DOI:
10.1039/C6RA19521K
(Paper)
RSC Adv., 2016,
6, 80493-80500
EPS solubilization and waste activated sludge acidification enhanced by alkaline-assisted bi-frequency ultrasonic pretreatment revealed by 3D-EEM fluorescence
Received
2nd August 2016
, Accepted 17th August 2016
First published on 17th August 2016
Abstract
The effect of alkaline-assisted bi-frequency (28 + 40 kHz) ultrasonic pretreatment on extracellular polymeric substances (EPS) solubilization and waste activated sludge (WAS) acidification was investigated. Experimental results showed that WAS disintegration and (volatile fatty acids) VFAs production were effectively improved by the pretreatment. The optimal ultrasonic treatment time and alkaline dosage were 45 min and 0.04 g NaOH per g TS. Under this optimal condition, a lysis rate of WAS of 36.8% was achieved, and the corresponding increased solute protein and carbohydrate concentrations were 4173 and 853 mg L−1, respectively. Three-dimensional excitation–emission matrix (3D-EEM) fluorescence indicated that the pretreatment applied in this study could effectively decompose the tightly-bound EPS matrix, located in the inner parts of sludge flocks, and further destroy the microbial cell wall. The detachment of EPS and release of intracellular substrates provided sufficient substrates for anaerobic acid-producing microorganisms, which evidently improved the subsequent VFA production from WAS fermentation. The maximum VFA concentration was 10
816 ± 275 mg COD per L (457.1 g COD per g VS) for 72 h fermentation time, which was 5.4-fold higher than that obtained for the un-pretreated WAS.
1. Introduction
With the development of the national economy and the explosion of the urban population, the discharged quantity of wastewater is increasing sharply in China. As the main solid waste produced during wastewater treatment, large amounts of waste activated sludge (WAS) are thereby accumulated. In 2013, 6.25 million tons of dry sludge (moisture content 80%) were produced in China, and still shows a rapidly increasing rate with an annual growth of 13%.1 However, the main methods to deal with WAS are landfill (77%) and land application (15%), which have their own environmental side-effects.2 WAS has been known as an abundant, inexpensive, and renewable source of organics. Proteins and carbohydrates are the main components, accounting for ∼60% and ∼15% of the total chemical oxygen demand (TCOD) of WAS.3 Recently, rather than being discarded as a waste, the conversion of WAS into high-value products and/or cheap energy resources, such as volatile fatty acids (VFAs), hydrogen, methane, etc., is starting to be considered with increasing interest.4–6 As a kind of high added-value chemical material, VFA production from WAS digestion has been proven to be a feasible and effective carbon resource recovery process, which produces promising substrates for biopolymer production,7 bioenergy generation8–10 and biological nutrient removal.11
Microbial conversion from WAS to valuable products is an innovative, cost-effective and promising way to gain social and economic benefits. Anaerobic digestion is acknowledged as the most cost-effective way for WAS treatment.12 However, organics in sludge bioaggregates mainly embedded in microbial cells or immobilized in extracellular polymeric substances (EPS).13 Due to the natural barrier of the cell wall and EPS matrix, the hydrolysis is regarded as the rate-limiting steps for WAS treatment. Only little of TCOD of WAS can be biodegraded unless the particulate organic matters are significantly solubilized. Thus, pretreatment is a prerequisite, in order to keep operations within an industrially acceptable time-frame. Many approaches involving chemical,14–16 biological17,18 and physicochemical19–21 methods have therefore been developed. Alkaline has been proved to be a potential way for improving VFAs production from WAS fermentation, which resulted in positive effects on not only the enhancement of hydrolysis but also the inhibition of methanogens.22 Moreover, it is of great strategic significance for its cost effective and operation convenient characteristics. However, although VFAs production can be improved under alkaline condition (normally at pH 10), there is still half of particulate organics could not be degraded.22 Ultrasonic pretreatment of sludge can disintegrate its flocs structure and release extracellular proteins, polysaccharides and enzymes in different sludge layers.13,23 Yan et al. (2010) utilized the ultrasonic pretreatment (20 kHz) combined with alkaline adjustment (pH 10.0) on WAS treatment and accumulated 3110 mg VFAs (in COD) per L successfully.23 However, strong alkaline adjustment during the WAS fermentation need sustained addition of alkaline and unstable, which increased the difficulty of process control. Meanwhile, compared with mono-frequency ultrasonic, our previous study showed bi-frequency ultrasonic was regarded as an alternative and more efficient strategy for WAS disintegration.20 Therefore, it is surmised that alkaline-assisted bi-frequency ultrasonic pretreatment could further harvest improvement of VFAs production from WAS. EPS in sludge rather than cells represent the major organic fraction determining flocs structure, integrity and strength. The biochemical composition of EPS is a critical factor to explore the destruction mechanisms of WAS enhanced by pretreatment. The majority of dissolved organic matter (DOM) is represented by soluble microbial products originated from bound EPS, which is ubiquitous in wastewater and sludge.24 Thus, characterization of EPS and DOM would be useful for understanding the WAS destruction mechanisms after pretreatment. Excitation–emission matrix (EEM) fluorescence spectroscopy, coupled with parallel factor analysis (PARAFAC), is an established tool for organic matter fingerprinting in aqueous systems.25 Yet, processes of EPS solubilization functioning at this coupling pretreatment have not been completely explored.
