DOI:
10.1039/C6RA18971G
(Paper)
RSC Adv., 2016,
6, 80286-80295
Synthesis, structure, and calf-thymus DNA binding of ternary fleroxacin–Cu(II) complexes†
Received
26th July 2016
, Accepted 18th August 2016
First published on 19th August 2016
Abstract
Two mononuclear copper(II) (Cu(II)) complexes containing quinolone drug fleroxacin (flrx) were synthesized in the presence of 1,10-phenanthrolin (phen) and 2,2′-bipyridine (bipy). To fully characterize these two complexes, single-crystal X-ray diffraction study and other characterizations were employed. X-ray crystallography results showed that the deprotonated flrx coordinated with the central Cu(II) via one pyridone oxygen and one carboxylate oxygen. Intermolecular interactions including hydrogen bonding and π–π stacking stabilized these complexes. Moreover, interactions of [Cu(flrx)(bipy)Cl]·4H2O and [Cu(flrx)(phen)·H2O]·9.8H2O complexes with calf-thymus DNA (CT DNA) were investigated. Fluorescence measurements and fluorescence lifetime investigations indicated that CT DNA could effectively quench the fluorescence of the complexes and the interaction modes were static quenching. Isothermal titration calorimetry showed that the two complexes exhibited a similar moderate binding affinity to CT DNA, and their binding constants were basically consistent with the results of the fluorescence measurements. Thermodynamic parameters revealed that the bindings were driven by considerable enhancements in beneficial entropy and unfavorable increases in enthalpy, and the negative Gibbs energy values indicated that the interactions were spontaneous. Circular dichroism, interaction with denatured CT DNA, and iodide quenching studies suggested that the possible interaction modes between CT DNA and these two complexes are both in the intercalation mode.
Introduction
Quinolones are a large class of synthetic antibacterial drugs with a 4-oxo-1,4-dihydroquinoline skeleton. These types of agents target topoisomerases II and IV, which influence DNA replication and transcription.1,2 Quinolones are not only widely used to treat various infections, but also effective for many cancers, such as human breast cancer and prostate cancer.3–5
Coordination between metal ions and adjacent carbonyl and carboxyl groups of quinolones has attracted considerable attention in the past because some of these modified quinolone derivatives exhibit favorable biological properties and improved resistance properties.6,7 Diverse metal–quinolone complexes have been proven to exhibit antibacterial activity,8 anticancer activity,9 and binding affinity to biomacromolecules.10 Among all these complexes, the mixed ligand complexes of quinolones stood out from the numerous quinolone complexes because of their noteworthy biological activities.11 A considerable number of ternary complexes exhibit surprising biological performances in the presence of a nitrogendonor heterocycle.12,13 Researches on the interaction between quinolone ternary complexes and DNA have shown that the complexes exhibit enhanced binding affinity.14,15 Besides, given that copper(II) (Cu(II)) plays an important role on numerous biological functions and influences gene expression in mammalian cells, its complexes attracted significant attention compared with other conventional metal–drug complexes.16,17
Fleroxacin (flrx) is a third-generation quinolone drug that efficiently inhibits Gram-positive and Gram-negative bacteria.18 Although the coordination of some quinolones with metals has been extensively investigated, only a few studies have focused on flrx complexes. In addition, the reported flrx complexes were simply characterized without obtaining their crystal structures.19,20
DNA is an important macromolecule that carries hereditary information, controls cellular structure and function, and plays an essential role in cellular progression, such as DNA transcription, translation, and replication.21 Cellular DNA is the target of various drugs, including antibacterial and anticancer drugs.22,23 Given that quinolone itself is an antibacterial drug and its complexes show antibacterial activities and certain anticancer effects, binding between quinolone complexes and DNA has been widely investigated.24,25
In our previous work, a mononuclear Cu(II) complex, [Cu(flrx)(phen)·H2O]·9.8H2O, was synthesized and fully characterized.26 The ability of this complex to cleave DNA and to interact with human serum albumin was investigated. As a continuation of our previous work, the present study investigated the coordination of flrx with Cu(II) in the presence of 2,2′-bipyridine (bipy). The obtained crystal was characterized by X-ray crystallography. Further characterizations were applied to confirm the structure of the crystal. Considering the advanced anticancer and antibacterial abilities of the ternary complexes of quinolones,12,27 we presented the DNA binding properties of flrx complexes in this article. The interactions of [Cu(flrx) (bipy)Cl]·4H2O (complex 1) and [Cu(flrx)(phen)·H2O]·9.8H2O (complex 2) with calf-thymus DNA (CT DNA) were investigated. Fluorescence studies were conducted to determine the effects of CT DNA on the intrinsic fluorescence of these two complexes. Time-resolved fluorescence lifetime determination was conducted to confirm the quenching type. Isothermal titration calorimetry (ITC) measurements were performed to probe the binding affinities and thermodynamic variables. Further analyses, covering circular dichroism (CD), interaction with single-stranded CT DNA (ss-CT DNA), and iodide quenching studies, were employed to assess the binding modes.
Experimental
Materials
Flrx and bipy, which were used to synthesize complex 1, were procured from J&K Scientific Ltd. (Beijing, China). CT DNA was purchased from Sigma Chemical Co. (St. Louis, MO, USA). The absorbance ratio of CT DNA at 260/280 nm was 1.8 to 1.9, indicating that CT DNA was sufficiently free of protein contamination.28 DNA stock solution was prepared with phosphate buffer solution (PBS, pH 7.40) and stored at 0 °C for no more than one week. Other analytical grade reagents and chemicals were obtained from Chengdu Kelong Chemical Co., Ltd. (China, Chengdu).
