DOI:
10.1039/C6RA19039A
(Paper)
RSC Adv., 2016,
6, 83820-83830
Novel water-soluble phosphatriazenes: versatile ligands for Suzuki–Miyaura, Sonogashira and Heck reactions of nucleosides†
Received
27th July 2016
, Accepted 26th August 2016
First published on 29th August 2016
Abstract
Two new water-soluble phosphatriazene ligands have been synthesized as versatile ligands for complexation reactions with Pd(OAc)2 and utilized for catalyzing column-free Suzuki–Miyaura cross-coupling of various purine and pyrimidine halonucleosides in water. The water-solubility of the catalytic system simplified the isolation of the cross-coupled products to mere filtration, while the catalytically active solution in the filtrate was recycled eight times. A novel copper-free Sonogashira coupling protocol for the nucleosides has also been established via a one-pot synthesis of FV-100, a nucleoside-based drug in phase 3 clinical trials for herpes zoster or shingles treatment. Application of the Heck reaction was demonstrated by the synthesis of another antiviral drug: BVDU.
Multifunctional nucleosides, nucleotides or oligonucleotides1 have proved to be molecules of synthetic and biological importance given their applications as antivirals,2 anticancer drugs3 and as fluorescent biological probes.4 An exponential rise in the synthesis of nucleoside-based therapeutic5,6 drugs has been brought about by efficient metal-catalyzed cross-coupling7 functionalization strategies for both purines and pyrimidines. In particular, palladium-catalyzed cross-coupling8 processes have contributed immensely to the growth of this field. Amongst others, palladium-catalyzed Suzuki–Miyaura,9 Sonogashira10 and Heck11 reactions have proved to be powerful tools in the hands of synthetic chemists facilitating greener and sustainable solutions for the modification of such structural motifs.
In recent years, sustainability in coupling processes has been associated with the employment of environmentally benign reaction solvents,12 recyclability of the catalyst and column-free product isolation as crucial characteristics.13 Often, catalytic transformations of nucleosides have exclusively been performed in aprotic polar solvents without the possibility of recyclability of the catalytic system.8b Water, in this regard could and should become the solvent of choice8b,14 advancing the sought-after optimized sustainable approach in particular when combined with recyclability. There are examples in the literature reported by Shaughnessy, Hocek and others in which water is used in combination with organic solvents (most commonly MeCN) for the palladium-catalyzed Suzuki–Miyaura reaction of purine and pyrimidine nucleosides.15 Only recently, our research group presented the application of water-soluble Pd-imidate complexes of the type [trans-[Pd(imidate)2(PTA)2] (PTA = 1,3,5-triaza-7-phosphaadamantane) as catalysts for synthesis of modified nucleosides in water.16 Although good yields of the products were obtained, the respective protocol did not permit recycling of the catalyst or isolating the products in a column-free manner.
To address this gap, we report herein the discovery of two new water-soluble phosphatriazene ligands (PTAPS 1a and PTABS 1b), which in combination with Pd(OAc)2 produce a powerful catalyst for the Suzuki–Miyaura cross-coupling of all four halonucleosides in water as the reaction solvent. Greater water-solubility of the resultant catalytic system allows column-free17 isolation of the cross-coupled products and ability to recycle the catalyst. Copper-free Sonogashira cross-coupling of nucleosides and the application of Heck alkenylation for the synthesis of fluorescent nucleosides further demonstrates the potential of the technology as unique and environmentally attractive for drug discovery.
The novel water-soluble ligands, PTAPS (1a) and PTABS (1b) (where PS is propane sultonate and BS is butane sultonate) were synthesized by simply stirring PTA with the respective sultones in acetone under reflux conditions (Scheme 1). Besides confirming the structures by general characterization techniques (NMR, IR, EA), single crystal X-ray analysis was performed on 1a revealing the expected structure with three water molecules co-crystallised in the crystal lattice (see ESI for more details†). The PTAPS (1a) and water molecules created an extensive H-bonding three dimensional network throughout the lattice, emphasizing the excellent water solubility of the ligand. Based on this data we believe that although, 1b did not yield crystals suitable for X-ray characterization, both reagents are expected to have similar H-bonding network.
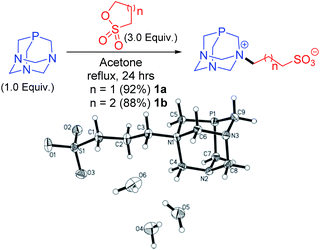 |
| Scheme 1 (a) Synthesis of water-soluble PTABS and PTAPS ligands. (b) Single crystal X-ray for PTAPS (CCDC 1489309). Ellipsoids are shown with 50% probability. | |
To investigate the applications of the two new ligands for cross-coupling processes, we initially examined these in the palladium-catalyzed Suzuki–Miyaura cross-coupling of halonucleosides in water. Preliminary observations revealed the formation of a homogeneous solution in course of the reaction between Pd(OAc)2 and ligands. This is a crucial observation as it allows a column-free isolation of the products and renders the catalytic system recyclable. Subsequently, screening studies were undertaken, (Table 1) identifying the combination of Pd(OAc)2/1b as the most efficient in catalyzing the coupling reaction between 5-iodo-2′-deoxyuridine and benzofuran-2-boronic acid at 1.0 mol% Pd(OAc)2 and 2.0 mol% 1b catalyst loading.
Table 1 Screening studies for Suzuki–Miyaura coupling of nucleosides in watera
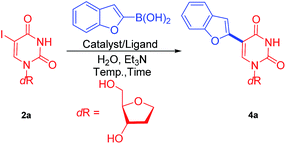
|
No. |
Catalyst |
Ligand |
Temp °C |
Time (h) |
Yieldb% |
Reaction conditions: 0.5 mmol of 5-IdU, 0.75 mmol of 2-benzofuranyl boronic acid, 1.0 mol% of catalyst, 2.0 mol% of ligand, 1.0 mmol of triethylamine, 3 ml of solvent (H2O). Isolated yields by filtration method. Isolated yields by column chromatography. 1.0 mol% of catalyst and 1.0 mol% of ligand. 0.5 mol% of catalyst and 1.0 mol% of ligand. 0.1 mol% of catalyst and 0.2 mol% of ligand. |
1 |
Pd(OAc)2 |
— |
80 |
6 |
35c |
2 |
Pd(OAc)2 |
PTA |
80 |
6 |
52c |
3 |
Pd(OAc)2 |
TPPTS |
80 |
6 |
69c |
4 |
Pd(OAc)2 |
PTAPS |
80 |
3 |
82c |
5 |
Pd(OAc)2 |
PTABS |
80 |
3 |
88c |
6 |
Pd(OAc)2 |
PTABS |
60 |
3 |
78c |
7 |
Pd(OAc)2 |
PTABS |
R.T. |
6 |
70c |
8d |
Pd(OAc)2 |
PTABS |
80 |
3 |
75 |
9e |
Pd(OAc)2 |
PTABS |
80 |
6 |
65 |
10f |
Pd(OAc)2 |
PTABS |
80 |
12 |
60 |
Notably, a column-free isolation protocol for the product could be established. Equally important is the fact that the discovered catalyst system is an order of magnitude more efficient than our 1st generation [trans-[Pd(imidate)2(PTA)2]16 complexes reflected by reducing the reaction time to half. This is most probably due to the latter's limited water-solubility, which also prevents a direct isolation of the products (column chromatographic purification was carried out).16
Next, an extensive investigation of various halonucleoside/aryl boronic acid combinations was undertaken (Scheme 2). The Pd/1b catalyst was found to be efficient in coupling all four unprotected halonucleosides namely, 2′-deoxy-uridine, -cytidine, -adenosine and -guanosine with a variety of arylboronic acids in good to excellent yields. For comparison purpose yields for column-free isolation (filtration of solids) as well as after column chromatography are summarized in Scheme 2. The yields for the cross-coupled products using 1st generation [trans-[Pd(saccharinate)2(PTA)2] complex16b is also shown where products were isolated after chromatography and it required extended reaction times these results clearly support the higher efficiency of the 2nd generation Pd/1b catalytic system available for the modification of nucleosides today. The sustainability of this catalytic system was tested next by recycling an important consideration for a green coupling process (Scheme 3).
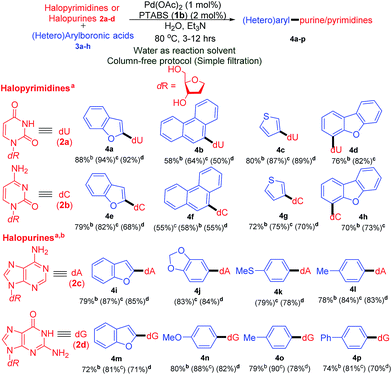 |
| Scheme 2 Palladium-catalyzed column-free Suzuki–Miyaura coupling of halonucleosides in neat water. aArylboronic acid (0.75 mmol), halonucleoside (0.5 mmol), Pd(OAc)2 (1.0 mol%), PTABS 1b (2.0 mol%), 3 ml H2O, Et3N (1.0 mmol) at 80 °C for 3–12 h. bIsolated yields by filtration. cIsolated yields after column chromatography. dIsolated yields for the reaction carried out by replacing Pd(OAc)2/1b with 1 mol% [Pd(Sacc)2(PTA)2] for 6 h with product isolated by column chromatography. | |
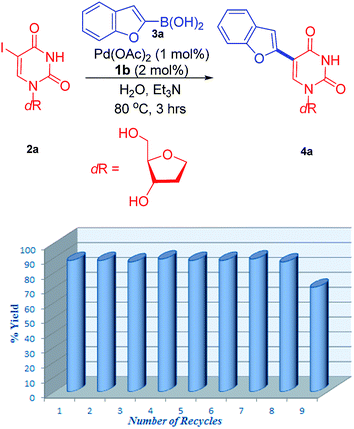 |
| Scheme 3 Recyclability studies for column-free Suzuki–Miyaura cross-coupling using water-soluble PTABS (1b) ligand with Pd(OAc)2. | |
The enhanced solubility exhibited by the Pd/1b catalytic system permits convenient recycling18 of the catalytically active metal that remains in the aqueous solution while the precipitated product can be simply isolated via filtration. The exact nature of the catalytic complex present in solution is still uncertain, however, and the possibility of a homotopic catalyst or stabilized nanoparticles cannot be ruled out. The column-free protocol was applied to the reaction of 5-iodo-2′-deoxyuridine and benzofuran-2-boronic acid at 1.0 mol% Pd(OAc)2 and 2.0 mol% 1b, which at the end of the reaction was simply filtered to furnish high purity cross-coupled product (confirmed by 1H NMR and HPLC). The filtrate containing the catalytic system was found to be fully active for seven more consecutive cycles. In the ninth catalytic application a slight drop in the yield was observed. This protocol constitutes the first reported example of recyclability achieved for the palladium-catalyzed Suzuki–Miyaura cross-coupling of halonucleosides with arylboronic acids in water.19 To check the amount of catalyst leaching/metal contamination during synthesis of biologically active nucleosides, the cross-coupled products were analysed by Inductively-Coupled-Plasma Mass Spectrometry (ICP-MS) after the 1st and the 8th cycle. The results revealed negligible amounts (ppb levels) of palladium in these samples.