The main objective of this study is to evaluate the feasibility of bi-frequency (28 + 40 Hz) ultrasonic pretreatment integrated into alkaline for enhancing VFAs production from WAS fermentation. The mechanisms of proposed method were explored by analyzing extracellular polymeric substances (EPSs) in different layers by excitation–emission matrix (EEM) fluorescence. The operation parameters of alkaline-assisted bi-frequency ultrasonic pretreatment were optimized. VFAs production and solute organics variation during WAS fermentation under the optimum pretreatment was investigated. The findings obtained in this work may provide a scientific basis for the potential implementation to solve the shortage of easily-degradable carbon source of a wastewater treatment plant.
2. Material and methods
2.1 Source of waste activated sludge and pretreatment procedure
WAS used in this study was obtained from the secondary sedimentation tank of Taiping municipal wastewater treatment plant in Harbin, China. The sludge was concentrated by settling at 4 °C for 24 h and its main characteristics are shown in Table 1. The ultrasonicator used in the study was customized in Ningbo Scientz Biotechnology Co., China, which could generate bi-frequency ultrasound of 28 + 40 kHz. The working volume is 2.0 L with an inner groove of 14.4 × 14.4 × 14.4 cm. Fig. 1 shows the schematic diagram of bi-frequency ultrasonicator. Ultrasonic pretreatment was performed at room temperature (21 ± 1 °C) at an energy density of 1.0 kW L−1. Ultrasonic time was controlled at 15, 30, 45 and 60 min. Alkaline dosages were 0.02, 0.04, 0.09 g NaOH per g TS, the corresponding pH values were about 10, 11 and 12. The control tests were conducted without pretreatment simultaneously. The operating parameters of alkaline-assisted bi-frequency pretreatment were optimized based on the performance of WAS hydrolysis.
Table 1 Characteristics of concentrated WAS
Parameter |
Value |
pH |
6.61 |
Total solids (TS)/(mg L−1) |
34 600 |
Volatile solids (VS)/(mg L−1) |
23 660 |
Total chemical oxygen demand (TCOD)/(mg L−1) |
29 970 |
Soluble chemical oxygen demand (SCOD)/(mg L−1) |
666 |
Soluble proteins/(mg COD per L) |
195 |
Soluble carbohydrates/(mg COD per L) |
50 |
 |
| Fig. 1 Schematic diagram of coupling pretreatment and anaerobic fermentation experiments. | |
2.2 Batch fermentation experiments setup and operations
WAS anaerobic fermentation experiments were conducted in a series of completely stirred tank reactors (CSTRs) (Fig. 1). The reactor consisted of two Plexiglass cylinders (120 mm and 160 mm diameters), the inner cylinder was used for fermentation with a volume of 3.0 L and a headspace of 1.0 L. The temperature of 35 ± 2 °C was maintained by circulating water from a water bath heating vessel. The mixing was carried out by a controlled electric stirrer. Nitrogen gas was flushed to remove oxygen, and all reactors were capped, sealed, and stirred at 100 rpm. All the fermentation experiments were carried out in triplicate.