Synthesis and characterization
Synthesis of complex 1 and 2. For complex 1, 0.5 mmol flrx (184.67 mg) was dissolved in 15 mL methanolic solution, and an equal molar amount of NaOH (0.5 mmol, 20 mg) was added. After 30 min of stirring, deprotonated flrx and bipy (78.09 mg, 0.5 mmol) in methanol (5 mL) were added dropwise into an aqueous solution (5 mL) of CuCl2·2H2O (0.5 mmol, 85.24 mg). The mixture was reacted for 2 h at room temperature and left for slow evaporation. Blue crystals were deposited for more than one month with a yield of approximately 78%. Anal. calcd for C27H33ClCuF3N5O7 (MW = 695.57) C 46.62%, H 4.78%, N 10.07%; found: C 45.97%, H 4.78%, N 10.83%. IR: νmax, cm−1; ν(C
O)pyridine, 1633; νasym (CO2), 1589; νsym (CO2), 1369; Δ = ν(CO2)asym − ν(CO2)sym: 220 cm−1 (KBr disk). The obtained complex is soluble in water, methanol, and ethanol. Complex 2 had been prepared via the same process, except that bipy (78.09 mg, 0.5 mmol) was replaced by 1,10-phenanthrolin (phen; 99.11 mg, 0.5 mmol).26
Single-crystal X-ray diffraction. A suitable crystal was selected and measured on the Xcalibur Eos diffractometer at 293.15 K. Olex2 was used to elucidate the structure of the complex.29 Moreover, the structure was solved using the ShelXS structure solution program through direct methods and refined with the ShelXL refinement package using least squares minimization.30 Complex 1 and 2 were measured using the same method.
Characterization. Fourier transform infrared (FTIR) spectroscopy was performed on a Nicolet-6700 FTIR spectrometer (Thermo Fisher Scientific, USA). Elemental determination (C, H, and N) was carried out on a Euro EA 3000 elemental analyzer (Leeman, USA). A thermogravimetric (TG) analyzer (TG209F1 Iris, Netzsch, Selb, Germany) was employed to analyze the thermal stabilization of the complex. The measured temperature range was 30 °C to 800 °C at a heating rate of 10 °C min−1 in air. Differential scanning calorimetry (DSC) was conducted via the DSC 8500 (PerkinElmer, USA) with N2 as purge gas to obtain information of the melting point. The temperature range was 20 °C to 300 °C at a heating rate of 10 °C min−1. Both complexes were characterized in a similar manner.It's necessary to produce stability investigation on the complexes in the PBS buffer (pH 7.40), which was employed for the next biological studies. The Cary Eclipse fluorescence spectrophotometer (Varian, USA) was used to monitor whether changes have occurred.
Interaction with CT DNA
Fluorescence measurements. Fluorescence spectra were recorded on the Cary Eclipse fluorescence spectrophotometer (Varian, USA) using 1.0 cm quartz cells. The excitation and emission slit widths were set at 5 and 10 nm with 280 nm as excitation wavelength. Fluorescence was measured by maintaining a constant concentration (2 μM) of the two complexes and varying the DNA concentrations from 2.88 μM to 17.28 μM at 298 K.Inner filter effect was considered and corrected in this study. All fluorescence intensities were corrected for the absorption of excited light and the re-absorption of emitted light by applying the following equation:31
|
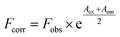 | (1) |
where
Fcorr and
Fobs are the corrected and original fluorescence intensities, respectively.
Aex and
Aem represent the absorption values corresponding to the excitation and emission wavelengths, respectively.
Time-resolved fluorescence lifetime determination. Time-resolved fluorescence lifetime determination was performed on the Horiba JobinYvonFluoroMax-4 spectrofluorometer (Horiba, France) with 280 and 415 nm as excitation and emission wavelengths, respectively. The concentration of the two complexes was 8 μM, and the corresponding DNA concentrations were 40 and 80 μM. PBS (pH 7.40) was used to prepare the samples.
ITC measurements. The isothermal titration calorimeter CN-ITC200 (MicroCal; USA) was applied to monitor the binding interactions of the two complexes with CT DNA. ITC-ready samples were prepared using degassed PBS (pH 7.40) to eliminate the influence of gas bubbles. All experiments were conducted at 298 K under stirring at 750 rpm. The solution of the complexes (0.5 mM) in the syringe was titrated to the CT DNA solution (0.02 mM) in the cell with successive injections. The reference cell contained double distilled water. After the data were corrected for heats of dilution, the heat curves generated by titrations were integrated to assess the heat related to the injection by MicroCal Origin software.
CD spectral measurements. CD spectra of free DNA and those mixed with complexes were recorded on a CD spectrometer (Model 400, Aviv, USA) using a 2 mm path length cell at 298 K. CD measurements were performed at a constant DNA concentration of 1 mM in the absence and presence of the two complexes (0.2 mM). The optical chamber of the CD spectrometer was deoxygenated with dry nitrogen prior to use and surrounded with nitrogen atmosphere during the experiments. Each sample was scanned thrice and averaged for the subsequent analysis.
Iodide quenching studies. Iodide quenching determinations were conducted using the aforementioned fluorescence spectrophotometer. Different concentrations of potassium iodide (KI) solution (2 mM to 12 mM) were added to the complexes (2 μM) and complex – CT DNA (2 μM complexes and 11.52 μM CT DNA) systems. The entire process was conducted at 280 nm excitation. Quenching effects were used to evaluate the binding modes of the complexes with CT DNA. Each experiment was performed in triplicate.
Interaction with ss-CT DNA. ds-CT DNA was treated in boiling water for 30 min and immediately cooled in ice water for 10 min to prepare ss-CT DNA.32 The fluorescence quenching effects of ss-CT DNA and ds-CT DNA on complexes provided a criterion for determining the binding mode. Fluorescence was measured by maintaining a constant concentration (2 μM) for the two complexes and varying the ss-CT DNA concentrations from 2.88 μM to 17.28 μM at 280 nm excitation wavelength. Each experiment was repeated three times.
