyst, 2015, 140, 2029–2036 RSC.
- C. Tao, Y. Yan, H. Xiang, D. Zhu, W. Cheng, H. Ju and S. Ding, Chem. Commun., 2015, 51, 4220–4222 RSC.
- W. Ren, Y. Zhang, W. T. Huang, N. B. Li and H. Q. Luo, Biosens. Bioelectron., 2015, 68, 266–271 CrossRef CAS PubMed.
- X. Wang, T. Hou, T. Lu and F. Li, Anal. Chem., 2014, 86, 9626–9631 CrossRef CAS PubMed.
- J. Chen, S. Zhou and J. Wen, Anal. Chem., 2014, 86, 3108–3114 CrossRef CAS PubMed.
- C. Chen and B. Li, Biosens. Bioelectron., 2014, 54, 48–54 CrossRef CAS PubMed.
- W. Li, X. Liu, T. Hou, H. Li and F. Li, Biosens. Bioelectron., 2015, 70, 304–309 CrossRef CAS PubMed.
- J. Zhao, M. Xin, Y. Cao, Y. Yin, Y. Shu and W. Ma, Anal. Chim. Acta, 2015, 860, 23–28 CrossRef CAS PubMed.
- X. Xue-tao, L. Kai-yi and Z. Jia-ying, Analyst, 2014, 139, 4982–4986 RSC.
- Y. Lv, Q. Xue, X. Gu, S. Zhang and J. Liu, Analyst, 2014, 139, 2583–2588 RSC.
- Y. Gao and B. Li, Anal. Chem., 2013, 85, 11494–11500 CrossRef CAS PubMed.
- C. Luo, H. Tang, W. Cheng, L. Yan, D. Zhang, H. Ju and S. Ding, Biosens. Bioelectron., 2013, 48, 132–137 CrossRef CAS PubMed.
- J. J. Zhang, F. F. Cheng, J. J. Li, J. J. Zhu and Y. Lu, Nano Today, 2016, 11, 309–329 CrossRef CAS PubMed.
- Q. Xue, Y. Lv, H. Cui, X. Gu, S. Zhang and J. Liu, Anal. Chim. Acta, 2015, 856, 103–109 CrossRef CAS PubMed.
- J. Q. Zhang, Y. S. Wang, J. H. Xue, Y. He, H. X. Yang, J. Liang, L. F. Shi and X. L. Xiao, J. Pharm. Biomed. Anal., 2012, 70, 362–368 CrossRef CAS PubMed.
- W. Wang, Y. Jin, Y. Zhao, X. Yue and C. Zhang, Biosens. Bioelectron., 2013, 41, 137–142 CrossRef CAS PubMed.
- Y. Gao and B. Li, Anal. Chem., 2014, 86, 8881–8887 CrossRef CAS PubMed.
- Y. Xu, J. Xu, Y. Xiang, R. Yuan and Y. Chai, Biosens. Bioelectron., 2014, 51, 293–296 CrossRef CAS PubMed.
- L. Guo, D. Nie, C. Qiu, Q. Zheng, H. Wu, P. Ye, Y. Hao, F. Fu and G. Chen, Biosens. Bioelectron., 2012, 35, 123–127 CrossRef CAS PubMed.
- R. Banerjee, Y. Katsenovich, L. Lagos, M. Senn, M. Naja, V. Balsamo, K. H. Pannell and C. Z. Li, Electrochim. Acta, 2010, 55, 7897–7902 CrossRef CAS.
- S. Betelu, C. Vautrin-Ul, J. Ly and A. Chaussé, Talanta, 2009, 80, 372–376 CrossRef CAS PubMed.
- X. Chen, L. He, Y. Wang, B. Liu and Y. Tang, Anal. Chim. Acta, 2014, 847, 55–60 CrossRef CAS PubMed.
- M. H. Li, Y. S. Wang, J. X. Cao, S. H. Chen, X. Tang, X. F. Wang, Y. F. Zhu and Y. Q. Huang, Biosens. Bioelectron., 2015, 72, 294–299 CrossRef CAS PubMed.
- Q. Tang, Y. Yuan, X. Xiao, J. Hu, D. Ma and Y. Gao, Microchim. Acta, 2013, 180, 1059–1064 CrossRef CAS.
- S. Lehmann, G. Geipel, G. Grambole and G. Bernhard, Spectrochim. Acta, Part A, 2009, 73, 902–908 CrossRef CAS PubMed.
- V. K. Jain, R. A. Pandya, S. G. Pillai and P. S. Shrivastav, Talanta, 2006, 70, 257–266 CrossRef CAS PubMed.
- S. Ozdemir and E. Kilinc, Microchim. Acta, 2012, 178, 389–397 CrossRef CAS.
Footnote |
† Electronic supplementary information (ESI) available. See DOI: 10.1039/c6ra20625e |
|
This journal is © The Royal Society of Chemistry 2016 |
Click here to see how this site uses Cookies. View our privacy policy here.