Encouraged by the positive Suzuki–Miyaura coupling results, we decided to explore the potential of the Pd/1b catalytic system for the copper-free Sonogashira coupling of halonucleosides with alkynes in water. In recent years the palladium-catalyzed Sonogashira11 coupling reaction has proven to be a powerful synthetic tool for installation of alkyne groups. This protocol has led to the synthesis of fluorescent nucleosides with applications as biological probes.20 Despite its importance, the Sonogashira reaction suffers from a major limitation. It requires the use of stoichiometric amounts of copper co-catalyst, which might also contaminate the modified nucleosides. Copper-free Sonogashira21 couplings have been reported in the literature for general substrates. However, only one report22 has highlighted its potential for the alkynylation of 5-iodo-2′-deoxyuridine in the mixed H2O/CH3CN solvent system, emphasizing the need for an improved and more general protocol for the copper-free Sonogashira coupling of halonucleosides. Here, we first investigated the utility of the phosphatriazene ligands with Pd(OAc)2 for coupling reactions with 5-iodo-2′-deoxycytidine.
Screening studies performed on the substrate in H2O/CH3CN revealed an outstanding activity of the new Pd/1b catalytic system (Table 2). Notably, the pronounced acceleration of the catalytic transformation by PTABS/Pd allowed the coupling to take place in just 45 minutes as compared to several hours in the case of PTA/Pd and Pd/1a systems. However, for this reaction type the isolation was done by subjecting the crude product to column chromatographic purification (column-free was not feasible due to lower purity attainment).
Table 2 Screening studies for Sonogashira coupling of 5-iodo-2-deoxycytidine with phenyl acetylenea
Given the reactivity of the catalyst system, we investigated the scope of the copper-free Sonogashira coupling reactions. Differently substituted alkynes when cross-coupled provided good yields of the alkynated product without much influence of any electronic effects (Scheme 4).
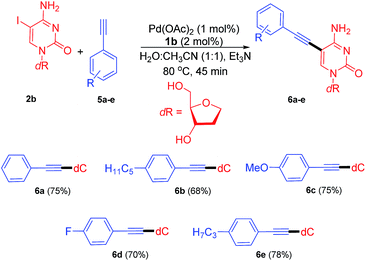 |
| Scheme 4 Sonogashira coupling of 5-iodo-2-deoxycytidine with alkynes. aReaction conditions: 0.5 mmol of 5-IdC, 1.0 mmol of alkyne, 1.0 mol% of Pd(OAc)2, 2.0 mol% of 1b, 1.0 mmol of triethylamine, 3 ml of H2O : ACN (1 : 1), bisolated yields. | |
Next, we utilized the Sonogashira coupling for synthesis of an antiviral nucleoside FV-100 starting with 5-iodo-2′-deoxyuridine.23 In literature, the synthesis of FV-100 via similar procedure yielded only 54% of the desired product with large amounts of copper co-catalyst added.23d,e Our initial attempts for the cross-coupling resulted in the formation of two inseparable products (see ESI for details†). These products were characterized as desired FV-100 and the intermediate (alkynated product). To drive this reaction to completion, we envisaged a sequential one-pot Sonogashira/cyclization24 protocol. Therefore, the first step involves the Sonogashira coupling of the substituted phenylacetylene with 5-iodo-2′-deoxyuridine, which, upon completion, is followed by a copper iodide mediated cyclization reaction. Our protocol allowed the synthesis of FV-100 and other molecules of similar structural features in high yields, which are a magnitude better than those from general routes reported in the literature (characterization data compared to literature for FV-100 was found to be identical for the isolated product).25
The Heck alkenylation reaction10,26 is yet another darling of cross-coupling reactions utilized by both academia and industry alike. Its applications range from simple to complex to organic molecules of biological relevance. Given the foregoing success with the Pd/1b catalytic system, we decided to explore the possibility of assembling fluorescent nucleosides via one-pot sequential Heck/Suzuki–Miyaura27 coupling reactions (Scheme 5). The experimental details are summarized in ESI.† The unique feature of the protocol comprise the initial selective C–I bond activation (in the presence of C–Br on the styrene synthon) using new Pd/1b system, followed by a Suzuki–Miyaura coupling in the presence of S-Phos/Pd catalytic system in H2O/CH3CN mixture. The resultant nucleosides were found to exhibit very good fluorescence properties due to extended conjugation. Therefore, these nucleosides may serve as building-blocks for construction of DNA probes.
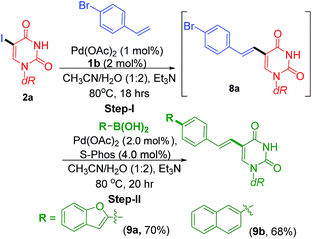 |
| Scheme 5 One-pot sequential Heck/Suzuki–Miyaura coupling. | |
Finally, to further demonstrate the potential of the Pd/1b system, we investigated the synthesis of a commercially important antiviral drug Brivudine (BVDU).28 Recently, we reported on the use of 1st generation [trans-[Pd(saccharinate)2(PTA)2] complex for Heck reaction in CH3CN.19 Taking advantage of the enhanced water solubility exhibited by the Pd/1b system, the catalytic reaction between 5-iodo-2′-deoxyuridine and methyl acrylate was now possible in a H2O/CH3CN mixture furnishing the coupled product in 87% yield (Scheme 6). Hydrolysis followed by NBS-mediated bromination, allowed the synthesis of BVDU in an overall yield of 72% over three synthetic steps with identical characterization data to that reported in literature was obtained for the product. Two key achievements of the novel protocol are the reduction in the amount of CH3CN used and the lowered metal content in the final product because the catalytic system exhibits such good water solubility.
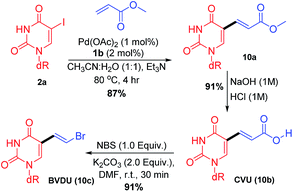 |
| Scheme 6 Efficient synthesis of anti-viral drug BVDU. | |
In conclusion, we have synthesized and characterized novel water-soluble phosphatriazine ligands (1a and 1b) and utilized them together with Pd(OAc)2 as catalytic systems for cross-coupling reactions. The potential utility of new water soluble catalytic system was clearly demonstrated by several C–C bond forming reactions. First, we established the Suzuki–Miyaura cross-coupling of halonucleosides in water exploiting the recycling of the catalytic system and column-free isolation of the coupled products. Second, implementation of copper-free Sonogashira coupling in aqueous media was a crucial step towards the development of an efficient one-pot sequential Sonogashira/cyclization methodology leading to the synthesis of an antiviral drug FV-100. Third, the Heck/Suzuki–Miyaura one-pot sequential synthesis of fluorescent nucleosides and the efficient synthesis of another antiviral drug BVDU were also achieved in high yields. Clearly, these results support and validate the value of the new Pd/1b catalyst system for the assembly of wide range of molecules in a simple, efficient and economical way. Use of this catalyst may hold unprecedented versatility for the metal-mediated modification of a variety of modified nucleosides and a step closer towards greener chemistry and improved sustainability.
Experimental section
All reactions were carried out under nitrogen atmosphere in Schlenk tubes using Schlenk system with magnetic stirring. Unless otherwise stated, all reagents were purchased from commercial suppliers and used without further purification. All solvents used were distilled from appropriate drying agents prior to use. All solvents employed in reactions were purged with nitrogen at least for 15 minutes prior to use. UV absorptions study was performed locally. Reactions were monitored by thin layer chromatography using TLC silica gel 60 F254 precoated plates (Merck). Visualization was accomplished by irradiation with UV light. C, H, and N analyses were carried out locally. NMR data (1H, 13C and 31P) were recorded locally 500 MHz spectrometers. Mass spectral analysis was carried out locally.
Synthesis of PTAPS (1a) & PTABS (1b)
Procedure. To a clean and dry Schlenk tube, which is evacuated and flushed with nitrogen, added TPA (0.786 mg, 5.0 mmol) and acetone (25 ml), stirred the solution at ambient temperature till solution becomes practically colorless. Then, added sultone (1,3-propane sultone (1.32 ml, 15 mmol) for 1a and 1,4-butane sultone (1.53 ml, 15 mmol) for 1b) drop wise and allow refluxing for 24 h. Then reaction mixture cooled to R.T. and precipitated solid was separated by filtration u/N2 atmosphere. The product was washed several times with acetone and dried well under vacuum to obtain a white solid in 92% (1.28 g) for 1a and 88% (1.29 g) for 1b.
PTAPS (1a). IR 3410, 2999, 2956, 1675, 1205, 1193, 1170, 1039, 1026, 809, 581, 559, 535, 521 cm−1.1H NMR (500 MHz, D2O) δ 5.07 (d, J = 11.4 Hz, 2H), 4.87 (d, J = 11.7 Hz, 2H), 4.65 (d, J = 13.8 Hz, 1H), 4.49 (d, J = 13.8 Hz, 1H), 4.41 (d, J = 6.0 Hz, 2H), 4.00 (t, J = 14.0 Hz, 2H), 3.90 (dd, J = 14.5, 8.8 Hz, 2H), 3.17–3.11 (m, 2H), 2.99 (t, J = 7.2 Hz, 2H), 2.28–2.20 (m, 2H). 13C NMR (126 MHz, D2O) δ 79.1, 69.4, 61.1, 53.0, 52.8, 47.4, 45.8, 45.6, 15.6. 31P NMR (151 MHz, D2O) δ −83.87. Anal. calcd for C9H18N3O3PS: C, 38.70; H, 6.50; N, 15.05; S, 11.48. Found: C, 38.62; H, 6.41; N, 14.97; S, 11.42.
PTABS (1b). IR 3419, 3003, 2960, 1675, 1451, 1210, 1171, 1044, 1024, 810, 612, 582, 559, 528, 522 cm−1. 1H NMR (500 MHz, D2O) δ 4.97 (d, J = 11.1 Hz, 2H), 4.79 (d, J = 11.7 Hz, 2H), 4.59 (d, J = 13.8 Hz, 1H), 4.43 (d, J = 13.7 Hz, 1H), 4.32 (d, J = 6.2 Hz, 2H), 4.02–3.77 (m, 4H), 2.95 (dd, J = 15.5, 7.5 Hz, 4H), 1.97–1.84 (m, 2H), 1.82–1.70 (m, 2H). 13C NMR (125 MHz, D2O) 78.7, 69.3, 62.1, 53.0, 52.6, 49.7, 45.7, 45.4, 21.1, 18.0. 31P NMR (151 MHz, D2O) δ −83.79. Anal. calcd for C10H20N3O3PS: C, 40.95; H, 6.87; N, 14.33; S, 10.93. Found: C, 40.87; H, 6.79; N, 14.28; S, 10.84.