2.3 Analytical methods
Sludge samples were centrifuged at 10
000 rpm, then supernatant through a 0.45 μm cellulose nitrate membrane filter and finally stored at 4 °C prior to analysis. The determinations of TS, VS, TCOD and SCOD have been performed according to standard methods.26 The pH value was measured by a pH meter (Seven Multi, Mettler Toledo, Switzerland). According to a previous study, carbohydrates and proteins were measured by the phenol-sulfuric and bicinchoninic acid methods with glucose and bull serum albumin as the standards, respectively.3 To analyze VFAs, the filtrate was collected in a 1.5 mL gas chromatography vial and 3% H3PO4 was added to adjust the pH to approximately 4.0. An Agilent 7890GC with flame ionization detector was utilized to analyze the concentration and composition of VFAs. VFAs production was calculated as the sum of the measured HAc, HPr, n-HBu, iso-HBu, n-HVa and iso-HVa. The COD conversion factors are 1.50 g COD per g protein (assumed as (C4H6.1O1.2N)x), 1.06 g COD per g carbohydrate (assumed as C6H12O6), 1.07 g COD per g HAc, 1.51 g COD per g HPr, 1.82 g COD per g HBu, and 2.04 g COD per g HVa.
The lysis rate for WAS solubilization was calculated as follows:
|
 | (1) |
where SCOD
t denotes SCOD value of pretreated WAS, SCOD
0 and TCOD
0 represents respectively SCOD and TCOD values of raw WAS.
2.4 Ultrasound protocol for EPS extraction of WAS samples
Three sludge layers (dissolved organic matter (DOM), loosely bound EPS (LB-EPS) and tightly bound EPS (TB-EPS)) were prepared and extracted in the different sludge samples. The WAS filtrate (0.45 μm cellulose nitrate membrane filter) was used for the analysis of DOM. The collected sediments were re-suspended to their original volume using a pH 7 buffer solution (1.3 mM Na3PO4, 2.7 mM NaH2PO4, 6 mM NaCl and 0.7 mM KCl). The suspensions were centrifuged again at 5000 g for 15 min. The supernatant represented the LB-EPS. Collected sediments were re-suspended again with buffer solution and then treated using ultrasound at 20 kHz and 480 W for 10 min. The extracted solutions were centrifuged at 20
000g for 20 min. The supernatant represented the TB-EPS.
2.5 EEM fluorescence analysis
The fluorescence excitation–emission matrix (EEM) was measured using a fluorescence spectrometry (FP-6500, Jasco, Tokyo, Japan). EEM spectra were obtained by scanning emission spectra from 220 nm to 600 nm at 2 nm increments, with excitation wavelengths from 220 nm to 450 nm at 5 nm intervals. The slit widths for emission and excitation were 5 nm and 5 nm, respectively, and the scanning speed was set at 1000 nm min−1 for all measurements. Parallel factor analysis (PARAFAC) was used to model EEM fluorescence data in our previous study.27 Before PARAFAC analysis, the Raman scattering was removed by subtracting the pure distilled water spectrum from the sample spectrum, and the Rayleigh scattering was overcome by inserting a series of zero values in the region of no fluorescence (excitation wavelength ≪ emission wavelength). The analysis of residuals (sum of squared error) in both, the excitation and emission dimensions, was used to find out the appropriate number of PARAFAC components. The software Matlab 2012a (MathWorks Inc., USA) was employed for handling EEM data.
3. Result and discussion
3.1 Enhanced WAS solubilization
Lysis rate of WAS presented the extent of cell wall broken and the release of intracellular organic matter into liquid phase by pretreatment. The effect of ultrasonic treatment time and alkaline dosages on lysis rate is shown in Fig. 2. As can be seen from the figure, alkaline dosage played a predominant role over the ultrasonic time. With alkaline dosage of 0.02 g NaOH per g TS, no matter how long ultrasonic time was, the lysis rates were all lower than 25%, which was not satisfactory. With the increase of alkaline dosage, the lysis rate apparently promoted. Taking ultrasonic time of 45 min as example, the lysis rate increased from 23 ± 5% (0.02 g NaOH per g TS) to 37 ± 4% (0.04 g NaOH per g TS) and further to 41 ± 4% (0.09 g NaOH per g TS). Apparently, lysis rate was not enhanced substantially at alkaline dosage >0.04 g NaOH per g TS.
