DOI:
10.1039/C6RA20435J
(Paper)
RSC Adv., 2016,
6, 100954-100961
Enhanced electrochemical capacitive performance of “sandwich-like” MWCNTs/PANI/PSS-GR electrode materials†
Received
13th August 2016
, Accepted 16th October 2016
First published on 17th October 2016
Abstract
A novel “sandwich-like” MWCNTs/PANI/PSS-GR nanocomposites are achieved via in situ chemical oxidative polymerization and electrostatic adsorption between the negatively-charged poly(sodium 4-styrenesulfonate) (PSS) modified graphene (GR) nanosheets and the positively-charged polyaniline (PANI) on the surface of multiwalled carbon nanotubes (MWCNTs). The morphology and structure of the composites are characterized by scanning electron microscopy (SEM), transmission electron microscopy (TEM), X-ray diffraction (XRD), ultraviolet-visible (UV-vis) and Fourier transform infrared (FT-IR), respectively. It is clearly seen that the PANI is uniformly coated on the surface of MWCNTs to form the MWCNTs/PANI bundles with diameter around 60 nm, and then the obtained MWCNTs/PANI are successfully coated on the surface of the PSS-GR nanosheets. In addition, the results of UV-vis and FT-IR confirm the successful synthesis of MWCNTs/PANI/PSS-GR nanocomposites and the existence of interaction between PSS-GR and MWCNTs/PANI. The results of electrochemical tests demonstrate that the “sandwich-like” nanocomposites present superior electrochemical properties in comparison with pure PANI and MWCNTs/PANI, which can ascribe to the structure advantage and the synergistic effect of MWCNTs, PANI and GR nanosheets. The specific capacitance (SC) of MWCNTs/PANI/PSS-GR nanocomposites is 602.5 F g−1 at the current density of 0.5 A g−1 in a three-electrode system, and still keeps 332.5 F g−1 (0.5 A g−1) in a symmetric two-electrode system. Furthermore, 78.32% of the initial specific capacitance is retained after 2000 charging/discharging cycles, which is much better than that of pure PANI.
Introduction
With the rapidly increasing energy demand and forthcoming energy crisis, supercapacitors, high-performance and efficient energy storage devices, have attracted intensive attention in recent decades due to their excellent performance, such as high power density, longer cycle life and smaller environmental impact.1–3 It has been demonstrated that the properties of supercapacitors are mainly determined by the electrode materials.4 Therefore, there have been growing interests in developing high-performance electrode materials for supercapacitors. Kinds of electrode materials, such as conducting polymers,5 carbon nanomaterials6 and metal oxides,7 have been studied for supercapacitors. Especially, the conducting polymers (PANI, polypyrrole, polythiophene) have been widely investigated as electrode materials for advanced device applications. By contrast, PANI has been seen as one of the best and the most promising candidates for supercapacitor electrode materials due to its low cost, easily available synthesis, good electronic properties, unique doping/dedoping property, and excellent electrochemical performance.8,9 However, PANI suffers from large volumetric swelling and shrinking during charge/discharge process as a result of ion doping and dedoping.10 This volumetric alternation often leads to structural breakdown, which exhibits poor structural stability and cycling stability, and limits its application for supercapacitor electrode materials.11–13 Therefore, reinforcing the structural stability and cycling stability of polyaniline is a major challenge for practical application. In order to overcome these problems, composites of PANI with other nanomaterials such as metal oxide nanoparticles, metal nanoparticles, carbon nanomaterials, have been prepared to improve its mechanical, electrical and electrochemical properties. Among them, some extensive promising researches have reported that the combination of PANI with carbon nanomaterials can enhance the electrochemical performance and the cycling stability.14
Carbon nanotubes (CNTs) possess novel properties such as good electrical conductivity,15 ideal specific surface area, low specific weight, high charge transport capability, and high chemical stability, which have been widely used as the supercapacitor electrode materials.16,17 The combination of CNTs with conducting polymers has stimulated much research interest in the application of electrode materials for supercapacitors. Besides, two-dimensional graphene (GR) nanosheets also have been anticipated as an ideal candidate for supercapacitor electrode materials similarly, because of their large surface area, high electrical conductivity and superior mechanical property.18,19 In particular, the composites of GR and conducting polymers have received tremendous attention as electrode materials for supercapacitors due to synergistic effect and enhanced electrochemical properties.20–22 However, due to the planar sp2-carbon, GR nanosheets have high tendency to agglomerate and restack together during their synthesis and processing procedure caused by van der Waals interaction.8 Therefore, the enhancement of homogeneous dispersion of GR nanosheets is a challenge to the application of GR nanocomposites.23 In order to overcome these problems, covalent and non-covalent modification of GR have been widely discussed. Covalent attachment to GR through covalent bonds may introduce defects onto the parallel planes and degrade its electrical conductivity.24,25 In contrast, non-covalent strategies, particularly using polyelectrolytes as functional agents, are more favorable. Poly(sodium 4-styrenesulfonate) (PSS), a kind of water soluble and non-toxic polyelectrolyte, has been used as the spacer to prevent GR nanosheets from agglomeration, so it can preserve the intrinsic electronic and structural properties of GR. The GR nanosheets can be dispersed by PSS via the interaction of the independent mechanism: the negatively charged PSS can interact with GR through π–π interaction and assist the dispersion of GR in water by electrostatic repulsion to prevent the agglomeration and restacking. Until now, massive composites of GR/PANI26–30 and MWCNTs/PANI31–33 have been widely explored as electrode materials. For instance, Ramana et al.34 reported CNTs/PANI nanocomposites with core–shell structures via an easy chemical oxidative polymerization. They found that the core–shell structures were electrochemically active and the composites possessed a high specific capacitance of 368.4 F g−1, which was higher than that of PANI (225.5 F g−1). This was attributed to the reduction in diffusion and migration lengths of the electrolyte ions during the fast charge/discharge process. Zhao et al.13 reported a carbon nanotube sponge grafted by two polymers (PANI and PPy) with a controlled layer sequence, which could serve as a porous supercapacitor electrode. The systematic electrochemical test showed that the nanocomposites with two polymers possessed an excellent cycling stability after 3500 cycles and much higher capacitance retention (88% retention of CNT/PANI/PPy) than that of the single polymer grafting (35.6% retention of CNT/PANI). Liu et al.35 prepared a novel tremella-like graphene/polyaniline composite via self-assembly of graphene nanosheets during polymerization of aniline in H2SO4/N,N-dimethylformamide solution. The composite presented a higher specific capacitance (497 F g−1) than that of the pure PANI (36 F g−1) and graphene (123 F g−1) at 0.5 A g−1. Moreover, an outstanding rate capability of after 1000 cycles was obtained.