Representative procedure. To a solution of palladium acetate (1.12 mg, 1.0 mol%) and PTABS ligand (2.93 mg, 2.0 mol%) in degassed water (1.0 ml) at ambient temperature under N2 added halo nucleoside (0.5 mmol) and the solution stirred for 5 min at 80 °C. After that reaction mixture allowed to cool at room temperature and then added aryl boronic acid (0.75 mmol) along with triethylamine (0.14 ml, 1 mmol) and degassed water (2.0 ml). The resulting solution was then stirred at 80 °C for 3–12 hours. The reaction progress was observed by TLC analysis, after the completion of the reaction, filtered the precipitated solid on Buchner funnel and washed with water (2 ml × 3 times) followed by ethyl acetate (2 ml × 2 times) and diethyl ether (2 ml) to afford the desired product as a solid.
5-(2-Benzofuranyl)-2′-deoxyuridine (4a)16a. Representative procedure was followed by using 5-IdU (177 mg, 0.5 mmol) and 2-benzofuranyl boronic acid (122 mg, 0.75 mmol), yielded 4a (151 mg, 88% by filtration while 161 mg, 94% by column chromatography) in 3 h as off-white solid. UV absorption in methanol (125 μM) λmax = 246, 323 nm. 1H NMR (500 MHz, DMSO-d6) δ 11.72 (s, 1H), 8.70 (s, 1H), 7.58 (d, J = 7.4 Hz, 1H), 7.50 (d, J = 8.1 Hz, 1H), 7.30 (s, 1H), 7.24 (t, J = 7.5 Hz, 1H), 7.17 (t, J = 7.4 Hz, 1H), 6.19 (t, J = 6.3 Hz, 1H), 5.28 (d, J = 4.2 Hz, 1H), 5.20 (t, J = 4.1 Hz, 1H), 4.30 (s, 1H), 3.84 (d, J = 2.8 Hz, 1H), 3.74–3.59 (m, 2H), 2.25–2.15 (m, 2H). 13C NMR (125 MHz, DMSO-d6) δ 160.7, 153.4, 149.7, 149.4, 137.5, 129.1, 124.7, 123.4, 121.4, 111.2, 105.1, 104.3, 87.9, 85.5, 70.4, 61.2, 40.8. ESI-MS (m/z) = 345 (M+ + H+).
5-(Phenanthren-9-yl)-2′-deoxyuridine (4b)16a. Representative procedure was followed by using 5-IdU (177 mg, 0.5 mmol) and 9-phenanthrenyl boronic acid (167 mg, 0.75 mmol), yielded 4b (117 mg, 58% by filtration while 129 mg, 64% by column chromatography) in 6 h as white solid. UV absorption in methanol (125 μM) λmax = 254 nm. 1H NMR (500 MHz, DMSO-d6) δ 11.57 (s, 1H), 8.83 (t, J = 8.2 Hz, 2H), 8.11 (s, 1H), 7.96 (d, J = 7.6 Hz, 1H), 7.82–7.52 (m, 6H), 6.27 (t, J = 6.6 Hz, 1H), 5.23 (d, J = 4.0 Hz, 1H), 4.82 (t, J = 4.6 Hz, 1H), 4.21 (s, 1H), 3.76 (d, J = 3.1 Hz, 1H), 3.52–3.39 (m, 2H), 2.26–2.10 (m, 2H). 13C NMR (125 MHz, DMSO-d6) δ 163.0, 150.9, 139.7, 131.4, 130.7, 130.2, 130.1, 129.1, 129.0, 127.6, 127.3, 127.1, 127.1, 127.1, 123.4, 123.2, 114.2, 87.8, 84.8, 70.7, 61.3, 40.4. ESI-MS (m/z) = 405 (M+ + H+). Anal. calcd for C23H20N2O5: C, 68.31; H, 4.98; N, 6.93. Found: C, 68.18; H, 4.89; N, 6.82.
5-(Thiophen-3-yl)-2′-deoxyuridine (4c)16a. Representative procedure was followed by using 5-IdU (177 mg, 0.5 mmol) and 3-thienyl boronic acid (192 mg, 1.5 mmol), yielded 4c (124 mg, 80% by filtration while 135 mg, 87% by column chromatography) in 3 h as off-white solid. UV absorption in methanol (125 μM) λmax = 296, 277 nm. 1H NMR (500 MHz, DMSO-d6) δ 11.54 (s, 1H), 8.47 (s, 1H), 7.97 (s, 1H), 7.58–7.39 (m, 2H), 6.24 (t, J = 6.0 Hz, 1H), 5.35–5.27 (m, 2H), 4.32 (s, 1H), 3.84 (s, 1H), 3.66 (d, J = 8.5 Hz, 2H), 2.23 (ddd, J = 15.6, 10.7, 5.2 Hz, 2H). 13C NMR (125 MHz, DMSO-d6) δ 162.1, 149.7, 137.1, 133.2, 126.0, 125.7, 121.8, 109.2, 87.6, 84.8, 70.1, 60.9, 45.4. ESI-MS (m/z) = 311 (M+ + H+).
5-(Dibenzofuran-4-yl)-2′-deoxyuridine (4d). Representative procedure was followed by using 5-IdU (177 mg, 0.5 mmol) and 4-(dibenzofuranyl)boronic acid (159 mg, 0.75 mmol), yielded 4d (150 mg, 76% by filtration while 162 mg, 82% by column chromatography) in 3 h as white solid. UV absorption in methanol (125 μM) λmax = 286 nm. 1H NMR (500 MHz, DMSO-d6) δ 11.62 (s, 1H), 8.41 (s, 1H), 8.12 (d, J = 7.4 Hz, 1H), 8.06 (d, J = 7.7 Hz, 1H), 7.67 (t, J = 7.8 Hz, 2H), 7.49 (t, J = 7.7 Hz, 1H), 7.38 (t, J = 7.6 Hz, 2H), 6.28 (t, J = 6.7 Hz, 1H), 5.29 (d, J = 3.8 Hz, 1H), 4.87 (t, J = 4.9 Hz, 1H), 4.27 (s, 1H), 3.81 (d, J = 2.9 Hz, 1H), 3.54 (s, 2H), 2.29–2.14 (m, 2H). 13C NMR (125 MHz, DMSO-d6) δ 161.7, 155.3, 152.9, 150.0, 140.1, 128.3, 127.6, 123.8, 123.6, 123.2, 122.8, 121.1, 120.3, 117.8, 111.7, 108.8, 87.6, 84.5, 70.5, 61.4, 39.9. ESI-MS (m/z) = 395 (M+ + H+). Anal. calcd for C21H18N2O6: C, 63.96; H, 4.60; N, 7.10. Found: C, 63.85; H, 4.64; N, 6.98.
5-(2-Benzofuranyl)-2′-deoxycytidine (4e)16a. Representative procedure was followed by using 5-IdC (177 mg, 0.5 mmol) and 2-benzofuranyl boronic acid (162 mg, 1 mmol), yielded 4e (136 mg, 79% by filtration while 141 mg, 82% by column chromatography) in 3 h as white solid. UV absorption in methanol (125 μM) λmax = 322, 271, 261 nm. 1H NMR (500 MHz, DMSO-d6) δ 8.50 (s, 1H), 7.71 (s, 1H), 7.56 (t, J = 7.1 Hz, 2H), 7.24 (dtd, J = 18.5, 7.3, 1.2 Hz, 2H), 6.99 (s, 1H), 6.93 (s, 1H), 6.15 (t, J = 6.3 Hz, 1H), 5.22 (d, J = 4.2 Hz, 1H), 5.12 (t, J = 4.7 Hz, 1H), 4.23 (s, 1H), 3.80 (d, J = 3.4 Hz, 1H), 3.69–3.53 (m, 2H), 2.26–2.02 (m, 2H). 13C NMR (125 MHz, DMSO-d6) δ 162.0, 154.5, 153.6, 150.5, 142.6, 129.0, 124.8, 123.7, 121.3, 111.9, 103.7, 98.1, 88.1, 86.2, 70.2, 61.1, 45.9. ESI-MS (m/z) = 344 (M+ + H+). Anal. calcd for C17H17N3O5: C, 59.47; H, 4.99; N, 12.24. Found: C, 59.41; H, 5.05; N, 12.34.
5-(Phenanthren-9-yl)-2′-deoxycytidine (4f)16a. Representative procedure was followed by using 5-IdC (177 mg, 0.5 mmol) and 9-phenanthrenyl boronic acid (222 mg, 1 mmol), yielded 4f (111 mg, 55% by filtration while 117 mg, 58% by column chromatography) in 6 h as off-white solid. UV absorption in methanol (125 μM) λmax = 298, 254 nm. 1H NMR (500 MHz, DMSO-d6): δ 8.85 (dd, J = 14.4, 8.2 Hz, 2H), 7.99 (t, J = 7.4 Hz, 1H), 7.92 (s, 1H), 7.76 (d, J = 4.7 Hz, 1H), 7.73–7.51 (m, 5H), 7.25 (s, 1H), 6.24 (dt, J = 10.1, 6.8 Hz, 1H), 6.14 (s, 1H), 5.18 (dd, J = 9.7, 4.2 Hz, 1H), 4.75 (dt, J = 14.0, 5.0 Hz, 1H), 4.20–4.09 (m, 1H), 3.76–3.68 (m, 1H), 3.48–3.37 (m, 2H), 2.19–1.98 (m, 2H). 13C NMR (125 MHz, DMSO-d6): δ 164.6, 155.3, 141.1, 131.7, 131.6, 131.4, 130.8, 130.6, 130.6, 130.1, 129.2, 129.2, 127.6, 127.4, 127.3, 127.2, 126.2, 106.3, 87.6, 85.4, 70.7, 61.4, 52.4. ESI-MS (m/z) = 392 (M+ + H+). Anal. calcd for C23H21N3O4: C, 68.47; H, 5.25; N, 10.42. Found: C, 68.33; H, 5.17; N, 10.31.