 |
| Fig. 2 Effect of ultrasonic time and alkaline dosage on lysis rate of WAS (note: error bars represent standard deviation). | |
3.2 Proteins and carbohydrates concentrations in different layers of sludge flocs
As proteins and carbohydrates were the main constituents of WAS, the solubilization of soluble organics can be expressed by that of proteins and carbohydrates. As shown in Fig. 3A, with the same alkaline dosage, solute protein concentration increased with ultrasonic treatment time while stayed constant after 45 min. With alkaline dosage of 0.02, 0.04 and 0.09 g NaOH per g TS, solute proteins concentrations were 2629, 4172 and 5377 mg COD per L, respectively. As has been recognized, microbial cells and EPS are the main components of bio-aggregates, such as bio-films and sludge flocs.28 Pretreatment could not only break cell wall of sludge microorganism to release intracellular organic matter, but also destroyed the flocculated structure of EPS. Moreover, it is believed that the disruption of EPS matrix could enhance the rate and extent of sludge biodegradation during anaerobic digestion.29 By analyzing proteins concentrations in the LB-EPS and TB-EPS under different pretreatment conditions, the extent of solubilization by alkaline-assisted bi-frequency ultrasonic pretreatment would be realized. As shown in Fig. 3B, with the alkaline dosage increased, the concentrations of increased proteins were negative, which meant the inner particulate proteins dissolved into the liquid phase. By contrast, the proteins concentrations in the LB-EPS were indeed increased (Fig. 3C). The reasons behind here maybe that bound EPS exhibited a dynamic double-layer-like structure, which is composed of LB-EPS and TB-EPS,30,31 the TB-EPS layer was tightly attached to the cell surface, due to the disintegration effect caused by ultrasonic cavitation, in which matrix the extracellular polymeric organics enmeshed were released. On the contrary, the LB-EPS layer was characterized by loose structure and rheological property. It easily adsorbed the intracellular and TB-EPS-released organics which resulted in the increase of the carbohydrates and proteins concentrations in the LB-EPS layer. It also can be seen from Fig. 3 that the increased amounts of soluble proteins were far above the released amount of bound EPS after the coupling pretreatment. This proved that the pretreatment used in this study had disrupted microbial cells and released intracellular substrates into the liquid. Meanwhile, with the increase of alkaline dosage, taking ultrasonic treatment time of 45 min as example, the increased proteins concentrations in the LB-EPS were 83 ± 5, 814 ± 53 and 1203 ± 124 mg COD per L with the alkaline dosages of 0.02, 0.04 and 0.09 g NaOH per g TS, respectively. However, proteins concentrations increased in a smaller amplitude in the LB-EPS as ultrasonic time increased under the same alkaline dosage, which indicated that alkaline plays an important role in breaking flocculated structure of EPS.
 |
| Fig. 3 Effect of ultrasonic time and alkaline dosage on carbohydrates concentrations in different WAS layers: (A) DOM; (B) TB-EPS; (C) LB-EPS (note: error bars represent standard deviation). | |
The effect on carbohydrates concentrations in different layers of WAS pretreated by alkaline-assisted bi-frequency ultrasonic technology was similar with that on the proteins (Fig. 4). The increase of alkaline dosage was also closely related to the increase of carbohydrates concentrations in the LB-EPS and TB-EPS layers. Differently, when ultrasonic treatment time exceeded to 45 min, alkaline dosage had little influence on the carbohydrates concentrations in the supernatant. Taking ultrasonic treatment time of 45 min as example, the soluble carbohydrate concentrations were 745 ± 69, 853 ± 144 and 862 ± 19 mg L−1 with alkaline dosages of 0.02, 0.04 and 0.09 g NaOH per g TS, respectively, which can prove that the bi-frequency ultrasonic has a dominant effect on carbohydrate dissolution.