Results and discussion
Crystal structure of complex 1
The crystallographic data of complex 1 are summarized in Table 1, which shows that the synthesized coordination compound behaves as P
space group in triclinic form. The crystal structure of the mononuclear complex 1 is shown in Fig. 1. Selected bond distances and angles of complex 1 are depicted in Table 2. For complex 1, the central Cu(II) is chelated to one pyridone oxygen atom and one carboxylate oxygen atom of the deprotonated flrx and to two nitrogen atoms from the pyridine rings of the bipy molecule, which is similar to the structure of complex 2. According to the angles of Cu(II) and the chlorine and oxygen atoms [O(1)–Cu(1)–Cl(1) = 97.66 (6)°, O(2)–Cu(1)–Cl(1) = 94.67(5)°], the chlorine atom from metal salt occupies the axial position. Moreover, four lattice water molecules providing hydrogen bond interactions to strengthen crystalline stability are included. As an index of the degree of trigonality to the five-coordinate structures, trigonality index t (t = (Φ1 − Φ2)/60, where Φ1 and Φ2 represent the two largest angles in the coordination sphere, respectively) was applied. t = 0 denotes a perfect square pyramid, while t = 1 indicates a perfect trigonal bipyramid.33 The calculated t = (168.43–158.50)/60 = 0.165 reveals that complex 1 behaved with distortion from the regular square-based pyramidal geometry, which is also observed in other similar Cu–quinolones complexes.34,35
Table 1 Crystal and experimental data of complex 1
Empirical formula |
Complex 1 |
Formula weight |
695.57 |
Temperature/K |
293.15 K |
Crystal system |
Triclinic |
Space group |
P![[1 with combining macron]](https://www.rsc.org/images/entities/char_0031_0304.gif) |
a/Å |
9.4109(7) |
b/Å |
11.7658(8) |
c/Å |
15.5101(7) |
α/° |
77.188(5) |
β/° |
80.638(5) |
γ/° |
67.200(7) |
Volume/Å3 |
1538.13(17) |
Z |
2 |
ρcalc/g cm−3 |
1.502 |
μ/mm−1 |
0.867 |
F(000) |
718.0 |
Crystal size/mm3 |
0.4 × 0.4 × 0.3 |
Radiation |
MoKα (λ = 0.71073) |
2θ range for data collection/° |
6.04 to 52.74 |
Index ranges |
−10 ≤ h ≤ 11, −14 ≤ k ≤ 14, −19 ≤ l ≤ 19 |
Reflections collected |
12 635 |
Independent reflections |
6276 [Rint = 0.0229, Rsigma = 0.0408] |
Data/restraints/parameters |
6276/0/420 |
Goodness-of-fit on F2 |
1.041 |
Final R indexes [I ≥ 2σ(I)] |
R1 = 0.0377, wR2 = 0.0887 |
Final R indexes [all data] |
R1 = 0.0503, wR2 = 0.0973 |
Largest diff. peak/hole/e Å−3 |
0.31/−0.38 |
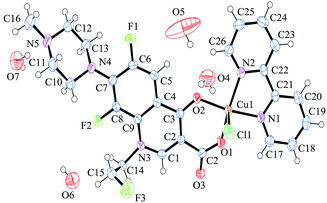 |
| Fig. 1 Molecule structure of complex 1 with atom numbering. | |
Table 2 Selected bond distance and angles for complex 1
Bond distance (Å) |
Cu(1)–O(1) |
1.908(15) |
Cu(1)–N(2) |
2.012(18) |
Cu(1)–O(2) |
1.949(15) |
O(1)–C(27) |
1.273(3) |
Cu(1)–Cl(1) |
2.572(8) |
O(2)–C(3) |
1.269(2) |
Cu(1)–N(1) |
1.999(19) |
O(3)–C(27) |
1.228(2) |
![[thin space (1/6-em)]](https://www.rsc.org/images/entities/char_2009.gif) |
Bond angle (°) |
O(1)–Cu(1)–O(2) |
93.88(6) |
O(2)–Cu(1)–N(1) |
168.43(8) |
O(1)–Cu(1)–Cl(1) |
97.77(6) |
O(2)–Cu(1)–N(2) |
93.18(7) |
O(1)–Cu(1)–N(1) |
88.58(7) |
N(1)–Cu(1)–Cl(1) |
96.19(6) |
O(1)–Cu(1)–N(2) |
158.50(8) |
N(1)–Cu(1)–N(2) |
80.71(7) |
O(2)–Cu(1)–Cl(1) |
94.67(5) |
N(2)–Cu(1)–Cl(1) |
101.85(6) |
In terms of the distances between central atom and coordinated atoms, the distances between the two oxygen atoms and central copper [Cu(1)–O(1) = 1.908 (15) Å, Cu(1)–O(2) = 1.949 (15) Å] are slightly shorter than those between nitrogen atoms and copper ion [Cu(1)–N(1) = 1.999 (19) Å, Cu(1)–N(2) = 2.012 (18) Å]. The largest distance is observed between copper and chlorine atoms [Cu(1)–Cl(1) = 2.572 (8) Å]. The whole structural feature was observed and described in previously reported similar quinolone complexes.36,37
Intermolecular hydrogen bonds are shown in Fig. S1,† and detailed information on hydrogen bonds are presented in Table S1.† The common types of existing hydrogen bonds include O–H⋯O, O–H⋯N, O–H⋯F, and O–H⋯Cl. The participating oxygen atoms all come from lattice water molecules, whereas the nitrogen and fluorine atoms come from the ligand flrx, and the chlorine atom comes from the Cu(II) salt. The crystal structure is stabilized by the hydrogen bonds to some degree. Fig. S2† shows the stacking interaction. The entire structure exhibits unique layers with an antiparallel arrangement. The distance between the adjacent flrx and bipy molecules is approximately 3.4 Å, which is often attributed to the possible existence of π–π stacking.38,39 Detailed structural information on complex 2 was provided in our previous work.26
Crystallographic data of complex 1 and 2 were submitted to the Cambridge Crystallographic Data Centre (CCDC), and these complexes were given the publication numbers CCDC-1494707 and 1457402,26 respectively.