5-(Thiophen-3-yl)-2′-deoxycytidine (4g)29a. Representative procedure was followed by using 5-IdC (177 mg, 0.5 mmol) and 3-thienylboronic acid (192 mg, 1.5 mmol), yielded 4g (111 mg, 72% by filtration while 116 mg, 75% by column chromatography) in 3 h as off-white solid. UV absorption in methanol (125 μM) λmax = 297 nm. 1H NMR (500 MHz, DMSO-d6) δ 7.98 (s, 1H), 7.62 (dd, J = 29.1, 24.8 Hz, 3H), 7.16 (d, J = 4.7 Hz, 1H), 6.47 (s, 1H), 6.20 (t, J = 6.4 Hz, 1H), 5.23 (d, J = 3.6 Hz, 1H), 5.05 (s, 1H), 4.23 (s, 1H), 3.79 (d, J = 2.9 Hz, 1H), 3.57 (dd, J = 24.1, 11.5 Hz, 2H), 2.20–2.03 (m, 2H). 13C NMR (125 MHz, DMSO-d6) δ 163.3, 154.2, 140.1, 133.8, 128.0, 127.1, 123.3, 103.0, 87.3, 85.2, 70.1, 61.0, 45.4. ESI-MS (m/z) = 310 (M+ + H+).
5-(Dibenzofuran-4-yl)-2′-deoxycytidine (4h)16a. Representative procedure was followed by using 5-IdC (177 mg, 0.5 mmol) and 4-(dibenzofuranyl)boronic acid (212 mg, 1 mmol), yielded 4h (138 mg, 70% by filtration while 143 mg, 73% by column chromatography) in 6 h as white solid. UV absorption in methanol (125 μM) λmax = 243, 285 nm. 1H NMR (500 MHz, DMSO-d6) δ 8.13 (t, J = 7.1 Hz, 1H), 7.98 (s, 1H), 7.67 (d, J = 8.2 Hz, 1H), 7.48 (t, J = 7.7 Hz, 1H), 7.44–7.33 (m, 2H), 6.46 (s, 1H), 6.22 (t, J = 6.6 Hz, 1H), 5.19 (d, J = 4.0 Hz, 1H), 4.83 (t, J = 4.8 Hz, 1H), 4.18 (s, 1H), 3.74 (d, J = 3.1 Hz, 1H), 3.51–3.40 (m, 1H), 2.18–2.02 (m, 1H). 13C NMR (125 MHz, DMSO-d6) δ 163.6, 155.8, 155.0, 154.0, 141.7, 129.1, 128.0, 124.6, 124.2, 123.8, 123.5, 121.5, 121.3, 118.4, 112.2, 103.0, 87.7, 85.5, 70.7, 61.5, 40.9. ESI-MS (m/z) = 394 (M+ + H+). Anal. calcd for C21H19N3O5: C, 64.12; H, 4.87; N, 10.68. Found: C, 64.01; H, 4.79; N, 10.58.
8-(2-Benzofuranyl)-2′-deoxyadenosine (4i)16a. Representative procedure was followed by using 8-BrdA (165 mg, 0.5 mmol) and 2-benzofuranyl boronic acid (161 mg, 1 mmol), yielded 4i (145 mg, 79% by filtration while 160 mg, 87% by column chromatography) in 6 h as off-white solid. UV absorption in methanol (125 μM) λmax = 324, 255 nm. 1H NMR (500 MHz, DMSO-d6) δ 8.19 (s, 1H), 7.89–7.33 (m, 7H), 6.63 (t, J = 6.8 Hz, 1H), 5.37 (t, J = 22.3 Hz, 2H), 4.56 (s, 1H), 4.01–3.88 (m, 1H), 3.78–3.48 (m, 2H), 2.34–2.23 (m, 1H). 13C NMR (125 MHz, DMSO-d6) δ 156.2, 154.3, 152.6, 149.8, 144.9, 140.7, 127.2, 126.1, 123.6, 122.0, 119.4, 111.3, 109.4, 88.1, 85.3, 71.0, 61.9, 45.1. ESI-MS (m/z) = 368 (M+ + H+). Anal. calcd for C18H17N5O4: C, 58.85; H, 4.66; N, 19.06. Found: C, 58.73; H, 4.71; N, 18.96.
8-(3,4-(Methylenedioxy)phenyl)-2′-deoxyadenosine (4j)16a. Representative procedure was followed by using 8-BrdA (165 mg, 0.5 mmol) and 3,4-(methylenedioxy)phenyl boronic acid (166 mg, 1 mmol), yielded 4j (154 mg, 83% by column chromatography) in 12 h as off-white solid. UV absorption in methanol (125 μM) λmax = 298 nm. 1H NMR (500 MHz, DMSO-d6): δ 8.13 (s, 1H), 7.52–6.99 (m, 5H), 6.15 (s, 3H), 5.54 (s, 1H), 5.26 (s, 1H), 4.46 (s, 1H), 3.87 (s, 1H), 3.73–3.45 (m, 2H), 2.15 (s, 2H). 13C NMR (125 MHz, DMSO-d6): δ 156.6, 152.4, 150.8, 150.5, 149.4, 148.1, 124.5, 123.7, 119.6, 110.0, 109.2, 102.3, 88.9, 86.2, 72.0, 62.8, 45.9. ESI-MS (m/z) = 372 (M+ + H+). Anal. calcd for C17H17N5O5: C, 54.98; H, 4.61; N, 18.86. Found: C, 54.88; H, 4.54; N, 18.77.
8-(4-(Methylthio)phenyl)-2′-deoxyadenosine (4k)16a. Representative procedure was followed by using 8-BrdA (165 mg, 0.5 mmol) and 4-(methylthio)phenyl boronic acid (168 mg, 1 mmol), yielded 4k (147 mg, 79% by column chromatography) in 12 h as off white solid. UV absorption in methanol (125 μM) λmax = 280 nm. 1H NMR (500 MHz, DMSO-d6): δ 8.13 (s, 1H), 7.59 (d, J = 7.9 Hz, 2H), 7.40 (t, J = 12.1 Hz, 4H), 6.13 (dd, J = 8.3, 6.4 Hz, 1H), 5.60 (dd, J = 8.4, 3.7 Hz, 1H), 5.24 (d, J = 3.9 Hz, 1H), 4.45 (s, 1H), 3.87 (s, 1H), 3.72–3.48 (m, 2H), 2.40 (s, 3H), 2.13 (dd, J = 12.7, 6.1 Hz, 1H). 13C NMR (125 MHz, DMSO-d6): δ 156.5, 152.2, 151.0, 150.2, 140.3, 129.7, 127.1, 119.5, 88.8, 86.1, 71.9, 62.7, 37.6, 21.4. ESI-MS (m/z) = 374 (M+ + H+). Anal. calcd for C17H19N5O3S: C, 54.68; H, 5.13; N, 18.75. Found: C, 54.55; H, 5.02; N, 18.62.
8-(p-Tolyl)-2′-deoxyadenosine (4l)16a. Representative procedure was followed by using 8-BrdA (165 mg, 0.5 mmol) and p-tolylboronic acid (136 mg, 1 mmol), yielded 4l (133 mg, 78% by filtration while 143 mg, 84% by column chromatography) in 12 h as white solid. UV absorption in methanol (125 μM) λmax = 301, 227 nm. 1H NMR (500 MHz, DMSO-d6): δ 8.15 (s, 1H), 7.66 (d, J = 8.1 Hz, 2H), 7.47 (d, J = 8.2 Hz, 4H), 6.15 (t, J = 7.3 Hz, 1H), 5.56 (s, 1H), 5.30 (d, J = 18.0 Hz, 1H), 4.47 (s, 1H), 3.88 (s, 1H), 3.74–3.49 (m, 2H), 2.56 (s, 3H), 2.14 (dd, J = 12.2, 5.7 Hz, 1H). 13C NMR (125 MHz, DMSO-d6): δ 155.9, 151.7, 150.1, 149.7, 141.1, 129.6, 125.4, 125.2, 119.0, 88.1, 85.4, 71.2, 62.0, 45.0, 36.8. ESI-MS (m/z) = 374 (M+ + H+). Anal. calcd for C17H19N5O3: C, 59.81; H, 5.61; N, 20.52. Found: C, 59.68; H, 5.46; N, 20.44.
8-(2-Benzofuranyl)-2′-deoxyguanosine (4m)29b. Representative procedure was followed by using 8-BrdG (173 mg, 0.5 mmol) and 2-benzofuranyl boronic acid (161 mg, 1 mmol), yielded 4m (138 mg, 72% by filtration) in 12 h as off-white solid. UV absorption in methanol (125 μM) λmax = 320 nm. 1H NMR (500 MHz, DMSO-d6) δ 10.97 (s, 1H), 7.74 (d, J = 7.6 Hz, 1H), 7.66 (dd, J = 8.3, 0.6 Hz, 1H), 7.45–7.37 (m, 2H), 7.34–7.28 (m, 1H), 6.60 (s, 2H), 6.46 (t, J = 7.2 Hz, 1H), 5.21 (d, J = 3.2 Hz, 1H), 4.99–4.91 (m, 1H), 4.46–4.36 (m, 1H), 3.81 (dd, J = 8.7, 5.1 Hz, 1H), 3.68–3.60 (m, 1H), 3.50 (dt, J = 11.0, 5.4 Hz, 1H), 2.13 (ddd, J = 13.0, 6.8, 3.1 Hz, 1H). 13C NMR (125 MHz, DMSO-d6) δ 157.0, 154.6, 153.9, 152.4, 146.3, 137.8, 128.0, 126.1, 124.1, 122.3, 118.2, 111.8, 108.4, 88.3, 84.8, 71.4, 62.4, 37.6. ESI-MS (m/z) = 384 (M+ + H+).
8-(4-Methoxyphenyl)-2′-deoxyguanosine (4n)15b. Representative procedure was followed by using 8-BrdG (173 mg, 0.5 mmol) and 4-methoxyphenylboronic acid (152 mg, 1 mmol), yielded 4n (149 mg, 80% by filtration) in 12 h as off-white solid. UV absorption in methanol (125 μM) λmax = 282 nm. 1H NMR (500 MHz, DMSO-d6) δ 10.74 (s, 1H), 7.58 (d, J = 8.55 Hz, 2H), 7.09 (d, J = 9.16 Hz, 2H), 6.38 (br. s., 2H), 6.04 (t, J = 7.32 Hz, 1H), 5.15 (d, J = 4.27 Hz, 1H), 4.99 (t, J = 6.1 Hz, 1H), 4.32–4.36 (m, 1H), 3.83 (s, 3H), 3.76–3.79 (m, 1H), 3.59–3.69 (m, 1H), 3.53 (dt, J = 12.06, 5.88 Hz, 1H), 1.99 (ddd, J = 13.12, 6.41, 2.44 Hz, 1H). 13C NMR (125 MHz, DMSO-d6) δ 160.3, 158.8, 153.0, 152.0, 147.3, 130.7, 122.7, 117.0, 114.2, 100.2, 87.9, 84.7, 71.2, 62.1, 55.3, 36.4. ESI-MS (m/z) = 374 (M+ + H+).