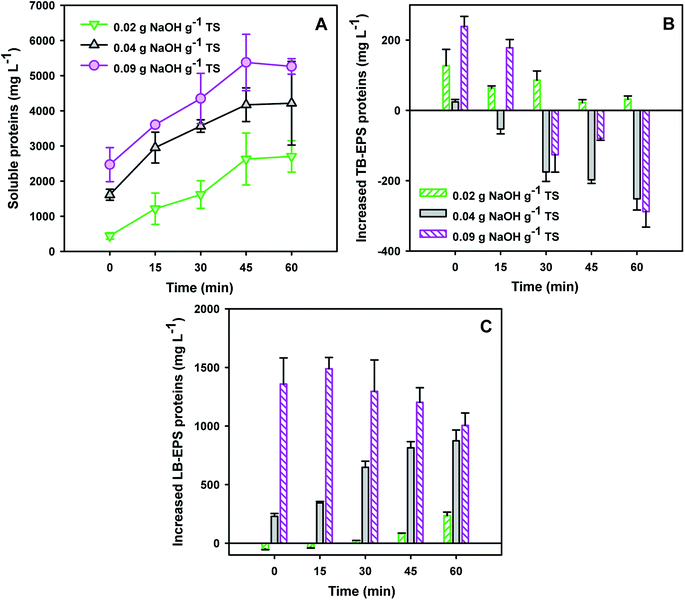 |
| Fig. 4 Effect of ultrasonic time and alkaline dosage on proteins concentrations in different WAS layers: (A) DOM; (B) TB-EPS; (C) LB-EPS (note: error bars represent standard deviation). | |
Based on the pretreatment results under different ultrasonic time and alkaline dosages, taking lysis rate, soluble proteins and carbohydrates concentrations in the sludge supernatant as the research objects, the following conclusions can be drawn: firstly, they all basically showed increasing trend with the increase of ultrasonic treatment time. However, they rose slowly and even had a slight decline after 45 min, which demonstrated that the suitable ultrasonic treatment time was 45 min; secondly, alkaline dosage played an important role on promoting the disintegration performance of the microbial cell wall and the floc structure of EPS. They all increased with the increasing of alkaline dosage. When the alkaline dosage was 0.09 g NaOH per g TS, the maximum WAS solubilization performance could be achieved. However, compared with that in the 0.04 g NaOH per g TS test, the efficiencies increased less than 20% when the ultrasonic time was 45 min. Consequently, taking economic cost into consideration, the alkaline dosage of 0.04 g NaOH per g TS was more appropriate. Moreover, the pH value of pretreated WAS in the 0.09 g NaOH per g TS test reached to 12, which was far beyond the tolerance range of the acidogenic bacteria and would inhibit their activity. Based on the above analysis, the optimal pretreatment conditions are: 28 + 40 Hz bi-frequency ultrasonic treatment for 45 min with an alkaline dosage of 0.04 g NaOH per g TS.
3.3 Characterization of pretreated WAS by EEM spectra with PARAFAC
Three-dimensional EEM spectroscopy was applied for characterizing the component changes of WAS, in terms of dissolved organic matter (DOM), loosely-bound EPS (LB-EPS) and tightly-bound EPS (TB-EPS), under the optimal treatment conditions (0.04 g NaOH per g TS, 45 min) (Fig. 5). Six EEM fluorescence spectra were similar in the peak locations, but had different fluorescence intensities (FI) (Fig. 5A and B). By separating the spectra of the main components from overlapped EEM spectra, three components were found to be appropriate, which were identified as (Com.1) tryptophan-like substances, Ex/Em (275/350); (Com.2) tyrosine-like substances, Ex/Em (275/300); (Com.3) fulvic-like substrates, Ex/Em (320/400) (Fig. 5C).32,33 Obviously, the intensities of DOM were enhanced by the coupling treatment. Protein-like substances, including tryptophan-like and tyrosine-like, were the primary components, with a FI of 298 and 247 under the coupling treatment, compared to only 203 and 151 in the un-pretreated WAS (control). This also revealed the performance of dissolving of proteins, inside the microbial cell or enmeshed in EPS, into liquid phase under the coupling treatment. Moreover, the FI of TB-EPS peaks in EEM spectra were significantly decreased by the coupling treatment, contrastively, that of LB-EPS were increased. Taking tyrosine-like substances into consideration, the FI in the LB-EPS and TB-EPS layers changed simultaneously from 289 and 385 in the control to 693 and 207 in the coupling treatment. Results were in accordance with practical measurements (Fig. 3 and 4). Small amounts of fulvic-acid-like substance were found in both, DOM and EPS fluorescence spectra, which were mainly formed by the microbial degradation of dead organic matter.32
 |
| Fig. 5 EEM fluorescence spectra for DOM, LB-EPS and TB-EPS obtained from (A) control and (B) pretreated WAS with the optimal treatment conditions. Three components of DOM and EPSs decomposed by the PARAFAC approach (C): (Com.1) tryptophan-like substances, Ex/Em (275/350); (Com.2) tyrosine-like substances, Ex/Em (275/300); (Com.3) fulvic-like substrates, Ex/Em (320/400). | |