Characterization
As for complex 1, FTIR was employed to obtain information on the coordination of ligands with metal. The presence of numerous functional groups in the molecules results in complicated spectra; thus, only the most typical vibrations are selected for interpretation.
The FTIR spectra (Fig. S3†) shows that the band at 1625 cm−1, which is attributed to pyridone stretching ν(C
O)p of flrx, slightly shifts to 1633 cm−1, revealing that flrx coordinates with Cu(II) via pyridone oxygen. The strong bands at 1720 and 1286 cm−1 assigned to ν(C
O)carboxyl and ν(C–O)carboxyl stretching vibrations of the carboxyl group (–COOH) of free flrx shift to 1589 and 1369 cm−1, respectively. These two new bands could be correspondingly ascribed to antisymmetric [νasym (CO2)] and symmetric [νsym (CO2)] stretching vibrations of the carboxylate group. Δ [=ν(CO2)asym − ν(CO2)sym] is a parameter used to determine the coordination mode of the carboxylate ligand, which is 220 cm−1 for complex 1, indicating that the carboxylate group coordinated in a monodentate mode.40 The FTIR results reveal that the deprotonated flrx ligand chelates to Cu(II) in a bidentate mode via pyridone oxygen and one carboxylate oxygen atom. The binding mode is similar to that in homogeneous complexes.10,41
Fig. S4† shows the TG result. The TG curve shows four weight loss stages. From 80 °C to 130 °C, a weight loss of 2.97% is observed, corresponding to the release of one crystal water molecule (calcd = 2.59%). The subsequent weight loss stages overlapped, causing difficulty in ascription. Taken as a whole, the subsequent weight loss of 50.13% at 130 °C to 450 °C could be generally ascribed to the loss of the three remaining crystal water molecules, the bipy, part of the piperazine ring of flrx, deprotonated carboxylgroup, and CH2CH2F (calcd = 50.38%). The final part of weight loss (7.01%) corresponds to the release of the remaining piperazine ring of flrx (calcd = 6.34%).
DSC measurement was employed to obtain information on melting point. As shown in Fig. S5,† the first endothermic peak between 120.70 °C and 160.14 °C is attributed to the release of lattice water molecules. A sharp endothermic peak appeared at 202.88 °C, corresponding to the melting point of the compound. The final exothermic peak can be attributed to the degradation of the complex. Related characterization of complex 2 was reported in our previous article.26
Given that subsequent biological studies will be performed in PBS (pH 7.40), investigating the stability of the complex in this buffer solution is necessary. Fluorescence was used to monitor whether changes have occurred. No evident change was observed (figure not shown) after monitoring for more than two days. The results revealed that the complex remained stable in PBS (pH 7.40) for a long period of time.
Interaction with CT DNA
Fluorescence studies. Fluorescence spectra can effectively provide information on the binding constants. Fig. 2 shows the fluorescence emission spectra of complex 1 (a) and 2 (b) in the presence of various concentrations of CT DNA. The fluorescence intensity of the complexes decreases regularly with increasing CT DNA concentrations, indicating that CT DNA can interact with both complexes and thus quench their intrinsic fluorescence. In addition, the shape and position of the fluorescence spectra show no detectable difference upon addition of various concentrations of CT DNA, indicating that non-covalent bonds are involved in the interactions.42
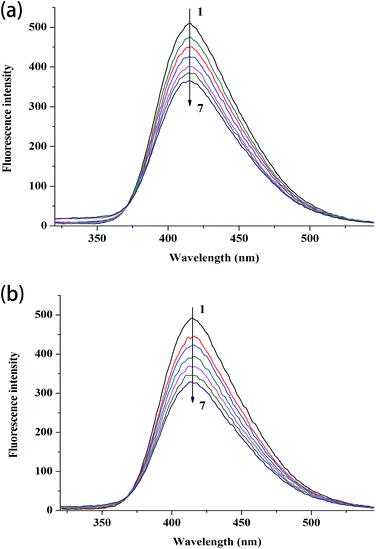 |
| Fig. 2 Fluorescence spectra of complex 1 (a) and complex 2 (b) in the absence and presence of CT DNA. Ccomplex 1 = Ccomplex 2 = 2 μM, CCT DNA (1–7): 0 μM, 2.88 μM, 5.76 μM, 8.64 μM, 11.52 μM, 14.4 μM and 17.28 μM, respectively. | |
The binding constants based on the fluorescence emission are estimated by the following equation:
|
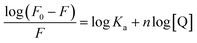 | (2) |
where
F0 and
F are the fluorescence intensities of the complexes before and after adding quencher, respectively. [Q] is the corresponding quencher concentration, and
Ka represents the binding constant. The binding constants of complex 1 and 2 are (4.98 ± 1.54) × 10
4 and (5.50 ± 1.10) × 10
4 M
−1, respectively, indicating that complex 1 and 2 exhibit high affinity to CT DNA, and complex 2 exhibits better affinity than complex 1.
Time-resolved fluorescence lifetime determination. Fluorescence studies showed that CT DNA can effectively quench the fluorescence of the two complexes. To explore the quenching mechanism, time-resolved fluorescence spectroscopy was employed as the most definitive method for distinguishing static and dynamic processing, fluorescence lifetime measurement is widely used in related studies.43 When static ground-state complexes form, the decay time of the uncomplexed fluorophores does not decrease, whereas dynamic quenching prompts part of the energy loss of the fluorophore molecules participating in the collision with quencher molecules, resulting in reduced fluorescence lifetime.44 The average florescence lifetime for biexponential iterative fitting is calculated based on the decay time and pre-exponential factors (α) using the following equation:Fig. 3 shows the fluorescence lifetime of the free complexes and the complexes after interacting with different CT DNA concentrations. As shown in Table 3, the lifetime values of free complex 1 and 2 are 0.973 and 0.958 ns, respectively. After interacting with different CT DNA concentrations, τ values for complex 1 are 1.006 and 1.028 ns and those for complex 2 are 0.999 and 1.045 ns. τ values only slightly increased with the increment in CT DNA concentration. These phenomena indicate the formation of complexes between CT DNA and the two metal complexes. Thus, the results confirm that fluorescence quenching involves a static mechanism, along with the formation of the ground-state complex.