In this study, we take advantage of polyaniline (PANI), multiwalled carbon nanotubes (MWCNTs) and graphene (GR) nanosheets to construct high-performance “sandwich-like” nanostructured composites as an electrode material for supercapacitors. The PSS is used to fabricate the interface bounded by electrostatic adsorption between the negatively-charged PSS modified GR nanosheets and the positively-charged MWCNTs/PANI, which opens a broad perspective to obtain the MWCNTs/PANI/PSS-GR nanocomposites. The preparation procedures are illustrated in Fig. 1. There are four potential benefits of constructing the “sandwich-like” nanostructured composites with MWCNTs/PANI coated on the surface of PSS-GR nanosheets. Firstly, MWCNTs cores are used as an appropriate supporting materials for PANI and the interaction between them would induce the formation of an ordered PANI chain structure on the surface of MWCNTs, which contributes to the improvement of mechanical and electrochemical properties. Secondly, the “sandwich-like ”structure can prevent the aggregation of GR nanosheets which is beneficial to improve the specific capacitance. Thirdly, the existence of GR nanosheets can suppress the volumetric swelling and shrinking during charge/discharge cycling of PANI and improve the cycling stability. Fourthly, the GR nanosheets as outer layers and the wrapped MWCNTs cores both own excellent electrical conductivity, which is useful to construct a conductive network and improves the electrochemical performance of the nanocomposites synergistically.
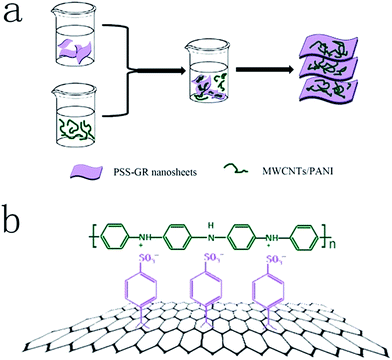 |
| Fig. 1 Schematic illustration for the synthesis of MWCNTs/PANI/PSS-GR nanocomposites (a); illustration of electrostatic adsorption between negatively-charged PSS-GR nanosheets and positively-charged PANI on the surface of MWCNTs (b). | |
Experimental
Materials and Reagents
Graphene nanosheets were supplied by Nanjing XFNANO Materials Tech. MWCNTs (Chengdu Organic Chemicals Co. Ltd., Chinese Academy of Sciences) were purified by HNO3, and then thoroughly washed with deionized water and ethanol before to be dried at 80 °C for 12 h. Aniline and ammonium peroxydisulfate ((NH4)2S2O8, APS) were obtained from Wuhan Shenshi chemical instrument network Co. Ltd (China). Poly(sodium 4-styrenesulfonate) (Mw = 80
000, 20% aqueous solution, PSS) was purchased from Sigma. All other chemicals and solvents were used as received without further treatment.
Preparation of MWCNTs/PANI nanocomposites
Firstly, in order to remove the amorphous carbons and increase the functional groups of the surface, the purified MWCNTs were treated in nitric acid for 6 h at 80 °C. The MWCNTs/PANI nanocomposites were synthesized via an in situ oxidative polymerization method using procedures documented in the literature.36 In this method, the purified MWCNTs (0.07 g) were dispersed in 50 mL HCl (1 M) and sonicated for 0.5 h. Meanwhile, 0.3 g of aniline monomer was dissolved in 150 mL HCl (1 M) and stirred for 1 h at room temperature. The MWCNTs solution was added into aniline solution and sonicated for another 0.5 h. Then APS (0.5 g) was added to the mixture and stirred for 5 h at 9 °C. It could be found that the colour of the mixture changed from black to greenish black. The mixture was filtered and washed with deionized water 3 times, then dried at 60 °C in vacuum for 12 h to get MWCNTs/PANI nanocomposites (the 72.8 wt% of PANI in the MWCNTs/PANI composites was evaluated by calculating the weight difference of MWCNTs).