3.4 VFAs production and organics degradation during WAS fermentation
Fig. 6A shows the time-course of VFAs production and organics degradation from WAS fermentation under the optimal alkaline-assisted bi-frequency ultrasonic pretreatment. VFAs production sharply increased until 72 h, and decreased a little with the further increase of fermentation time. At a fermentation time of 72 h, the maximum VFAs concentration was achieved (10
816 ± 275 mg COD per L, 457.1 g COD per g VS), while that was only 2008 ± 173 mg COD per L (84.9 g COD per g VS) in the control test (96 h). The reason maybe that the coupling pretreatment efficiently disintegrated sludge flocs matrices and hydrolyzed the large molecules organics, which provided more substrates for subsequent anaerobic acidification. Previous researches also explored almost the same approach to produce VFAs by alkaline and ultrasonic methods. Zhuo et al. (2012) obtained 4649 mg VFAs per L (as COD) from the alkaline (pH 10) assisted ultrasonic (20 kHz, 10 min) pretreated WAS.34 Liu et al. (2009) studied the effects of alkaline-ultrasonic (28 kHz, 60 min, pH 12) pretreatment on VFAs production from WAS and obtained 3700 mg VFAs per L (as COD) (224 mg VFAs per g VS), respectively.35 Obviously, the production of VFAs in this study was enhanced by this coupling pretreatment.
 |
| Fig. 6 Time-course of VFAs production (composition), soluble organics and pH value from WAS fermentation with alkaline-assisted bi-frequency ultrasonic pretreatment (note: error bars represent standard deviation). | |
The individual VFA percentage accounting for total VFAs was shown in Fig. 6A for 72 h fermentation time, when the total VFAs had reached a plateau in most of the reactors. VFAs composition is considered crucial when the WAS hydrolysate is used as external carbon source. Among the six VFA, HAc was the favorite substrate for biological nutrients removal.36 As shown in Fig. 6A, HAc formed the major part of total VFAs in the coupling pretreated WAS (53% versus 27% in the control); HPr and iso-HVa represented the second and third most abundant metabolites, respectively. These results seemed to be in accordance with Wang et al. (1999),37 which showed that the top two VFAs were HAc and HPr, no matter which type of sludge pretreatment method was used (ultrasonic, thermal and freezing). This would suggest that the coupling pretreatment used in this study can, indeed, influence the acidification product spectrum. Based on the above considerations of both production and composition, it is ideal to harvest VFAs as target product from WAS digestion by alkaline-assisted bi-frequency ultrasonic pretreatment.
During the anaerobic fermentation, soluble proteins and soluble carbohydrates were both decreased from initially 4172 and 853 mg L−1 to finally 1748 and 345 mg L−1, respectively (Fig. 6B). The appropriate pH range for methanogens is between 6.5 to 7.2,38,39 while that is much wider for acidogenic bacteria, which can generally grow well in the range of 4.0–8.5.40 The initial pH of pretreated WAS prior to anaerobic fermentation was 10.4, which would strongly inhibit the activity of methanogens, and it rapidly went down into the optimum range for acidogenic bacteria within 24 h. Due to rich substrates and inhibited consumption, the production of VFAs reached to a higher level than that in the control test. During the latter fermentation, the pH value gradually trended to neutral, thus the activity of methanogens began to recover, which lead to a slow downward trend of VFAs production after 5 d.