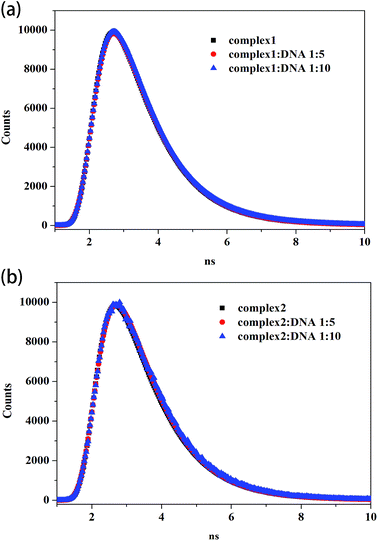 |
| Fig. 3 Time-resolved fluorescence lifetime of complex 1 (a) and complex 2 (b) (8 μM) in the absence and presence of CT DNA (40 μM, 80 μM). | |
Table 3 Fluorescence lifetime of free complexes and CT DNA-complex systems with different concentrations of CT DNA
System |
Molar ratio (CT DNA : com) |
τ1 (ns) |
τ2 (ns) |
α1 |
α2 |
τ (ns) |
χ2 |
CT DNA-complex 1 |
0 |
0.503 |
1.102 |
0.213 |
0.786 |
0.973 |
1.494 |
5 : 1 |
0.773 |
1.310 |
0.567 |
0.433 |
1.006 |
1.296 |
10 : 1 |
0.787 |
1.458 |
0.641 |
0.359 |
1.028 |
1.385 |
CT DNA-complex 2 |
0 |
0.339 |
1.053 |
0.133 |
0.867 |
0.958 |
1.051 |
5 : 1 |
0.822 |
1.357 |
0.670 |
0.330 |
0.999 |
1.252 |
10 : 1 |
0.920 |
2.175 |
0.900 |
0.100 |
1.045 |
1.288 |
ITC analysis. ITC is a well-established process that aims to investigate biological interactions fully. ITC is considered as the most effective tool to monitor the thermodynamic profiles during interactions between small molecules and macromolecules, because it can directly gain enthalpic and entropic information associated with interactions.45 Estimated binding constant (K), enthalpy of binding (ΔH), and entropy of binding (ΔS) were obtained based on the one-site mode. Gibbs energies (ΔG) can be calculated with the following equation:The ITC profiles of the binding of complex 1 and 2 with CT DNA are depicted in Fig. 4, and the related parameters are summarized in Table 4. The K value of small molecule to CT DNA is a vital reference standard to determine the magnitude of binding strength. As shown in Table 4, the K value of complex 1 to CT DNA is (1.03 ± 0.199) × 105 M−1, whereas that of complex 2 is (1.59 ± 0.238) × 105 M−1. The ITC results show that the two complexes and CT DNA systems exhibit moderately intense combinations,46 revealing a tendency that is similar to that in the fluorescence results mentioned above. Moreover, the binding constants of complex 1 and 2 to CT DNA are of the same magnitude compared to other quinolone–Cu mixed ligand complexes.46,47 The K values of complex 1 and 2 are of the same order of magnitude as that of the classical intercalator EB (K = (1.23 ± 0.07) × 105 M−1).14 Although the two K values are in the same order of magnitude, the ternary complex containing phen (complex 2) exhibits better affinity to CT DNA than the ternary complex containing bipy (complex 1), which is consistent with previous results.37,44 This disparity can be explained by the fact that a relatively better planar is present in phen, and flat molecules can slide into the base pairs of DNA (intercalate).48 For the binding of complex 1 and 2 to CT DNA, ΔH is 41.2 ± 5.51 and 38.7 ± 2.83 kJ mol−1, respectively; ΔS is 233 and 229 J mol−1 K−1, respectively; and the calculated ΔG is −28.3 ± 5.51 and −29.6 ± 2.83 kJ mol−1, respectively. The ΔH and ΔS values reveal that the interactions of the complexes with CT DNA are driven by an unfavorable increase in enthalpy, along with considerable enhancements in beneficial entropy.49 The main interaction force occurring between CT DNA and small molecules can be deduced via thermodynamic parameters. ΔH < 0 or ΔH ≈ 0 along with ΔS > 0 corresponds to electrostatic force; ΔH < 0 and ΔS < 0 refer to van der Waals interactions or hydrophobic interactions; and ΔH > 0 as well as ΔS > 0 indicates hydrophobic force.50 The positive values of ΔH and ΔS illustrate that the main force for both interactions is hydrophobic force, which is speculated to existed in intercalating agent–DNA systems.48 Moreover, the negative values of ΔG indicates that the two interactions are spontaneous processes. Apart from the binding affinities, the thermodynamic parameters of the two complexes to CT DNA also show no considerable differences, which can be explained by the similar crystal structures. Furthermore, the K, ΔH, and ΔS values indicate that the most possibility binding mode between the two complexes and CT DNA is intercalation.