Preparation of stable aqueous dispersions of PSS-GR nanosheets
Briefly, 20 mg of GR nanosheets were fully dispersed in distilled water with ultrasonication for several hours to obtain a black suspension (1 mg mL−1). After that, the pre-prepared GR aqueous solution were added into 100 mL PSS solution (2 mg mL−1, 20% PSS aqueous solution) and treated via ultrasonic processing for 1 h. Finally, the mixture was centrifuged to eliminate excess PSS and made a stable black water dispersion of PSS-GR.
Preparation of MWCNTs/PANI/PSS-GR nanocomposites
The MWCNTs/PANI/PSS-GR nanocomposites were prepared by mechanically blending the prepared PSS-GR aqueous solution with MWCNTs/PANI aqueous solution. The obtained PSS-GR dispersion was dropped into 50 mL of the fresh prepared MWCNTs/PANI solution (1 mg mL−1) and then the mixture was treated via ultrasonic processing for 0.5 h, subsequently stirred for 6 h at the room temperature. Finally, the MWCNTs/PANI/PSS-GR nanocomposites were fabricated after vacuum filtration and dried at 60 °C (the product was abbreviated as MPG20). The final mass of the nanocomposites was 71.9 mg. The mass ratio of PANI, MWCNTs and PSS-GR nanosheets were 50.62%, 18.92% and 30.46%, respectively, and the PSS coated on the surface of GR nanosheets was 71.09% (Fig. S1†). For comparison, MWCNTs/PANI/PSS-GR nanocomposites with various amount of GR nanosheets (10 mg, 30 mg) were synthesized under the same conditions (the products were abbreviated as MPG10 and MPG30, respectively).
Preparation of the modified electrodes
The glass carbon electrode (GCE) was polished with 0.05 μm alumina slurry and sonicated with ethanol and deionized water, successively. The MWCNTs/PANI/PSS-GR nanocomposites were added into the Nafion (1 wt%) and ultrasonically treated for 1 h. The homogeneous mixture (10 μL) was spread on a glass carbon electrode surface and dried at room temperature to obtain the working electrode.
All the electrochemical measurements (cyclic voltammery (CV) and galvanostatic charge–discharge (GCD)) were done using Autolab (μ3AUT71018) electrochemical work station. In a three-electrode system, the glass carbon electrode coated with as-prepared sample was used as the working electrode, platinum wire and Ag/AgCl electrodes were used as the counter and reference electrodes in 1 M H2SO4 electrolyte. The electrochemical properties of two-electrode system were performed using assembled coin cell (CR2032) in symmetric configuration. The prepared MPG20 nanocomposites, acetylene black and poly(vinylidene fluoride) were mixed at a weight ratio of 8
:
1
:
1 and made into a film by rolling. Then, the obtained film was cut into round slices using as two electrodes with the mass loading of about 2 mg for a single electrode, whereas the glass fiber was used as the separator and stainless steel sheets were used as the current collectors. The photographs of the cell are shown in Fig. S2.† CV tests were performed with the potential range from −0.2 to 1 V and the scan rates from 10 to 100 mV s−1. The GCD curves were measured in the potential range from 0 to 0.8 V at different current densities of 0.5, 1, 2, 4 and 10 A g−1. The cycling stability of the electrode materials was conducted by consecutive GCD tests at a constant current density of 1 A g−1.
Results and discussion
Structure and chemical composition analysis
The microstructures of the obtained MWCNTs/PANI and “sandwich-like” MPG20 nanocomposites are characterized by SEM and TEM, respectively, as shown in Fig. 2. From Fig. 2A and C, MWCNTs/PANI nanocomposites display the random three-dimensional network structure and fiber-like morphology with an average diameter of 60 nm, which is useful for the transmission of the electrolyte ion between the electrolyte and the electrode, and beneficial for high specific capacitance supercapacitor electrode materials because of the larger specific surface area (SSA).37 It is obviously seen that the aniline monomer is uniformly polymerized along radial direction of MWCNTs axis with core–shell structure. The reason can be due to the formation of active sites by the polymerization between the functional groups of MWCNTs and PANI. Compared with the MWCNTs/PANI, the “sandwich-like” MPG20 nanocomposites are evidently composed of the MWCNTs/PANI and PSS-GR (Fig. 2B). The “sandwich-like” structure is also revealed by the TEM image. As shown in Fig. 2D, the MWCNTs/PANI are coated on the surface of GR nanosheets and formed a hierarchical structure. The TEM images of MPG10 and MPG30 are shown in Fig. S3.† Compared with the MPG20, it can be clearly seen that the MWCNTs/PANI are coated on the surface of less GR nanosheets in MPG10. On the contrary, the excessive GR nanosheets are adsorbed around MWCNTs/PANI with much stacking in MPG30.