4. Conclusions
WAS disintegration and EPS solubilization successfully improved under alkaline-assisted bi-frequency ultrasonic pretreatment. Considering the economic cost and potential capacity of biocarbon-source recovery in the subsequent anaerobic fermentation, the optimal pretreatment conditions were: 28 + 40 kHz bi-frequency ultrasonic treatment for 45 min with an alkaline dosage of 0.04 g NaOH per g TS. Under this condition, the tightly-bound EPS matrix, located in inner parts of sludge flocks, could be effectively decomposed by 3D-EEM fluorescence analysis. Calculation of organics concentrations in different layers of sludge flocs indicated that this coupling pretreatment further destroyed the microbial cell wall. Meanwhile, this coupling pretreatment exerted positive influence on VFAs production and composition (higher HAc proportion) from WAS. The findings obtained in this work may provide a scientific basis for the potential implementation to solve the shortage of easily-degradable carbon source of a wastewater treatment plant.
Acknowledgements
This research was supported by National Natural Science Foundation of China (NSFC, No. 51378330, 51408396 and 51578534), by State Key Laboratory Breeding Base of Coal Science and Technology Co-founded by Shanxi Province and the Ministry of Science and Technology, Taiyuan University of Technology (No. MKX201504), by China Postdoctoral Science Foundation (No. 2015M570241 and 2016M591416), by the Key Research and Development (R&D) Project of Shanxi Province (No. 201603D321012), by the Major Science and Technology Program for Water Pollution Control and Treatment (No. 2014ZX07204-005) and by International S&T cooperation program (No. S2015GR1012).
References
- G. Yang, G. Zhang and H. Wang, Water Res., 2015, 78, 60–73 CrossRef CAS PubMed.
- A. Bouskova, M. Dohanyos, J. E. Schmidt and I. Angelidaki, Water Res., 2005, 39, 1481–1488 CrossRef CAS PubMed.
- A. Zhou, J. Du, C. Varrone, W. Liu and A. Wang, Process Biochem., 2014, 49, 283–289 CrossRef CAS.
- A. Zhou, Z. Guo, C. Yang, F. Kong, W. Liu and A. Wang, J. Biotechnol., 2013, 168, 234–239 CrossRef CAS PubMed.
- W. Liu, S. Huang, A. Zhou, G. Zhou, N. Ren, A. Wang and G. Zhuang, Int. J. Hydrogen Energy, 2012, 37, 13859–13864 CrossRef CAS.
- R. Sun, D. Xing, J. Jia, A. Zhou, L. Zhang and N. Ren, Bioresour. Technol., 2014, 169, 496–501 CrossRef CAS PubMed.
- Y. Jiang, Y. Chen and X. Zheng, Environ. Sci. Technol., 2009, 43, 7734–7741 CrossRef CAS PubMed.
- S. Kavitha, R. Y. Kannah, I. T. Yeom, K. U. Do and J. R. Banu, Bioresour. Technol., 2015, 197, 383–392 CrossRef CAS PubMed.
- S. Kavitha, C. Jayashree, S. A. Kumar, S. Kaliappan and J. R. Banu, Bioresour. Technol., 2014, 173, 32–41 CrossRef CAS PubMed.
- D. Zhang, Y. Chen, Y. Zhao and X. Zhu, Environ. Sci. Technol., 2010, 44, 4802–4808 CrossRef CAS PubMed.
- X. Wang, Y. Zhang, T. Zhang, J. Zhou and M. Chen, Chem. Eng. J., 2016, 283, 167–174 CrossRef CAS.
- L. Appels, J. Baeyens, J. Degrève and R. Dewil, Prog. Energy Combust. Sci., 2008, 34, 755–781 CrossRef CAS.
- G.-H. Yu, P.-J. He, L.-M. Shao and Y.-S. Zhu, Water Res., 2008, 42, 1925–1934 CrossRef CAS PubMed.
- J. Zhao, Y. Liu, B. Ni, Q. Wang, D. Wang, Q. Yang, Y. Sun, G. Zeng and X. Li, Sci. Rep., 2016, 6, 21622 CrossRef CAS PubMed.
- B. Xiao, C. Liu, J. Liu and X. Guo, Bioresour. Technol., 2015, 196, 109–115 CrossRef CAS PubMed.
- A. Zhou, W. Liu, C. Varrone, Y. Wang, A. Wang and X. Yue, Bioresour. Technol., 2015, 192, 835–840 CrossRef CAS PubMed.
- C. Yang, A. Zhou, Z. He, L. Jiang, Z. Guo, A. Wang and W. Liu, Environ. Sci. Pollut. Res. Int., 2015, 22, 9100–9109 CrossRef CAS PubMed.