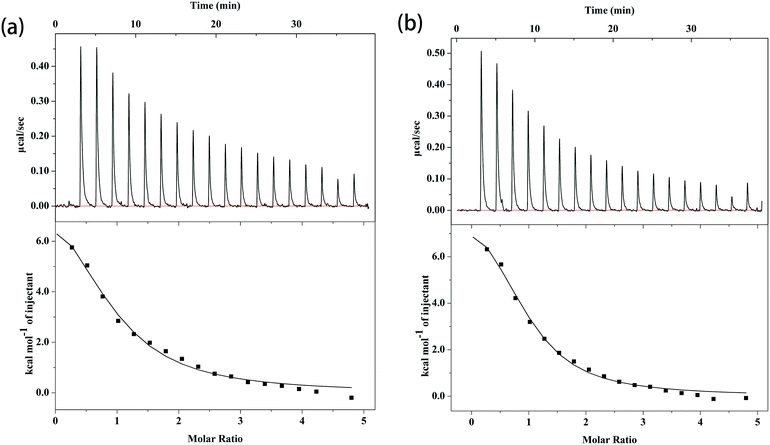 |
| Fig. 4 The isothermal calorimetry titration curves of complex 1 (a) and complex 2 (b) with CT DNA. | |
Table 4 ITC-derived parameters for the binding of complex 1 and complex 2 to CT DNA
Complex |
Complex 1 |
Complex 2 |
K (M−1) |
(1.03 ± 0.199) × 105 |
(1.59 ± 0.238) × 105 |
ΔH (kJ mol−1) |
41.2 ± 5.51 |
38.7 ± 2.83 |
ΔS (J mol−1 K−1) |
233 |
229 |
ΔG (kJ mol−1) |
−28.3 ± 5.51 |
−29.6 ± 2.83 |
Iodide quenching studies. KI, an effective fluorescence quencher to small molecules, can be used to determine the interaction modes between small molecules and CT DNA by monitoring the variation of fluorescence in the presence and absence of CT DNA.57 When small molecules intercalate into DNA base pairs, the fluorescence quenching effect of KI in the DNA–small molecule system would be lower than that in the free small molecule because of the electrostatic repulsion interaction between DNA phosphate backbone and iodide anions. By contrast, DNA provides less protection to small molecules during groove binding, resulting in easy quenching of the groove binders compared with that during intercalative binding.58 Fig. 6a and b shows the quenching efficiency of KI in the CT DNA – complex system. This finding indicated that the two complexes can intercalate into CT DNA and thus are protected by the double helix of CT DNA.
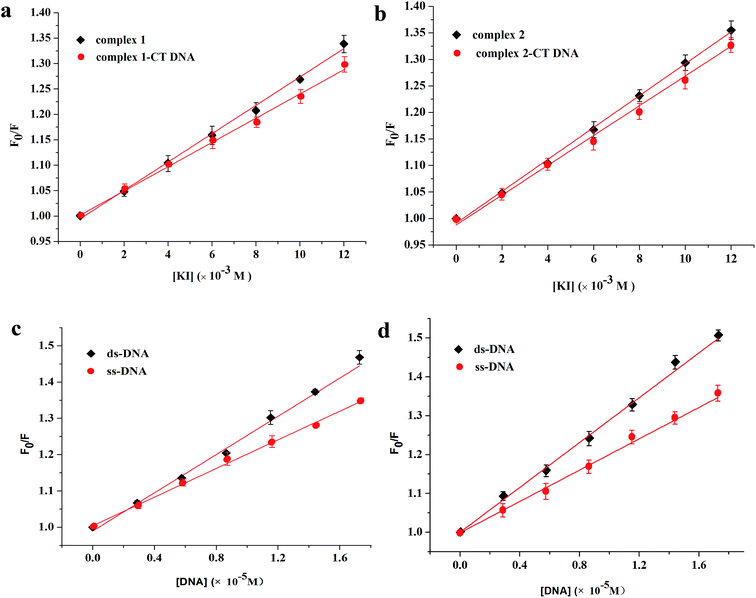 |
| Fig. 6 Quenching effects of KI to complex 1 (a) and complex 2 (b) in the presence and absence of CT DNA and fluorescence quenching results of complex 1 (c) and complex 2 (d) by ds-DNA and ss-DNA. | |
Interaction between ss-CT DNA and ds-CT DNA. The diverse effects of ss-DNA and ds-DNA on the intrinsic fluorescence of small molecules correspond to different binding modes. When small molecules intercalate into the base pairs of CT DNA, the quenching effect of ss-DNA should be weaker than that of ds-DNA because ss-DNA exhibits released strands. If the binding mode is groove binding, the fluorescence quenching effect on small molecules of ss-DNA should be greater than that of ds-DNA because ss-DNA provides a relatively larger groove space. The electrostatic effect contributes to the interaction between small molecules and DNA; the quenching effect of ss-DNA and ds-DNA on small molecules should be the same.59 In order to determine the binding modes between CT DNA and the two complexes, the fluorescence intensity changes after adding various concentration of ss-DNA and ds-DNA were investigated. The quenching constant can be deduced by the Stern–Volmer equation, as follows:42where F0 and F represent the fluorescence intensities of the complexes before and after adding various concentrations of quencher, respectively. [Q] denotes the quencher concentration and Ksv is the Stern–Volmer quenching constant.Fig. 6c and d shows the quenching results after the reaction. For complex 1, the calculated quenching constants are (2.63 ± 0.06) × 104 and (1.97 ± 0.04) × 104 M−1 for ds-DNA and ss-DNA, respectively. For complex 2, the slopes are (2.92 ± 0.04) × 104 and (2.09 ± 0.06) × 104 M−1 for ds-DNA and ss-DNA, respectively. The quenching ability of ds-DNA is evidently stronger than that of ss-DNA, indicating that the two complexes could intercalate into CT DNA. The possible binding modes identified in this article are consistent with those shown in the previous experiments.
Conclusion
This study characterized the complex of Cu(II) with the quinolone antibacterial drug flrx which was synthesized in the presence of bipy. X-ray crystallography and other complementary methods revealed that flrx coordinated with Cu(II) via pyridone oxygen and one carboxylate oxygen atom. Moreover, the complex exhibited the space group P
in triclinic form.