8-(p-Tolyl)-2′-deoxyguanosine (4o)29c. Representative procedure was followed by using 8-BrdG (173 mg, 0.5 mmol) and p-tolylboronic acid (136 mg, 1 mmol), yielded 4o (141 mg, 79% by filtration) in 12 h as off-white solid. UV absorption in methanol (125 μM) λmax = 298 nm. 1H NMR (500 MHz, DMSO-d6) δ 8.13 (s, 1H), 7.59 (d, J = 7.9 Hz, 2H), 7.40 (t, J = 12.1 Hz, 4H), 6.13 (dd, J = 8.3, 6.4 Hz, 1H), 5.60 (dd, J = 8.4, 3.7 Hz, 1H), 5.24 (d, J = 3.9 Hz, 1H), 4.45 (s, 1H), 3.87 (s, 1H), 3.72–3.48 (m, 2H), 2.40 (s, 3H), 2.13 (dd, J = 12.7, 6.1 Hz, 1H). 13C NMR (125 MHz, DMSO-d6) δ 156.5, 152.2, 151.0, 150.2, 140.3, 129.7, 127.1, 119.5, 88.8, 86.1, 71.9, 62.7, 37.6, 21.4. ESI-MS (m/z) = 358 (M+ + H+).
8-(4-Biphenyl)-2′-deoxyguanosine (4p)16a. Representative procedure was followed by using 8-BrdG (173 mg, 0.5 mmol) and 4-biphenylboronic acid (198 mg, 1 mmol), yielded 4p (155 mg, 74% by filtration) in 12 h as off-white solid. UV absorption in methanol (125 μM) λmax = 309 nm. 1H NMR (500 MHz, DMSO-d6) δ 10.79 (s, 1H), 8.15–7.92 (m, 4H), 7.78 (d, J = 8.3 Hz, 2H), 7.67–7.52 (m, 3H), 6.45 (s, 2H), 6.18 (t, J = 7.3 Hz, 1H), 5.13 (d, J = 4.2 Hz, 1H), 5.02 (t, J = 5.6 Hz, 1H), 4.31 (s, 1H), 3.80 (d, J = 2.7 Hz, 1H), 3.72–3.53 (m, 2H), 2.06 (dd, J = 13.1, 6.4 Hz, 1H). 13C NMR (125 MHz, DMSO-d6): δ 157.0, 153.5, 152.5, 150.2, 147.4, 133.3, 132.8, 129.0, 128.5, 128.2, 128.1, 127.5, 127.2, 126.7, 117.6, 88.1, 84.9, 71.4, 62.4, 37.0. ESI-MS (m/z) = 420 (M+ + H+). Anal. calcd for C22H21N5O4: C, 63.00; H, 5.05; N, 16.70. Found: C, 63.09; H, 5.16; N, 16.79.
Recyclability study of column-free Suzuki–Miyaura cross-coupling using water-soluble PTABS ligand with Pd(OAc)2
To a solution of palladium acetate (2.24 mg, 1.0 mol%) and PTABS ligand (5.86 mg, 2.0 mol%) in degassed water (2.0 ml) at ambient temperature under N2 added 5-IdU (354 mg, 1.0 mmol) and the solution stirred for 5 min at 80 °C. After that reaction mixture allowed to cool at room temperature and then added 2-benzofuranyl boronic acid (243 mg, 1.5 mmol) along with triethylamine (0.28 ml, 2.0 mmol) and degassed water (4.0 ml). The resulting solution was then stirred at 80 °C for 3 hours. The reaction progress was observed by TLC analysis, after the completion of the reaction, the reaction mixture directly filtered on Buchner funnel, aqueous filtrate collected. The collected catalytic aqueous filtrate was recharged with 5-IdU (354 mg, 1.0 mmol), 2-benzofuranyl boronic acid (243 mg, 1.5 mmol), triethylamine (0.14 ml, 0.5 mmol) and the reaction was again continued with reported conditions.
Sonogashira coupling of 5-iodo-2′-deoxycytidine with alkynes
Representative procedure. To a solution of palladium acetate (1.12 mg, 1.0 mol%) and PTABS ligand (2.93 mg, 2.0 mol%) in degassed water
:
acetonitrile solution (1
:
1, 1.0 ml) at ambient temperature under N2 added 5-iodo-2′-deoxycytidine (177 mg, 0.5 mmol) and the solution stirred for 5.0 min at 80 °C. After that reaction mixture allowed to cool at room temperature and then added phenyl acetylene derivative (1.0 mmol) along with triethylamine (0.14 ml, 1.0 mmol) and degassed water
:
acetonitrile solution (1
:
1, 2.0 ml). The resulting solution was then stirred at 80 °C for 45 min. After the completion of the reaction, the solvent was removed in vacuo and the resultant residue obtained was purified using column chromatography in the CH2Cl2/MeOH solvent system (96
:
4) to afford the desired product (6a–e) as a white solid.
5-(Phenylethynyl)-2′-deoxycytidine (6a)29d. Representative procedure was followed by using phenyl acetylene (0.11 ml, 1 mmol), yielded 6a (123 mg, 75%) as off-white solid. UV absorption in methanol (125 μM) λmax = 286 nm. 1H NMR (500 MHz, DMSO-d6) δ 8.28 (s, 1H), 7.73 (s, 1H), 7.56 (dd, J = 7.5, 1.8 Hz, 2H), 7.41–7.29 (m, 3H), 6.98 (s, 1H), 6.09 (t, J = 6.4 Hz, 1H), 5.18 (d, J = 3.0 Hz, 1H), 5.07 (t, J = 4.7 Hz, 1H), 4.19 (s, 1H), 3.81–3.74 (m, 1H), 3.65–3.51 (m, 2H), 2.18–1.95 (m, 2H). 13C NMR (125 MHz, DMSO-d6) δ 164.2, 153.8, 145.3, 131.6, 128.8, 122.9, 94.1, 89.9, 87.8, 85.8, 82.0, 70.3, 61.3, 41.2.
5-((4-Pentylphenyl)ethynyl)-2′-deoxycytidine (6b)29e. Representative procedure was followed by using 1-ethynyl-4-pentylbenzene, yielded 6b (135 mg, 68%) as off-white solid. UV absorption in methanol (125 μM) λmax = 273 nm. 1H NMR (500 MHz, DMSO-d6) δ 8.28 (s, 1H), 7.73 (s, 1H), 7.48 (d, J = 8.2 Hz, 2H), 7.20 (d, J = 8.2 Hz, 2H), 6.97 (s, 1H), 6.12 (t, J = 6.5 Hz, 1H), 5.21 (d, J = 4.3 Hz, 1H), 5.10 (t, J = 5.0 Hz, 1H), 4.22 (td, J = 7.5, 3.8 Hz, 1H), 3.79 (q, J = 3.4 Hz, 1H), 3.67–3.54 (m, 2H), 2.57 (t, J = 7.6 Hz, 2H), 2.16 (ddd, J = 13.2, 6.0, 3.7 Hz, 1H), 2.01 (dt, J = 13.1, 6.5 Hz, 1H), 1.55 (dt, J = 14.9, 7.5 Hz, 2H), 1.32–1.19 (m, 4H), 0.84 (t, J = 7.1 Hz, 3H). 13C NMR (125 MHz, DMSO-d6) δ 164.2, 153.8, 145.0, 143.4, 131.6, 128.8, 120.1, 94.3, 90.1, 87.8, 85.8, 81.3, 70.3, 61.3, 41.2, 35.3, 31.2, 30.8, 22.3, 14.3. ESI-MS (m/z) = 398 (M+ + H+). Anal. calcd for C22H27N3O4: C, 66.48; H, 6.85; N, 10.57. Found: C, 66.38; H, 6.77; N, 10.51.
5-((4-Methoxyphenyl)ethynyl)-2′-deoxycytidine (6c). Representative procedure was followed by using 4-ethynylanisole, yielded 6c (134 mg, 75%) as off-white solid. UV absorption in methanol (125 μM) λmax = 275 nm. 1H NMR (500 MHz, DMSO-d6) δ 8.22 (s, 1H), 7.72 (s, 1H), 7.49 (d, J = 8.5 Hz, 2H), 6.94 (t, J = 11.7 Hz, 3H), 6.09 (t, J = 6.1 Hz, 1H), 5.13 (d, J = 45.0 Hz, 2H), 4.19 (s, 1H), 3.74 (s, 4H), 3.57 (dt, J = 13.7, 12.8 Hz, 2H), 2.16–2.08 (m, 1H), 1.99 (dd, J = 12.9, 6.1 Hz, 1H). 13C NMR (125 MHz, DMSO-d6) δ 164.1, 159.7, 153.7, 144.7, 141.5, 133.3, 114.8, 114.5, 94.2, 90.3, 87.8, 85.7, 80.3, 70.4, 61.3, 55.6. ESI-MS (m/z) = 358 (M+ + H+). Anal. calcd for C18H19N3O5: C, 60.50; H, 5.36; N, 11.76. Found: C, 60.42; H, 5.27; N, 11.66.
5-((4-Fluorophenyl)ethynyl)-2′-deoxycytidine (6d). Representative procedure was followed by using 1-ethynyl-4-fluorobenzene, yielded 6d (120 mg, 70%) as off-white solid. UV absorption in methanol (125 μM) λmax = 284, 306 nm. 1H NMR (500 MHz, DMSO-d6) δ 8.30 (s, 1H), 7.78 (s, 1H), 7.67–7.57 (m, 2H), 7.21 (t, J = 8.9 Hz, 2H), 7.08 (s, 1H), 6.09 (t, J = 6.3 Hz, 1H), 5.18 (d, J = 42.2 Hz, 2H), 4.22–4.17 (m, 1H), 3.77 (d, J = 3.2 Hz, 1H), 3.58 (dd, J = 29.5, 11.6 Hz, 2H), 2.14 (ddd, J = 12.8, 5.8, 3.8 Hz, 1H), 1.99 (dt, J = 13.0, 6.4 Hz, 1H). 13C NMR (125 MHz, DMSO) δ 164.1, 163.8, 153.7, 145.3, 133.9, 119.3, 116.1, 93.1, 89.8, 87.8, 85.8, 81.8, 70.3, 61.2, 41.2. ESI-MS (m/z) = 346 (M+ + H+). Anal. calcd for C17H16N3O4F: C, 59.13; H, 4.67; N, 12.17. Found: C, 59.05; H, 4.60; N, 12.08.