- Q. Yang, K. Luo, X.-m. Li, D.-b. Wang, W. Zheng, G.-m. Zeng and J.-j. Liu, Bioresour. Technol., 2010, 101, 2924–2930 CrossRef CAS PubMed.
- N. M. G. Coelho, R. L. Droste and K. J. Kennedy, Water Res., 2011, 45, 2822–2834 CrossRef CAS PubMed.
- A. Zhou, C. Yang, F. Kong, D. Liu, Z. Chen, N. Ren and A. Wang, J. Environ. Biol., 2013, 34, 381–389 CAS.
- J. Sheng, R. Vannela and B. E. Rittmann, Environ. Sci. Technol., 2011, 45, 3795–3802 CrossRef CAS PubMed.
- H. Yuan, Y. Chen, H. Zhang, S. Jiang, Q. Zhou and G. Gu, Environ. Sci. Technol., 2006, 40, 2025–2029 CrossRef CAS PubMed.
- Y. Yan, L. Feng, C. Zhang, C. Wisniewski and Q. Zhou, Water Res., 2010, 44, 3329–3336 CrossRef CAS PubMed.
- S. Tang, Z. Wang, Z. Wu and Z. Qi, J. Hazard. Mater., 2010, 178, 377–384 CrossRef CAS PubMed.
- D. Markechova, M. Tomkova and J. Sadecka, Pol. J. Environ. Stud., 2013, 22, 1289–1295 CAS.
- A. Eaton, L. S. Clesceri, E. W. Rice, A. E. Greenberg and M. Franson, APHA: Standard Methods for the Examination of Water and Wastewater, Centennial Edition., APHA, AWWA, WEF, Washington, DC, 2005 Search PubMed.
- A. Zhou, H. Luo, C. Varrone, Y. Wang, W. Liu, A. Wang and X. Yue, Process Biochem., 2015, 50, 1413–1421 CrossRef CAS.
- P. H. Nielsen and A. Jahn, in Extraction of EPS, ed. J. Wingender, T. R. Neu and H. C. Flemming, Spinger, Berlin, 1999, pp. 49–72 Search PubMed.
- C. Park and J. T. Novak, Water Res., 2007, 41, 1679–1688 CrossRef CAS PubMed.
- A. Ramesh, D.-J. Lee and S. Hong, Appl. Microbiol. Biotechnol., 2006, 73, 219–225 CrossRef CAS PubMed.
- X. Y. Li and S. F. Yang, Water Res., 2007, 41, 1022–1030 CrossRef CAS PubMed.
- L. Lu, D. Xing, B. Liu and N. Ren, Water Res., 2011, 46, 1015–1026 CrossRef PubMed.
- T. Liu, Z. L. Chen, W. Z. Yu and S. J. You, Water Res., 2011, 45, 2111–2121 CrossRef CAS PubMed.
- G. Zhuo, Y. Yan, X. Tan, X. Dai and Q. Zhou, J. Biotechnol., 2012, 159, 27–31 CrossRef CAS PubMed.
- X. L. Liu, H. Liu, G. C. Du and J. Chen, Water Environ. Res., 2009, 81, 13–20 CrossRef CAS PubMed.
- G. A. Ekama and G. v. R. Marais, Influence of wastewater characteristic on process design. In: Theory Design and Operation of Nutrient Removal Activated Sludge Processes, Water Research Commission, Pretoria, South Africa, 1984 Search PubMed.
- Q. Wang, M. Kuninobu, H. I. Ogawa and Y. Kato, Biomass Bioenergy, 1999, 16, 407–416 CrossRef CAS.
- I. S. Turovskiy and P. Mathai, Wastewater sludge processing, John Wiley & Sons, 2006 Search PubMed.
- K. Boe and I. Angelidaki, Online monitoring and control of the biogas process, Technical University of DenmarkDanmarks Tekniske Universitet, Department of Systems BiologyInstitut for Systembiologi, 2006 Search PubMed.
- M. H. Hwang, N. J. Jang, S. H. Hyun and I. S. Kim, J. Biotechnol., 2004, 111, 297–309 CrossRef CAS PubMed.
|
This journal is © The Royal Society of Chemistry 2016 |