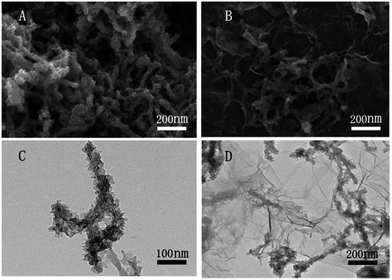 |
| Fig. 2 SEM images for MWCNTs/PANI (A) and MPG20 nanocomposites (B), respectively; TEM images for MWCNTs/PANI (C) and MPG20 nanocomposites (D), respectively. | |
The XRD patterns of pure PANI, MWCNTs/PANI and MWCNTs/PANI/PSS-GR nanocomposites are shown in Fig. 3A, respectively. PANI shows three broad peaks at 2θ = 14.90°, 20.19° and 25.23°, corresponding to (011), (020) and (200) planes of PANI in its emeraldine salt form, respectively.38 The exhibited crystalline peaks of MWCNTs/PANI and MWCNTs/PANI/PSS-GR are similar to that of pure PANI, revealing that no additional crystalline order has been introduced into the nanocomposites.
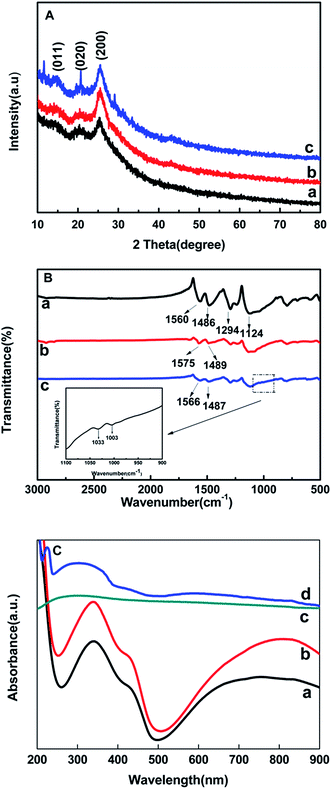 |
| Fig. 3 XRD pattern (A) and FTIR spectra (B) of pure PANI (a), MWCNTs/PANI (b), MWCNTs/PANI/PSS-GR (c); UV-visible spectra (C) of pure PANI (a), MWCNTs/PANI (b), GR (c), and MWCNTs/PANI/PSS-GR nanocompsoites (d). | |
The chemical composition of the as-prepared samples is characterized by FT-IR, as shown in Fig. 3B. The inset is the amplification of the curve c. For the pure PANI, the adsorption peaks located at 1560 and 1486 cm−1 can be ascribed to the C
C stretching vibration mode of the quinoid ring and benzenoid ring in the emeraldine salt (ES). The peaks at 1294 and 1124 cm−1 correspond to the C–N stretching vibration of secondary aromatic amine ring and C–H stretching vibration, respectively. In curve b, the peaks at 1560 and 1486 cm−1 shift to 1575 and 1489 cm−1, this may be due to the interaction between MWCNTs and PANI, which results in the change of electronic distribution. From the inset, it can be seen that there are two peaks around 1033 cm−1 and 1003 cm−1, which are considered as the characteristic adsorption of –SO3− symmetric stretching vibration. This reveals that the PSS is successfully modified onto the surface of GR nanosheets. Moreover, when the MWCNTs/PANI are composited with PSS-GR, compared with MWCNTs/PANI, the red-shift of peaks ascribed to the C
C stretching vibration mode of the quinoid ring and benzenoid ring is observed. This may result from the strong interaction between PSS-GR and PANI polymerized on the surface of MWCNTs/PANI composites.39
Fig. 3C shows the UV-vis absorption spectra of GR, PANI, MWCNTs/PANI and MWCNTs/PANI/PSS-GR nanocomposites. It can be seen that the PANI exhibits three absorbance peaks at 341 nm, 435 nm and 763 nm as shown in curve a, which can be attributed to the π–π* transition of benzenoid rings, polaron–π* transition and π–polaron transition, respectively. For the MWCNTs/PANI, the polaron–π* transition shifts to 429 nm, this indicates the interaction between the quinoid ring of PANI and MWCNTs, as shown in curve b. The GR nanosheets display a weak peak at around 270 nm (curve c). In curve d, the absorption at 224 nm is assigned to PSS, which suggests the existence of PSS on the surface of GR nanosheets. Furthermore, the broad peak at about 264–375 nm may be due to the interaction between PANI and PSS-GR nanosheets.
Electrochemical properties
The electrochemical properties of the electrode materials are characterized by cyclic voltammetric (CV) and galvanostatic charge/discharge (GCD) in 1 M H2SO4.
Fig. 4A shows the CV curves of pure PANI, MWCNTs/PANI and theMPG20 nanocomposites at a scan rate of 10 mV s−1 in a three-electrode system. All the curves exhibit typical CV characteristic of pure PANI with two pairs of redox peaks, corresponding to redox transition leucoemeraldine/emeraldine and faradic transformation emeraldine/pernigraniline.35 The current response of MPG20 nanocomposites electrode is higher than those of pure PANI and MWCNTs/PANI, implying that the MPG20 nanocomposites possess an excellent electrochemical activity. Furthermore, the CV loop area of the MPG20 nanocomposite is the largest, which indicates a highest capacitance behaviour. This is a result of the synergistic effect between GR nanosheets and PANI. The CV curves of MPG20 nanocomposites at different scan rates are shown in Fig. 4B. It is noted that the redox current increases clearly with the increasing of scan rates, this indicates a good rate capacity of the electrode materials. Besides, the cathodic peaks shift positively and the anodic peaks shift negatively with the increasing scan rates, which is mainly due to the resistance of electrode materials.36 The CV curves of MPG20 in a two-electrode system are shown in Fig. S4.† Although the redox peaks are not as obvious as those in the three-electrode system, the change trend is consistent with the results of the three-electrode system.