5-((4-Propylphenyl)ethynyl)-2′-deoxycytidine (6e)29e. Representative procedure was followed by using 1-ethynyl-4-propylbenzene, yielded 6e (144 mg, 78%) as off-white solid. UV absorption in methanol (125 μM) λmax = 273 nm. 1H NMR (500 MHz, DMSO-d6) δ 8.25 (s, 1H), 7.71 (s, 1H), 7.46 (d, J = 8.1 Hz, 2H), 7.18 (d, J = 8.0 Hz, 2H), 6.94 (s, 1H), 6.09 (t, J = 6.5 Hz, 1H), 5.18 (d, J = 4.3 Hz, 1H), 5.07 (t, J = 5.0 Hz, 1H), 4.19 (td, J = 7.7, 3.9 Hz, 1H), 3.77 (dd, J = 6.6, 3.2 Hz, 1H), 3.65–3.50 (m, 2H), 2.53 (t, J = 7.5 Hz, 2H), 2.17–1.94 (m, 2H), 1.60–1.49 (m, 2H), 0.84 (t, J = 7.3 Hz, 3H). 13C NMR (125 MHz, DMSO-d6) δ 164.2, 153.8, 145.0, 143.2, 131.6, 128.8, 120.1, 94.3, 90.1, 87.8, 85.8, 81.3, 70.3, 61.3, 41.2, 37.5, 24.2, 13.9. ESI-MS (m/z) = 370 (M+ + H+). Anal. calcd for C20H23N3O4: C, 65.03; H, 6.28; N, 11.37. Found: C, 64.92; H, 6.22; N, 11.28.
Sequential one-pot Sonogashira-cyclization reaction
Representative procedure. To a solution of palladium acetate (1.12 mg, 1.0 mol%) and PTABS ligand (2.93 mg, 2.0 mol%) in degassed water
:
acetonitrile solution (1
:
1, 1.0 ml) at ambient temperature under N2 added 5-iodo-2′-deoxyuridine (177 mg, 0.5 mmol) and the solution stirred for 5.0 min at 80 °C. After that reaction mixture allowed to cool at room temperature and then added phenyl acetylene (0.11 ml, 1.0 mmol) along with triethylamine (0.14 ml, 1.0 mmol) and degassed water
:
acetonitrile solution (1
:
1, 2.0 ml). The resulting solution was then stirred at 80 °C for 45 min. After the completion of the reaction, CuI (0.380 mg, 4.0 mol%), triethylamine (3.5 ml) and methanol (4.5 ml) was added and the solution was stirred for 4.0 h. After the completion of the reaction, the solvent was removed in vacuo and the resultant residue obtained was purified using column chromatography in the CH2Cl2/MeOH solvent system (96
:
4) to afford the desired product as a white solid.
3-(2′-Deoxy-ribofuranosyl)-6-(4-n-pentylphenyl)-2,3-dihydrofuro[2,3-d]pyrimidin-2-one (7a)29f. Representative procedure was followed by using 1-ethynyl-4-pentylbenzene, yielded 7a (149 mg, 75%) as off-white solid. UV absorption in methanol (125 μM) λmax = 281, 352 nm. 1H NMR (500 MHz, DMSO-d6) δ 8.80 (s, 1H), 7.68 (d, J = 8.0 Hz, 2H), 7.27 (d, J = 7.9 Hz, 2H), 7.16 (s, 1H), 6.14 (t, J = 5.9 Hz, 1H), 5.28 (d, J = 4.2 Hz, 1H), 5.16 (t, J = 4.9 Hz, 1H), 4.25–4.18 (m, 1H), 3.89 (d, J = 3.3 Hz, 1H), 3.69–3.55 (m, 2H), 2.56 (t, J = 7.3 Hz, 2H), 2.41–2.33 (m, 1H), 2.10–2.01 (m, 1H), 1.59–1.47 (m, 2H), 1.21 (dt, J = 14.1, 9.7 Hz, 4H), 0.81 (t, J = 6.7 Hz, 3H). 13C NMR (125 MHz, DMSO-d6) δ 171.4, 154.3, 154.2, 144.4, 138.2, 129.4, 126.2, 124.9, 107.3, 99.0, 88.5, 88.0, 69.9, 61.0, 41.6, 35.3, 31.2, 30.8, 22.3, 14.3.
3-(2′-Deoxy-ribofuranosyl)-6-(4-methoxyphenyl)-2,3-dihydrofuro[2,3-d]pyrimidin-2-one (7b)25. Representative procedure was followed by using 4-ethynylanisole, yielded 7b (107 mg, 60%) as white solid. UV absorption in methanol (125 μM) λmax = 285, 356 nm. 1H NMR (500 MHz, DMSO-d6) δ 8.75 (s, 1H), 7.73 (d, J = 8.7 Hz, 2H), 7.07 (s, 1H), 7.02 (d, J = 8.8 Hz, 2H), 6.15 (t, J = 6.0 Hz, 1H), 5.26 (d, J = 4.2 Hz, 1H), 5.14 (t, J = 5.1 Hz, 1H), 4.24–4.18 (m, 1H), 3.91–3.86 (m, 1H), 3.77 (s, 3H), 3.69–3.56 (m, 2H), 2.40–2.32 (m, 1H), 2.09–2.01 (m, 1H). 13C NMR (125 MHz, DMSO-d6) δ 171.4, 160.4, 154.3, 154.1, 137.6, 126.6, 121.3, 115.0, 107.5, 97.7, 88.5, 87.9, 69.9, 61.0, 55.7, 41.6.
3-(2′-Deoxy-ribofuranosyl)-6-(4-n-propylphenyl)-2,3-dihydrofuro[2,3-d]pyrimidin-2-one (7c)29e. Representative procedure was followed by using 1-ethynyl-4-propylbenzene, yielded 7c (129 mg, 70%) as off-white solid. UV absorption in methanol (125 μM) λmax = 281, 351 nm. 1H NMR (500 MHz, DMSO-d6) δ 8.82 (s, 1H), 7.72 (d, J = 8.2 Hz, 2H), 7.30 (d, J = 8.2 Hz, 2H), 7.19 (s, 1H), 6.18 (t, J = 6.1 Hz, 1H), 5.28 (d, J = 4.3 Hz, 1H), 5.15 (t, J = 5.2 Hz, 1H), 4.24 (dq, J = 8.4, 4.2 Hz, 1H), 3.92 (q, J = 3.6 Hz, 1H), 3.73–3.59 (m, 2H), 2.58 (t, J = 7.5 Hz, 2H), 2.40 (ddd, J = 13.4, 6.2, 4.4 Hz, 1H), 2.14–2.04 (m, 1H), 1.64–1.53 (m, 2H), 0.88 (t, J = 7.3 Hz, 3H). 13C NMR (125 MHz, DMSO-d6) δ 171.4, 154.3, 154.1, 144.2, 138.2, 129.4, 126.3, 124.9, 107.3, 99.1, 88.5, 88.0, 69.9, 61.0, 41.6, 37.4, 24.3, 14.0.
One-pot sequential Heck/Suzuki–Miyaura coupling
Representative procedure. To a solution of palladium acetate (1.12 mg, 1.0 mol%) and PTABS ligand (2.93 mg, 2.0 mol%) in degassed water
:
acetonitrile solution (2
:
1, 1.0 ml) at ambient temperature under N2 added 5-iodo-2′-deoxyuridine (177 mg, 0.5 mmol) and the solution stirred for 5.0 min at 80 °C. After that reaction mixture allowed to cool at room temperature and then added 4-bromo styrene (0.078 ml, 1.2 mmol) along with triethylamine (0.14 ml, 1.0 mmol) and degassed water: acetonitrile solution (2
:
1, 2.0 ml). The resulting solution was then stirred at 80 °C for 18 h. The reaction progress was observed by TLC analysis, after the completion of the reaction, palladium acetate (2.24 mg, 2 mol%) and S-Phos ligand (5.8 mg, 4.0 mol%) along with aryl boronic acid (1.0 mmol) followed by addition of degassed water
:
acetonitrile solution (2
:
1, 1.0 ml) at ambient temperature under N2 added and the reaction mixture was stirred at 80 °C for further 20 h. After the completion of the reaction, the solvent was removed in vacuo and the resultant residue obtained was purified using column chromatography in the CH2Cl2/MeOH solvent system (98
:
2) to afford the desired product as a yellow solid.
5-(4-Bromostyryl)-2′-deoxyuridine (8a).
Procedure.
To a solution of palladium acetate (1.12 mg, 1.0 mol%) and PTABS ligand (2.93 mg, 2.0 mol%) in degassed water
:
acetonitrile solution (2
:
1, 1.0 ml) at ambient temperature under N2 added 5-iodo-2′-deoxyuridine (177 mg, 0.5 mmol) and the solution stirred for 5.0 min at 80 °C. After that reaction mixture allowed to cool at room temperature and then added 4-bromo styrene (0.078 ml, 1.2 mmol) along with triethylamine (0.14 ml, 1.0 mmol) and degassed water
:
acetonitrile solution (2
:
1, 2.0 ml). The resulting solution was then stirred at 80 °C for 18 h. The reaction progress was observed by TLC analysis, after the completion of the reaction, the solvent was removed in vacuo and the resultant residue obtained was purified using column chromatography in the CH2Cl2/MeOH solvent system (98
:
2) to afford the desired product as off-white solid. Yielded 8a (360 mg, 88%). 1H NMR (500 MHz, DMSO-d6) δ 11.47 (s, 1H), 8.20 (s, 1H), 7.49 (d, J = 8.2 Hz, 2H), 7.36 (dd, J = 18.2, 12.4 Hz, 3H), 6.88 (d, J = 16.4 Hz, 1H), 6.15 (t, J = 6.4 Hz, 1H), 5.23 (d, J = 4.2 Hz, 1H), 5.14 (t, J = 4.8 Hz, 1H), 4.28–4.22 (m, 1H), 3.78 (d, J = 3.3 Hz, 1H), 3.67–3.55 (m, 2H), 2.19–2.09 (m, 2H). 13C NMR (125 MHz, DMSO-d6) δ 162.5, 149.8, 138.5, 137.8, 129.1, 128.0, 127.7, 126.4, 121.6, 111.1, 87.8, 84.8, 70.3, 61.4, 40.3. ESI-MS (m/z) = 410 (M+ + H+). Anal. calcd for C17H17N2O5Br: C, 49.89; H, 4.19; N, 6.85. Found: C, 49.79; H, 4.14; N, 6.76.