The interactions of the synthesized and previous reported complexes with CT DNA were investigated using ITC and various spectroscopic techniques. Fluorescence investigation showed that CT DNA quenched the fluorescence of the two complexes effectively. The quenching mechanisms involved static model, as shown by time-resolved fluorescence lifetime determination. The binding constants determined by ITC showed that both complexes exhibited moderate binding ability, although complex 2 exhibited better affinity to CT DNA than complex 1, which is consistent with the fluorescence results. The thermodynamic profiles, including ΔG, ΔS, and ΔH, indicated that binding occurred spontaneously, along with considerable enhancement in beneficial entropy and unfavorable increase in enthalpy. The binding mode of the two complexes to CT DNA is most likely intercalative binding based on the results of CD explorations, KI quenching studies, and DNA denaturation experiments.
Acknowledgements
This work was supported by the National Development and Reform Commission and Education of China (Grant No. 2014BW011) and the Large-scale Science Instrument Shareable Platform Construction of Sichuan Province (Grant No. 2015JCPT0005-15010102).
References
- J. Tuma, W. H. Connors, D. H. Stitelman and C. Richert, J. Am. Chem. Soc., 2002, 124, 4236–4246 CrossRef CAS PubMed.
- I. Tsitsa, A. Tarushi, P. Doukoume, F. Perdih, A. de Almeida, A. Papadopoulos, S. Kalogiannis, A. Casini, I. Turel and G. Psomas, RSC Adv., 2016, 6, 19555–19570 RSC.
- L. A. Mitscher, Chem. Rev., 2005, 105, 559–592 CrossRef CAS PubMed.
- P. Mukherjee, E. R. Mandal and S. K. Das, Int. J. Hum. Genet., 2005, 5, 57 CAS.
- O. Aranha, R. Grignon, N. Fernandes, T. J. McDonnell, D. P. Wood and F. H. Sarkar, Int. J. Oncol., 2003, 22, 787–794 CAS.
- G. G. Mohamed, H. F. Abd El-Halim, M. M. I. El-Dessouky and W. H. Mahmoud, J. Mol. Struct., 2011, 999, 29–38 CrossRef CAS.
- W. H. Mahmoud, G. G. Mohamed and M. M. I. El-Dessouky, J. Mol. Struct., 2015, 1082, 12–22 CrossRef CAS.
- N. E. A. El-Gamel and M. A. Zayed, Spectrochim. Acta, Part A, 2011, 82, 414–423 CrossRef CAS PubMed.
- M. A. Ragheb, M. A. Eldesouki and M. S. Mohamed, Spectrochim. Acta, Part A, 2015, 138, 585–595 CrossRef CAS PubMed.
- M. Zampakou, S. Balala, F. Perdih, S. Kalogiannis, I. Turel and G. Psomas, RSC Adv., 2015, 5, 11861–11872 RSC.
- G. Psomas and D. P. Kessissoglou, Dalton Trans., 2013, 42, 6252 RSC.
- M. E. Katsarou, E. K. Efthimiadou, G. Psomas, A. Karaliota and D. Vourloumis, J. Med. Chem., 2008, 51, 470–478 CrossRef CAS PubMed.
- R. Singh, R. N. Jadeja, M. C. Thounaojam, T. Patel, R. V. Devkar and D. Chakraborty, Inorg. Chem. Commun., 2012, 23, 78–84 CrossRef CAS.
- A. Tarushi, C. P. Raptopoulou, V. Psycharis, A. Terzis, G. Psomas and D. P. Kessissoglou, Bioorg. Med. Chem., 2010, 18, 2678–2685 CrossRef CAS PubMed.
- K. C. Skyrianou, F. Perdih, I. Turel, D. P. Kessissoglou and G. Psomas, J. Inorg. Biochem., 2010, 104, 161–170 CrossRef CAS PubMed.
- R. Uauy, M. Olivares and M. Gonzalez, Am. J. Clin. Nutr., 1998, 67, 952S–959S CAS.
- R. B. Rucker, T. Kosonen, M. S. Clegg, A. E. Mitchell, B. R. Rucker, J. Y. Uriu-Hare and C. L. Keen, Am. J. Clin. Nutr., 1998, 67, 996S–1002S CAS.
- S. Lian, G. Wang, L. Zhou and D. Yang, Luminescence, 2013, 28, 967–972 CrossRef CAS PubMed.
- W. Yan, Z. Tieli, Z. Huichun and J. Linpei, Anal. Lett., 2000, 33, 3303–3314 CrossRef CAS.
- S. Q. Shah and M. R. Khan, Transition Met. Chem., 2011, 36, 283–287 CrossRef CAS.
- A. Subastri, C. H. Ramamurthy, A. Suyavaran, R. Mareeswaran, P. Lokeswara Rao, M. Harikrishna, M. Suresh Kumar, V. Sujatha and C. Thirunavukkarasu, Int. J. Biol. Macromol., 2015, 78, 122–129 CrossRef CAS PubMed.
- L. H. Hurley, Nat. Rev. Cancer, 2002, 2, 188–200 CrossRef CAS PubMed.
- S. U. Rehman, Z. Yaseen, M. A. Husain, T. Sarwar, H. M. Ishqi and M. Tabish, PLoS One, 2014, 9, e93913 Search PubMed.
- M. N. Patel, P. A. Dosi and B. S. Bhatt, Med. Chem. Res., 2011, 21, 2723–2733 CrossRef.
- K. Shiva Prasada, L. Shiva Kumara, B. Vijaya and H. D. Revanasiddappa, MyScience, 2010, 5, 1–10 Search PubMed.
- Y. Xiao, Q. Wang, Y. Huang, X. Ma, X. Xiong and H. Li, Dalton Trans., 2016, 45, 10928–10935 RSC.
- C.-Y. Chen, Q.-Z. Chen, X.-F. Wang, M.-S. Liu and Y.-F. Chen, Transition Met. Chem., 2009, 34, 757–763 CrossRef CAS.
- J. Marmur, J. Mol. Biol., 1961, 3, 208–218 CrossRef CAS.
- O. V. Dolomanov, L. J. Bourhis, R. J. Gildea, J. A. K. Howard and H. Puschmann, J. Appl. Crystallogr., 2009, 42, 339–341 CrossRef CAS.