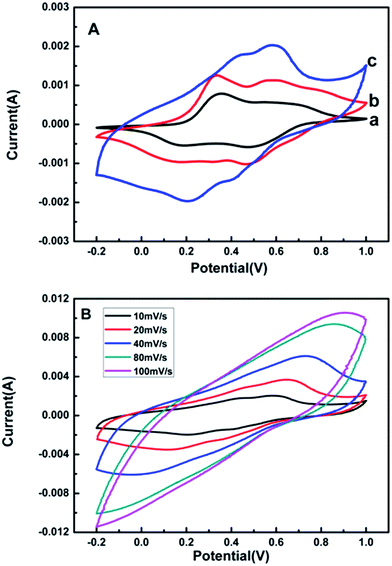 |
| Fig. 4 CV curves of pure PANI (a), MWCNTs/PANI (b), MPG20 nanocomposites (c) at 10 mV s−1 in 1 M H2SO4 (A); CV curves of MPG20 nanocomposites at different scan rates (B) (three-electrode system). | |
To further demonstrate the capacitance behaviours of each electrode material, GCD tests are performed in a three-electrode system at different current densities of 0.5, 1, 2, 4 and 10 A g−1 between 0 and 0.8 V, as exhibited in Fig. 5A–C. The specific capacitance (SC) is calculated according to eqn (1),
|
 | (1) |
where
Cm is the specific capacitance (F g
−1),
I is the charge/discharge current (A),
t is the time for discharging (s), Δ
V is the potential change during discharge process, and
m is the mass of active material within the electrode (g).
40
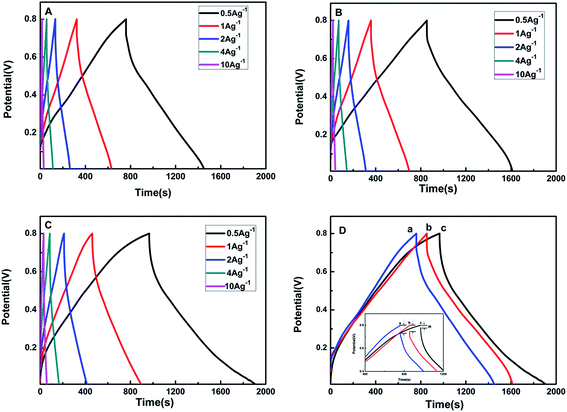 |
| Fig. 5 GCD curves of pure PANI (A), MWCNTs/PANI (B) and MPG20 (C) at the different current density; GCD curves of pure PANI (a), MWCNTs/PANI (b) and MPG20 (c) at the current density of 0.5 A g−1 in 1 M H2SO4 (D), the inset is the magnification at high potential region (three-electrode system). | |
Fig. 5D presents the GCD curves of pure PANI, MWCNTs/PANI and MPG20 nanocomposites at a current of 0.5 A g−1. The calculated SCs are 435.75 F g−1, 476.98 F g−1, 602.5 F g−1, respectively. Moreover, the SCs of MPG10 and MPG30 are 570.2 F g−1 and 461 F g−1 (Fig. S5A†). The largest SC of MPG20 nanocomposites may be due to two reasons. Firstly, the existence of GR nanosheets can enhance the specific surface area of the nanocomposites (SSAs of the PANI, MWCNTs/PANI and MPG20 are 25.97 m2 g−1, 34.83 m2 g−1 and 50.25 m2 g−1, respectively Fig. S6†), and eventually increases the electrical double-layer capacitance. Secondly, the GR nanosheets on the surface of PANI will not hinder the transfer of proton and electron between the electrolyte and PANI, this can retain a larger pseudocapacitor of PANI. However, the MPG10 electrode exhibits smaller SC than that of MPG20 electrode, which is due to small amount of GR nanosheets can only produce small double-layer capacitance and the synergistic effect between PANI and GR is weaker. MPG30 electrode possesses a large number of GR, which will hinder the electron transfer between PANI and electrolyte, as well as decrease the pseudocapacitance performance of PANI. As a result, MPG30 exhibits a relatively low specific capacitance, which is even lower than that of MWCNTs/PANI. The IR drop is related to the internal resistance of the electrode materials. The smallest IR drop can be observed for the MPG20 nanocomposites, indicating that the introduction of GR can reduce internal resistance significantly.
The SC curves of these three electrode materials corresponding to different current densities are displayed in Fig. 6A. The maximal SC value for MPG20 nanocomposites (602.5 F g−1) at current density of 0.5 A g−1 is 38.3% higher than that of the pure PANI (435.75 F g−1) and 26.3% higher than that of the MWCNTs/PANI (476.98 F g−1). Furthermore, the SC of MPG20 nanocomposites still retains 68.6% (from 602.5 F g−1 to 413.4 F g−1) as current density increases from 0.5 A g−1 to 10 A g−1, which indicates a great possibility for high-power application, whereas the pure PANI and MWCNTs/PANI keep 42.8% and 54.9% of their capacity in the same current density range. The cycling stability is examined by a large number of charge–discharge cycles at the current density of 1 A g−1 as shown in Fig. 6B. The MPG20 nanocomposites are found to exhibit excellent stability after 2000 cycles and the capacitance decreases only 21.68% of initial capacitance. On the contrary, up to 59.82% and 39.45% of initial capacitance decreases are found for pure PANI and MWCNTs/PANI composites. Therefore, the “sandwich-like” MPG20 nanocomposites possess better cycling stability over 2000 charge–discharge cycles with good electrochemical performance. The cycling stability of MPG10 and MPG30 are 70.28% and 81.15%, respectively (Fig. S5B†).