5-(4-(Benzofuran-2-yl)styryl)-2′-deoxyuridine (9a). Representative procedure was followed by using 2-benzofuranyl boronic acid (161 mg, 1.0 mmol), yielded 9a (312 mg, 70%) as yellow solid. UV absorption in methanol (125 μM) λmax = 350 nm. 1H NMR (500 MHz, DMSO-d6) δ 11.51 (s, 1H), 8.23 (s, 1H), 7.86 (d, J = 8.3 Hz, 2H), 7.67–7.50 (m, 4H), 7.46–7.38 (m, 2H), 7.31–7.20 (m, 2H), 6.96 (d, J = 16.5 Hz, 1H), 6.15 (t, J = 6.6 Hz, 1H), 5.29–5.18 (m, 2H), 4.29–4.22 (m, 1H), 3.81–3.75 (m, 1H), 3.69–3.55 (m, 2H), 2.20–2.09 (m, 2H). 13C NMR (125 MHz, DMSO-d6) δ 162.5, 155.4, 149.8, 138.8, 138.4, 132.9, 132.7, 129.3, 128.7, 127.2, 127.0, 125.4, 125.0, 122.4, 121.5, 111.4, 111.1, 102.3, 87.8, 84.8, 70.3, 61.4, 35.4. ESI-MS (m/z) = 447 (M+ + H+). Anal. calcd for C25H22N2O6: C, 67.26; H, 4.97; N, 6.27. Found: C, 67.15; H, 4.88; N, 6.19.
5-(4-(Naphth-2-yl) styryl)-2′-deoxyuridine (9b). Representative procedure was followed by using 2-benzofuranyl boronic acid (161 mg, 1.0 mmol), yielded 9b (310 mg, 68%) as yellow solid. UV absorption in methanol (125 μM) λmax = 334 nm. 1H NMR (500 MHz, DMSO-d6) δ 11.50 (s, 1H), 8.21 (d, J = 14.9 Hz, 1H), 7.86 (d, J = 7.4 Hz, 2H), 7.70–7.08 (m, 9H), 6.92 (dd, 16.4 Hz, 2H), 6.18–6.11 (m, 1H), 5.28–5.16 (m, 2H), 4.29–4.22 (m, 1H), 3.81–3.75 (m, 1H), 3.68–3.55 (m, 2H), 2.14 (m, 2H). 13C NMR (125 MHz, DMSO-d6) δ 162.5, 154.6, 149.8, 138.8, 138.4, 129.3, 128.7, 127.2, 127.0, 125.4, 125.1, 125.0, 123.6, 122.4, 121.5, 111.4, 111.1, 110.4, 108.9, 102.3, 87.8, 84.8, 70.3, 61.4, 40.5. ESI-MS (m/z) = 457 (M+ + H+). Anal. calcd for C27H24N2O5: C, 71.04; H, 5.30; N, 6.14. Found: C, 70.93; H, 5.24; N, 6.02.
Synthesis of anti-viral drug BVDU via palladium-catalyzed Heck reaction using the PTABS/Pd system
Step-A. To a solution of palladium acetate (2.24 mg, 1.0 mol%) and PTABS ligand (5.86 mg, 2.0 mol%) in degassed water: acetonitrile solution (1
:
1, 2.0 ml) at ambient temperature under N2 added 5-iodo-2′-deoxycytidine (354 mg, 1.0 mmol) and the solution stirred for 5 min at 80 °C. After that reaction mixture allowed to cool at room temperature and then added methyl acrylate (0.18 ml, 2.0 mmol) along with triethylamine (0.27 ml, 2.0 mmol) and degassed water
:
acetonitrile solution (1
:
1, 4.0 ml). The resulting solution was then stirred at 80 °C for 4 h. After the completion of the reaction, the solvent was removed in vacuo and the resultant residue obtained was purified using column chromatography in the CH2Cl2/MeOH solvent system (96
:
4) to afford the desired 10a product as a white solid (271 mg, 87%).
Step-B. (E)-5-[2-Carbomethoxyvinyl]-2′-deoxyuridine (dUMA) (265 mg, 0.85 mmol) was dissolved in 1 M NaOH (17 ml) and the mixture stirred for 3 h, filtered and filtrate adjusted to pH 4–6 with 1 M HCl. On cooling at 4 °C a white precipitate formed. This was filtered off and washed with cold water (1 ml × 3 times) and acetone (1 ml × 2 times) and dried under vacuum to give 10b, white solid (280 mg, 91%).
Step-C. To a solution of (E)-5-(2-carboxyvinyl)-2′-deoxyuridine (280 mg, 0.84 mmol) in DMF (5 ml) was added K2CO3 (226 mg, 1.68 mmol) and the suspension stirred at room temperature for 15 min. Then added NBS (149 mg, 0.84 mmol) fraction-wise over 30 min at ambient temperature. After completion of reaction, the solvent was removed under vacuo and the resultant residue obtained was purified using column chromatography in CH2Cl2
:
MeOH solvent system (96
:
4) to afford the desired product 10c, a white solid (254 mg, 91%).
(E)-5-[2-Carbomethoxyvinyl]-2′-deoxyuridine (10a)19. 1H NMR (400 MHz, DMSO-d6) δ 11.62 (s, 1H), 8.38 (s, 1H), 7.32 (d, J = 15.8 Hz, 1H), 6.81 (d, J = 15.8 Hz, 1H), 6.08 (t, J = 6.4 Hz, 1H), 5.23 (d, J = 4.3 Hz, 1H), 5.14 (t, J = 5.1 Hz, 1H), 4.21 (dt, J = 8.6, 4.2 Hz, 1H), 3.75 (d, J = 3.3 Hz, 1H), 3.70–3.47 (m, 5H), 2.19–2.06 (m, 2H). 13C NMR (101 MHz, DMSO-d6) δ 167.6, 162.1, 149.6, 144.4, 138.5, 116.5, 108.5, 88.0, 85.2, 70.1, 61.2, 51.7, 40.4.
(E)-5-[2-Carboxyvinyl]-2′-deoxyuridine (10b)19. 1H NMR (400 MHz, DMSO-d6) δ 12.12 (s, 1H), 11.58 (s, 1H), 8.34 (s, 1H), 7.24 (d, J = 15.8 Hz, 1H), 6.73 (d, J = 15.8 Hz, 1H), 6.09 (t, J = 6.2 Hz, 1H), 5.19 (d, J = 31.0 Hz, 2H), 4.21 (s, 1H), 3.75 (d, J = 3.0 Hz, 1H), 3.57 (dd, J = 31.9, 11.6 Hz, 2H), 2.20–2.02 (m, 2H). 13C NMR (101 MHz, DMSO-d6) δ 168.4, 162.1, 149.6, 143.9, 137.9, 118.1, 108.7, 88.0, 85.1, 70.1, 61.2, 40.4.
(E)-5-[2-Bromovinyl]-2′-deoxyuridine (BVDU) (10c)19. 1H NMR (400 MHz DMSO-d6) δ 11.54 (s, 1H), 8.03 (s, 1H), 7.20 (d, J = 13.5 Hz, 1H), 6.81 (d, J = 13.5 Hz, 1H), 6.08 (t, J = 6.4 Hz, 1H), 5.23 (d, J = 4.2 Hz, 1H), 5.06 (t, J = 5.1 Hz, 1H), 4.22–4.17 (m, 1H), 3.74 (dd, J = 6.2, 2.9 Hz, 1H), 3.60–3.49 (m, 2H), 2.12–2.05 (m, 2H). 13C NMR 161.5, 149.4, 139.4, 129.8, 109.5, 106.4, 87.5, 84.4, 69.7, 60.9, 45.6.
Acknowledgements
A. R. K. and C. S. acknowledge The Alexander von Humboldt Foundation for the research cooperation programme and is also thanked for the equipment grant to A. R. K. We also thank the University Grants Commission India for a UGC-SAP fellowship for V. G. and S. B., UGC-FRP position and funding for A. R. K. and a UGC-CSIR fellowship for A. A.
References
- Chemistry of Nucleosides and Nucleotides, ed. L. B. Townsend, Springer, 1994, vol. 3 Search PubMed.
- E. De Clercq, Nucleosides, Nucleotides Nucleic Acids, 2009, 28, 586 CAS.
-
(a) T. Robak, Clin. Cancer Drugs, 2014, 1, 2 CrossRef CAS;
(b) L. P. Jordheim, D. Durantel, F. Zoulim and C. Dumontet, Nat. Rev. Drug Discovery, 2013, 12, 447 CrossRef CAS PubMed.
-
(a) R. W. Sinkeldam, N. J. Greco and Y. Tor, Chem. Rev., 2010, 110, 2579 CrossRef CAS PubMed;
(b) A. A. Tanpure, M. G. Pawar and S. G. Srivatsan, Isr. J. Chem., 2013, 53, 366 CrossRef CAS;
(c) A. Matarazzo and R. H. E. Hudson, Tetrahedron, 2015, 71, 1627 CrossRef CAS.
-
(a) Antiviral Nucleosides, ed. C. Chu, Elsevier Science, 2003 Search PubMed;
(b) Y. S. Lee, S. M. Park and B. H. Kim, Bioorg. Med. Chem. Lett., 2009, 19, 1126 CrossRef CAS PubMed.
- V. L. Damaraju, S. Damaraju, J. D. Young, S. A. Baldwin, J. Mackey, M. B. Sawyer and C. E. Cass, Oncogene, 2003, 22, 7524 CrossRef CAS PubMed.
-
(a) Metal-Catalyzed Cross-Coupling Reactions, ed. A. de Meijere and F. Diederich, Wiley−VCH, 2004, vol. 2 Search PubMed;
(b) Cross-Coupling and Heck-Type Reactions, Workbench Edition, ed. G. Molander, C. Wolfe and M. Larhed, Science of Synthesis. Thieme Chemistry, 2013, vol. 1−3 Search PubMed.
-
(a) S. De Ornellas, T. J. Williams, C. G. Baumann and I. J. S. Fairlamb, in C–H and C–X Bond Functionalization: Transition Metal Mediation, ed. X. Ribas, RSC Catalysis Series No. 11, RSC, Dorchester, 2013 Search PubMed;
(b) K. H. Shaughnessy, Molecules, 2015, 20, 9419 CrossRef CAS PubMed.
- A. Suzuki, Angew. Chem., Int. Ed., 2011, 50, 6722 CrossRef CAS PubMed.
-
(a) Palladium Reagents in Organic Syntheses, ed. R. F. Heck, Academic, New York, 1985 Search PubMed;
(b) Palladium Reagents and Catalysts, ed. J. Tsuji, Wiley, Chichester, 1995 Search PubMed.
- K. Sonogashira, Y. Tohda and N. Hagihara, Tetrahedron Lett., 1975, 16, 4467 CrossRef.
- R. A. Sheldon, Green Chem., 2005, 7, 267 RSC.
- Green Techniques for Organic Synthesis and Medicinal Chemistry, ed. W. Zhang and B. W. Cue, Wiley, 2012 Search PubMed.
-
(a) H. C. Hailes, Org. Process Res. Dev., 2007, 11, 114 CrossRef CAS;
(b) A. Chanda and V. V. Fokin, Chem. Rev., 2009, 109, 725 CrossRef CAS PubMed.