- G. M. Sheldrick, Acta Crystallogr., Sect. C: Struct. Chem., 2015, 71, 3–8 CrossRef PubMed.
- M. Shahlaei, B. Rahimi, M. R. Ashrafi-Kooshk, K. Sadrjavadi and R. Khodarahmi, J. Lumin., 2015, 158, 91–98 CrossRef CAS.
- F. Y. Wu, Y. L. Xiang, Y. M. Wu and F. Y. Xie, J. Lumin., 2009, 129, 1286–1291 CrossRef CAS.
- A. W. Addison, T. N. Rao, J. Reedijk, J. van Rijn and G. C. Verschoor, J. Chem. Soc., Dalton Trans., 1984, 7, 1349–1356 RSC.
- E. Yareth Bivián-Castro, F. Cervantes-Lee and G. Mendoza-Díaz, Inorg. Chim. Acta, 2004, 357, 349–353 CrossRef.
- E. Chalkidou, F. Perdih, I. Turel, D. P. Kessissoglou and G. Psomas, J. Inorg. Biochem., 2012, 113, 55–65 CrossRef CAS PubMed.
- D. K. Saha, S. Padhye, C. E. Anson and A. K. Powell, Transition Met. Chem., 2003, 28, 579–584 CrossRef CAS.
- P. Ruiz, R. Ortiz, L. Perello, G. Alzuet, M. Gonzalez-Alvarez, M. Liu-Gonzalez and F. Sanz-Ruiz, J. Inorg. Biochem., 2007, 101, 831–840 CrossRef CAS PubMed.
- P. Fernandes, I. Sousa, L. Cunha-Silva, M. Ferreira, B. de Castro, E. F. Pereira, M. J. Feio and P. Gameiro, J. Inorg. Biochem., 2014, 131, 21–29 CrossRef CAS PubMed.
- V. A. Kawade, A. A. Kumbhar, A. S. Kumbhar, C. Nather, A. Erxleben, U. B. Sonawane and R. R. Joshi, Dalton Trans., 2011, 40, 639–650 RSC.
- E. P. Irgi, G. D. Geromichalos, S. Balala, J. Kljun, S. Kalogiannis, I. Turel and G. Psomas, RSC Adv., 2015, 5, 36353–36367 RSC.
- C. Protogeraki, E. G. Andreadou, F. Perdih, I. Turel, A. A. Pantazaki and G. Psomas, Eur. J. Med. Chem., 2014, 86, 189–201 CrossRef CAS PubMed.
- J. Chai, J. Wang, Q. Xu, F. Hao and R. Liu, Mol. BioSyst., 2012, 8, 1902–1907 RSC.
- K. Shanmugaraj, S. Anandakumar and M. Ilanchelian, Dyes Pigm., 2015, 112, 210–219 CrossRef CAS.
- D. Xiao, L. Zhang, Q. Wang, X. Lin, J. Sun and H. Li, J. Lumin., 2014, 146, 218–225 CrossRef CAS.
- S. K. Jana, S. K. Seth, H. Puschmann, M. Hossai and S. Dala, RSC Adv., 2014, 4, 57855–57868 RSC.
- M. N. Patel, C. R. Patel and H. N. Joshi, Appl. Biochem. Biotechnol., 2013, 169, 1329–1345 CrossRef CAS PubMed.
- F. Dimiza, S. Fountoulaki, A. N. Papadopoulos, C. A. Kontogiorgis, V. Tangoulis, C. P. Raptopoulou, V. Psycharis, A. Terzis, D. P. Kessissoglou and G. Psomas, Dalton Trans., 2011, 40, 8555–8568 RSC.
- M. Hasanzadeh and N. Shadjou, Mater. Sci. Eng., C, 2016, 61, 1002–1017 CrossRef CAS PubMed.
- G. Wang, H. Wu, D. Wang, C. Yan and Y. Lu, Spectrochim. Acta, Part A, 2013, 104, 492–496 CrossRef CAS PubMed.
- S. Kashanian, M. M. Khodaei, H. Roshanfekr, N. Shahabadi and G. Mansouri, Spectrochim. Acta, Part A, 2012, 86, 351–359 CrossRef CAS PubMed.
- P. Zhao, S.-F. Jin, J.-Z. Lu, J.-L. Lv, G.-Q. Wu, P.-P. Chen, C.-L. Tan and D.-W. Chen, Spectrochim. Acta, Part A, 2015, 137, 227–235 CrossRef CAS PubMed.
- K. Nejedly, J. Chladkova, M. Vorlickov, I. Hrabcova and J. Kypr, Nucleic Acids Res., 2005, 33, e5 CrossRef PubMed.
- J. Kypr and M. Vorlickova, Biopolymers, 2002, 67, 275–277 CrossRef PubMed.
- N. Shahabadi, Z. Mirzaei kalar and N. H. Moghadam, Spectrochim. Acta, Part A, 2012, 96, 723–728 CrossRef CAS PubMed.
- P. Uma Maheswari and M. Palaniandavar, J. Inorg. Biochem., 2004, 98, 219–230 CrossRef CAS PubMed.
- U. Saha and K. K. Mukherjea, Int. J. Biol. Macromol., 2014, 66, 166–171 CrossRef CAS PubMed.
- G. Zhang, X. Hu and P. Fu, J. Photochem. Photobiol., B, 2012, 108, 53–61 CrossRef CAS PubMed.
- B. Qiu, L. Guo, W. Wang and G. Chen, Biosens. Bioelectron., 2007, 22, 2629–2635 CrossRef CAS PubMed.
- C. Cai, X. Chen and F. Ge, Spectrochim. Acta, Part A, 2010, 76, 202–206 CrossRef PubMed.
Footnote |
† Electronic supplementary information (ESI) available. CCDC 1494707. For ESI and crystallographic data in CIF or other electronic format see DOI: 10.1039/c6ra18971g |
|
This journal is © The Royal Society of Chemistry 2016 |