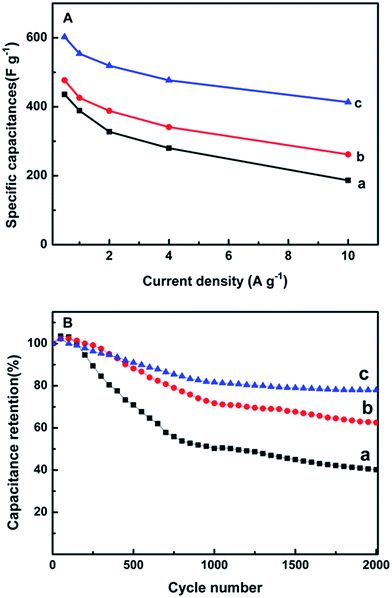 |
| Fig. 6 Specific capacitances of pure PANI (a), MWCNTs/PANI (b) and MPG20 (c) at different current densities in 1 M H2SO4 (A); cycling stability of pure PANI (a), MWCNTs/PANI (b) and MPG20 (c) at the current density of 1 A g−1 in 1 M H2SO4 (B) (three-electrode system). | |
To further assess the feasibility of the as-obtained MPG20 hybrid, a symmetric two-electrode configuration device (Fig. S2†) was assembled and tested (Fig. S4 and S7†). The specific capacitance is calculated according to eqn (2),
|
 | (2) |
where
Cm is the specific capacitance (F g
−1),
I is the charge/discharge current (A),
t is the time for discharging (s), Δ
V is the potential change during discharge process, and
m is the total mass of active material in both electrodes (g).
41 The SC of MPG20 is as high as 332.5 F g
−1 at 0.5 A g
−1 and decreases to 201.6 F g
−1 when the current density increases to 10 A g
−1. By comparison, it can be seen that the SC of MPG20 obtained from the two-electrode system is lower than that measured in the three-electrode system and declines more rapidly with the increase of current density, which is reasonable due to the difference in the capacitor configuration for the electrochemical measurement and the increased amount of the electrode materials in the symmetric two-electrode system. The total mass of MPG20 in the two-electrode system is 18 times higher than that on a GCE in a three-electrode system. The SC of MPG20 nanocomposites in two-electrode system retains 69.58% of initial capacitance at the current density of 1 A g
−1 after 2000 cycles (Fig. S7B
†).
Conclusions
In this paper, a novel electrode material for supercapacitors based on MWCNTs/PANI coated on the surface of GR nanosheets modified by PSS is successfully constructed. The electrochemical properties of the electrode material is greatly enhanced by the synergistic effect of PSS-GR nanosheets, MWCNTs and PANI. The outer GR nanosheets serve as current collector to improve the electrical conductivity and a protective material to suppress the volumetric swelling and shrinking during charge/discharge cycling of PANI. The inner MWCNTs act as rigid cores to improve the mechanical stability and a structural support to create a three-dimensional network structure, which is conducive to the spread of the electrolyte ion between the electrolyte and the electrode. The obtained electrode material exhibits an excellent electrochemical performance with high specific capacitance of 602.5 F g−1 at 0.5 A g−1 in a three-electrode system, and 332.5 F g−1 at 0.5 A g−1 in a symmetric two-electrode system. The nanocomposites also exhibit good rate capability (68.6% capacity retention at 10 A g−1) and excellent cycling stability (21.68% capacity loss after 2000 cycles), which indicates the MPG20 nanocomposites may be a promising electrode material for the actual supercapacitors applications.
Acknowledgements
This work is financially supported by National Natural Science Foundation of China (Grant No: 51371129 and 11174226), Hubei Science and Technology Supported Project (YJG0261), Wuhan Science and Technology Research Project (2014010101010002), the key project of Guangdong Province (2013B090500078).
References
- B. Wang, J. Qiu, H. Feng and E. Sakai, Preparation of graphene oxide/polypyrrole/multi-walled carbon nanotube composite and application in supercapacitors, Electrochim. Acta, 2015, 151, 230–239 CrossRef CAS.
- C. Yang, Z. Chen, I. Shakir, Y. Xu and H. Lu, Rational synthesis of carbon shell coated polyaniline/MoS2 monolayer composites for high-performance supercapacitors, Nano Res., 2016, 9, 951–962 CrossRef CAS.
- W. Dai, L. Ma, M. Gan, S. Wang, X. Sun, H. Wang, H. Wang and T. Zhou, Fabrication of sandwich nanostructure graphene/polyaniline hollow spheres composite and its applications as electrode materials for supercapacitor, Mater. Res. Bull., 2016, 76, 344–352 CrossRef CAS.
- X. Liu, Y. Zheng and X. Wang, Controllable preparation of polyaniline-graphene nanocomposites using functionalized graphene for supercapacitor electrodes, Chem.–Eur. J., 2015, 21, 10408–10415 CrossRef CAS PubMed.