-
(a) E. Mayer, L. Valis, R. Huber, N. Amann and H. A. Wagenknecht, Synthesis, 2003, 15, 2335 Search PubMed;
(b) E. C. Western, J. R. Daft, E. M. Johnson II, P. M. Gannett and K. H. Shaughnessy, J. Org. Chem., 2003, 68, 6767 CrossRef CAS PubMed;
(c) P. Čapek and M. Hocek, Synlett, 2005, 19, 3005 Search PubMed;
(d) P. Čapek, R. Pohl and M. Hocek, Org. Biomol. Chem., 2006, 4, 2278 RSC;
(e) M. Vrábel, R. Pohl, B. Klepetářová, I. Votruba and M. Hocek, Org. Biomol. Chem., 2007, 5, 2849 RSC;
(f) M. Vrábel, R. Pohl, I. Votruba, M. Sajadi, S. A. Kovalenko, N. P. Ernsting and M. Hocek, Org. Biomol. Chem., 2008, 6, 2852 RSC;
(g) V. Vongsutilers, J. R. Daft, K. H. Shaughnessy and P. M. Gannett, Molecules, 2009, 14, 3339 CrossRef CAS PubMed;
(h) N. Fresneau, M. A. Hiebel, L. A. Agrofoglio and S. Berteina-Raboin, Molecules, 2012, 17, 14409 CrossRef CAS PubMed;
(i) T. Lussier, G. Herve, G. Enderlin and C. Len, RSC Adv., 2014, 4, 46218 RSC. For more examples of palladium-catalyzed Suzuki-Miyaura modification of nucleosides or nucleotides see:
(j) N. Amann, E. Pandurski, T. Fiebig and H. A. Wagenknecht, Chem.–Eur. J., 2002, 8, 4877 CrossRef CAS PubMed;
(k) N. Amann and H. A. Wagenknecht, Synlett, 2002, 5, 687 CrossRef;
(l) M. F. Jacobsen, E. E. Ferapontova and K. V. Gothelf, Org. Biomol. Chem., 2009, 7, 905 RSC;
(m) A. Okamoto, T. Inasaki and I. Saito, Tetrahedron Lett., 2005, 46, 791 CrossRef CAS;
(n) N. Fresneau, M. A. Hiebel, L. A. Agrofoglio and S. Berteina-Raboin, Molecules, 2012, 17, 14409 CrossRef CAS PubMed;
(o) E. Mayer, L. Valis, R. Huber, N. Amann and H. A. Wagenknecht, Synthesis, 2003, 15, 2335 Search PubMed;
(p) T. Lussier, G. Herve, G. Enderlin and C. Len, RSC Adv., 2014, 4, 46218 RSC;
(q) V. Vongsutilers, J. R. Daft, K. H. Shaughnessy and P. M. Gannett, Molecules, 2009, 14, 3339 CrossRef CAS PubMed;
(r) A. A. H. Elmehriki, M. Suchy, K. J. Chicas, F. Wojciechowski and R. H. E. Hudson, Artif. DNA PNA XNA, 2014, 5, e29174 CrossRef PubMed;
(s) A. Matarazzo and A. H. E. Hudson, Tetrahedron, 2015, 71, 1627 CrossRef CAS.
-
(a) A. R. Kapdi, V. Gayakhe, Y. S. Sanghvi, J. Garcia, P. Lozano, I. da Silva, J. Perez and J. L. Serrano, RSC Adv., 2014, 4, 17567 RSC;
(b) V. Gayakhe, A. Ardhapure, A. R. Kapdi, Y. S. Sanghvi, J. L. Serrano, L. Garcia, J. Perez, J. Garcia, G. Sanchez, C. Fischer and C. Schulzke, J. Org. Chem., 2016, 81, 2713 CrossRef CAS PubMed.
- For details on column-free protocol see: A. R. Kapdi, A. Ardhapure and Y. S. Sanghvi, Synthesis, 2015, 47, 1163 CrossRef CAS.
- Recoverable and Recyclable Catalysts, ed. M. Benaglia, Wiley, 2009 Search PubMed.
- Only example showing the recyclability in Heck alkenylation of nucleosides was reported recently A. V. Ardhapure, Y. S. Sanghvi, A. R. Kapdi, J. Garcia, G. Sanchez, P. Lozano and J. L. Serrano, RSC Adv., 2015, 5, 24558 RSC.
- For more examples of palladium-catalyzed Sonogashira alkynylation of nucleosides see:
(a) E. Petricci, M. Radi, F. Corelli and M. Botta, Tetrahedron Lett., 2003, 44, 9181 CrossRef CAS;
(b) N. K. Garg, C. C. Woodroofe, C. J. Lacenere, S. R. Quake and B. M. Stoltz, Chem. Commun., 2005, 4551 RSC;
(c) M. Hocek and M. Fotja, Org. Biomol. Chem., 2008, 6, 2233 RSC;
(d) J. H. Cho, C. D. Prickett and K. H. Shaughnessy, Eur. J. Org. Chem., 2010, 3678 CrossRef CAS;
(e) D. K. Kolmel, L. J. Barandun and E. T. Kool, Org. Biomol. Chem., 2016, 14, 6407 RSC.
- For general copper-free Sonogashira coupling protocols see:
(a) B. Liang, M. Dai, J. Chen and Z. Yang, J. Org. Chem., 2005, 70, 391 CrossRef CAS PubMed;
(b) T. Ljungdahl, T. Bennur, A. Dallas, H. Emtenas and J. Martensson, Organometallics, 2008, 27, 2490 CrossRef CAS;
(c) Z. Gu, Z. Li, Z. Liu, Z. Wang, C. Liu and J. Xiang, Catal. Commun., 2008, 2154 CrossRef CAS;
(d) A. Komaromi and Z. Novak, Chem. Commun., 2008, 4968 RSC;
(e) H. Zhong, J. Wang, L. Li and R. Wang, Dalton Trans., 2014, 43, 2098 RSC.
- L. Yuan, Z. Zhang, X. Xu and X. Zhou, Synth. Commun., 2014, 44, 1007 CrossRef CAS.
-
(a) C. McGuigan, C. J. Yarnold, G. Jones, S. Velazquez, H. Barucki, A. Brancale, G. Andrei, R. Snoeck, E. De Clercq and J. Balzarini, J. Med. Chem., 1999, 42, 4479 CrossRef CAS PubMed;
(b) E. De Clercq, Nucleosides, Nucleotides Nucleic Acids, 2004, 23, 457 CrossRef CAS PubMed;
(c) V. Aucagne, F. Amblard and L. A. Agrofoglio, Synlett, 2004, 2406 CAS;
(d) C. McGuigan, R. N. Pathirana, R. Snoeck, G. Andrei, E. De Clercq and J. Balzarini, J. Med. Chem., 2004, 47, 1847 CrossRef CAS PubMed;
(e) M. Migliore, Antiviral Chem. Chemother., 2010, 20, 107 CrossRef CAS PubMed.
- Sonogashira/cyclization strategy has been used for general substrates:
(a) G. W. Kabalka, L. Wang and R. M. Pagni, Tetrahedron, 2001, 57, 8017 CrossRef CAS;
(b) S. Debnath and S. Mondal, J. Org. Chem., 2015, 80, 3940 CrossRef CAS PubMed;
(c) K. Shekarrao, P. P. Kaishap, S. Gogoi and R. C. Boruah, Adv. Synth. Catal., 2015, 357, 1187 CrossRef CAS. Sonogashira/cyclization strategy for obtaining FV-100 previously reported
(d) G. M. Luoni, C. McGuigan, G. Andrei, R. Snoeck, E. De Clercq and J. Balzarini, Antiviral Chem. Chemother., 2004, 15, 333 CrossRef CAS;
(e) M. J. Robbins and P. J. Barr, Tetrahedron Lett., 1981, 22, 421 CrossRef , although in this case it was not one-pot.
- N. Fresneau, M.-A. Hiebel, L. A. Agrofoglio and S. Berteina-Raboin, Tetrahedron Lett., 2012, 53, 1760 CrossRef CAS.
- For more examples of palladium-catalyzed Heck alkenylation of nucleosides see:
(a) V. Aucagne, S. Berteina-Raboin, P. Guenot and L. A. Agrofoglio, J. Comb. Chem., 2004, 6, 717 CrossRef CAS PubMed;
(b) P. Lagisetty, L. Zhang and M. K. Lakshman, Adv. Synth. Catal., 2008, 350, 602 CrossRef CAS;
(c) M. V. Reddington and D. Cunningham-Bryant, Tetrahedron Lett., 2011, 52, 181 CrossRef CAS;
(d) J. Dadová, P. Vidláková, R. Pohl, L. Havran, M. Fojta and M. Hocek, J. Org. Chem., 2013, 78, 9627 CrossRef PubMed;
(e) G. Herve and C. Len, RSC Adv., 2014, 4, 46926 RSC.
- One-pot sequential Heck/Suzuki coupling strategy has been used for general substrates:
(a) L. Joucla, G. Cusati, C. Pinel and L. Djakovitch, Adv. Synth. Catal., 2010, 352, 1993 CrossRef CAS;
(b) P. Cotugno, A. Monopoli, F. Ciminale, N. Cioffi and A. Nacci, Org. Biomol. Chem., 2012, 10, 808 RSC.
- X. Rabasseda, Drugs Today, 2003, 39, 359 CrossRef CAS PubMed.
-
(a) C. H. Macickova, R. Pohl, P. Horakova, L. Havran, J. Spacek, M. Fojta and M. Hocek, Chem.–Eur. J., 2011, 17, 5833 CrossRef PubMed;
(b) K. M. Rankin, M. Sproviero, K. Rankin, P. Sharma, S. D. Wetmore and R. A. Manderville, J. Org. Chem., 2012, 77, 10498 CrossRef CAS PubMed;
(c) B. C. Train, S. A. Bilgesü, E. C. Despeaux, V. Vongsutilers and P. M. Gannett, Chem. Res. Toxicol., 2014, 27, 1176 CrossRef CAS PubMed;
(d) D. Rai, M. Johar, T. Manning, B. Agrawal, D. Y. Kunimoto and R. Kumar, J. Med. Chem., 2005, 48, 7012 CrossRef CAS PubMed;
(e) C. McGuigan, H. Barucki, S. Blewett, A. Carangio, J. T. Erichsen, G. Andrei, R. Snoeck, E. D. Clercq and J. Balzarini, J. Med. Chem., 2000, 43, 4993 CrossRef CAS PubMed;
(f) A. Angell, C. McGuigan, L. G. Sevillano, R. Snoeck, G. Andrei, E. D. Clercq and J. Balzarini, Bioorg. Med. Chem. Lett., 2004, 14, 2397 CrossRef CAS PubMed.
Footnote |
† Electronic supplementary information (ESI) available. CCDC 1489309. For ESI and crystallographic data in CIF or other electronic format see DOI: 10.1039/c6ra19039a |
|
This journal is © The Royal Society of Chemistry 2016 |