- Y. Wang, H. Li and Y. Xia, Ordered whisker like polyaniline grown on the surface of mesoporous carbon and its electrochemical capacitance performance, Adv. Mater., 2006, 18, 2619–2623 CrossRef CAS.
- D. Lv, j. Shen and G. Wang, A post-oxidation strategy for the synthesis of graphene/carbon nanotube-supported polyaniline nanocomposites as advanced supercapacitor electrode, RSC Adv., 2015, 5, 24599–24606 RSC.
- D. Cai, H. Huang, D. Wang, B. Liu, L. Wang, Y. Liu, Q. Li and T. Wang, High-performance supercapacitor electrode based on the unique ZnO@Co3O4 core/shell heterostructures on nickel foam, ACS Appl. Mater. Interfaces, 2014, 6, 15905–15912 CAS.
- X. Zhang, Z. Zhou and G. Lu, Reductant- and stabilizer-free synthesis of graphene-polyaniline aqueous colloids for potential waterborne conductive coating application, RSC Adv., 2015, 5, 20186–20192 RSC.
- L. Tang, F. Duan and M. Chen, Silver nanoparticles decorated polyaniline/multiwalled super-short carbon nanotube nanocomposites for supercapacitor application, RSC Adv., 2016, 6, 65012–65019 RSC.
- T. Liu, L. Finn, M. Yu, H. Wang, T. Zhai, X. Lu, Y. Tong and Y. Li, Polyaniline and polypyrrole pseudocapacitor electrodes with excellent cycling stability, Nano Lett., 2014, 14, 2522–2527 CrossRef CAS PubMed.
- N. Hu, L. Zhang, C. Yang, J. Zhao, Z. Yang, H. Wei, H. Liao, Z. Feng, A. Fisher, Y. Zhang and Z. J. Xu, Three-dimensional skeleton network of graphene wrapped polyaniline nanofiber: an excellent structure for high-performance flexible solid-state supercapacitors, Sci. Rep., 2016, 6, 1–10 Search PubMed.
- W. Fan, Y.-E. Miao, L. Zhang, Y. Huang and T. Liu, Porous graphene-carbon nanotube hybrid paper as a flexible nano-scaffold for polyaniline immobilization and application in all-solid-state supercapacitors, RSC Adv., 2015, 5, 31064–31073 RSC.
- W. Zhao, S. Wang, C. Wang, S. Wu, W. Xu, M. Zou, A. Ouyang, A. Cao and Y. Li, Double polymer sheathed carbon nanotube supercapacitors show enhanced cycling stability, Nanoscale, 2016, 8, 626–633 RSC.
- X. Fan, Z. Yang and Z. Liu, One-step synthesis of graphene/polyaniline nanotube composite for supercapacitor electrode, Chin. J. Chem., 2016, 34, 107–113 CrossRef CAS.
- W. Zhao, Y. Li, S. Wang, X. He, Y. Shang, Q. Peng, C. Wang, S. Du, X. Cui, Y. Yang, Q. Yuan, E. Shi, S. Wu, W. Xu and A. Cao, Elastic improvement of carbon nanotube sponges by depositing amorphous carbon
coating, Carbon, 2014, 76, 19–26 CrossRef CAS.
- Ka. Y. T. Lee, H. T. H. Shi, K. Lian and H. E. Naguib, Flexible multiwalled carbon nanotubes/conductive polymer composite electrode for supercapacitor application, Smart Mater. Struct., 2015, 24, 1–17 Search PubMed.
- R. Sainz, A. M. Benito, M. T. Martinez, J. F. Galindo, J. Sotres, A. M. Baro, B. Corraze, O. Chauvet and W. K. Maser, Soluble self-aligned carbon nanotube/polyaniline composites, Adv. Mater., 2015, 17(3), 278–281 CrossRef.
- S. Wang, L. Ma, M. Gan, S. Fu, W. Dai, T. Zhou, X. Sun, H. Wang and H. Wang, Free-standing 3D graphene/polyaniline composite film electrodes for high-performance supercapacitors, J. Power Sources, 2015, 299, 347–355 CrossRef CAS.
- Y. Tan and J.-M. Lee, Graphene for supercapacitor applications, J. Mater. Chem. A, 2013, 14814–14843 CAS.
- Q. Wu, Y. Xu, Z. Yao, A. Liu and G. Shi, Supercapacitors based on flexible/polyaniline nanofiber composite films, ACS Nano, 2010, 4, 1963–1970 CrossRef CAS PubMed.
- T. Fan, S. Tong, W. Zeng, Q. Niu, Y. Liu, C. Kao, J. Liu, W. Huang, Y. Min and A. J. Epstein, Self-assembling sulfonated grapheme/polyaniline nanocomposite paper for high performance supercapacitor, Synth. Met., 2015, 199, 79–86 CrossRef CAS.
- H. Liu, X. Han, F. Qiu and J. Yang, Facile route to covalently-jointed graphene/polyaniline composite and it's enhanced electrochemical performances for supercapacitors, Appl. Surf. Sci., 2016, 376, 261–268 CrossRef.
- K.-Y. Shin, S. Cho and J. Jang, Graphene/polyaniline/poly(4-styrenesulfonate) hybrid film with uniform surface resistance and its flexible dipole tag antenna application, Small, 2013, 22, 3792–3798 CrossRef PubMed.
- Y. Li, J. Tang, L. Huang, Y. Wang, J. Liu, X. Ge, S. C. Tjong, R. K. Y. Li and L. A. Belfiore, Facile preparation, characterization and performance of noncovalently functionalized graphene/epoxy nanocomposites with poly(sodium 4-styrenesulfonate), Composites, Part A, 2015, 68, 1–9 CrossRef CAS.
- W. Yan, W.-J. Yu, L. Wang, D. Zhang, X.-Q. Ge, J.-Z. Hang, W. Deng and Li-Y. Shi, Preparation of partially reduced graphene oxide nanosheets/poly(sodium 4-styrenesulfonate) composites with high capacitance, Electrochim. Acta, 2014, 147, 257–264 CrossRef CAS.
- X. Feng, H. Cheng, Y. Pan and H. Zheng, Development of glucose biosensors based on nanostructured graphene-conducting polyaniline composite, Biosens. Bioelectron., 2015, 70, 411–417 CrossRef CAS PubMed.
- Y. Meng, K. Wang, Y. Zhang and Z. Wei, Hierarchical porous graphene/polyaniline composite film with superior rate performance for flexible supercapacitors, Adv. Mater., 2013, 25, 6985–6990 CrossRef CAS PubMed.
- S. Cho, J. S. Lee, J. Jun, S. G. Kim and J. Jang, Fabrication of water-dispersible and highly conductive PSS-doped PANI/graphene nanocomposites using a high-molecular weight PSS dopant and their application in H2S detection, Nanoscale, 2014, 6, 15181–15195 RSC.
- B. Song, C.-C. Tuan, X. Huang, L. Li, K.-S. Moon and C.-P. Wong, Sulfonated polyaniline decorated graphene nanocomposites as supercapacitor electrodes, Mater. Lett., 2016, 166, 12–15 CrossRef CAS.
- T. Wu, X. Xu, L. Zhang, H. Chen, J. Gao and Y. Liu, A polyaniline/graphene nanocomposite prepared by in situ polymerization of polyaniline onto polyanion grafted graphene and its electrochemical properties, RSC Adv., 2014, 4, 7673–7681 RSC.
- S. Ebrahim, R. EI-Raey, A. Hefnawy, H. Ibrahim, M. Soliman and T. M. Abdel-Fattah, Electrochemical sensor based on polyaniline nanofibers/single wall carbon nanotube composite for detection of malathion, Synth. Met., 2014, 190, 13–19 CrossRef CAS.
- S. Dhibar, P. Bhattacharya, G. Hatui, S. Sahoo and C. K. Das, Transition metal-doped polyaniline/single-walled carbon nanotube nanocomposites: efficient electrode material for high performance supercapacitors, ACS Sustainable Chem. Eng., 2014, 2, 1114–1127 CrossRef CAS.
- M. Ding, Y. Tang, P. Gou, M. J. Reber and A. Star, Chemical sensing with polyaniline coated single-walled carbon nanotube, Adv. Mater., 2011, 23, 536–540 CrossRef CAS PubMed.
- G. V. Ramana, V. V. S. S. Srikanth, B. Padya and P. K. Jain, Carbon nanotube - polyaniline nanotube core-shell structures for electrochemical applications, Eur. Polym. J., 2014, 57, 137–142 CrossRef CAS.
- H. Liu, W. Zhang, H. Song, X. Chen, J. Zhou and Z. Ma, Tremella-like graphene/polyaniline spherical electrode material for supercapacitors, Electrochim. Acta, 2014, 146, 511–517 CrossRef CAS.
- J.-W. Kim, E. J. Siochi and J. Carpena-Nunez, et al. Polyaniline/carbon nanotube sheet nanocomposite: fabrication and characterization, ACS Appl. Mater. Interfaces, 2013, 5, 8597–8606 CAS.
- S. Zhou, H. Zhang, X. Wang, J. Li and F. Wang, Sandwich nanocomposites of polyaniline embedded between graphene layers and multi-walled carbon nanotubes for cycle-stable electrode materials of organic supercapacitors, RSC Adv., 2013, 3, 1797–1807 RSC.
- J. Wu and L. Yin, Platinum Nanoparticle Modified Polyaniline-Functionalized Boron Nitride Nanotubes for Amperometric Glucose Enzyme Biosensor, Appl. Mater. Interfaces, 2011, 3, 4354–4362 CrossRef CAS PubMed.
- S. Liu, X. Liu, Z. Li, S. Yang and J. Wang, Fabrication of free-standing graphene/polyaniline nanofibers composite paper via electrostatic adsorption for electrochemical supercapacitors, New J. Chem., 2011, 35, 369–374 RSC.
- A. Ubul, R. Jamal, A. Rahman, T. Awut, I. Nurulla and T. Abdiryim, Solid-state synthesis and characterization of polyaniline/multi-walled carbon nanotube composite, Synth. Met., 2011, 161, 2097–2102 CrossRef CAS.
- S. Gao, P. Zang, L. Dang, H. Xu, F. Shi, Z. Liu and Z. Lei, Extraordinarily high-rate capability of polyaniline nanorod arrays on graphene nanomesh, J. Power Sources, 2016, 304, 111–118 CrossRef CAS.
Footnote |
† Electronic supplementary information (ESI) available. See DOI: 10.1039/c6ra20435j |
|
This journal is © The Royal Society of Chemistry 2016 |